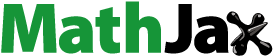
Abstract
Burn wounds are associated with infections, drug resistance, allergic reactions, odour, bleeding, excess exudates, and scars, requiring prolonged hospital stay. It is crucial to develop wound dressings that can effectively combat allergic reactions and drug resistance, inhibit infections, and absorb excess exudates to accelerate wound healing. To overcome the above-mentioned problems associated with burn wounds, SA/PVA/PLGA/Capparis sepiaria and SA/PVA/Capparis sepiaria nanofibers incorporated with Capparis sepiaria plant extract were prepared using an electrospinning technique. Fourier-transform infrared spectroscopy confirmed the successful incorporation of the extract into the nanofibers without any interaction between the extract and the polymers. The nanofibers displayed porous morphology and a rough surface suitable for cellular adhesion and proliferation. SA/PVA/PLGA/Capparis sepiaria and SA/PVA/Capparis sepiaria nanofibers demonstrated significant antibacterial effects against wound infection-associated bacterial strains: Pseudomonas aeruginosa, Enterococcus faecalis, Mycobaterium smegmatis, Escherichia coli, Enterobacter cloacae, Proteus vulgaris, and Staphylococcus aureus. Cytocompatibility studies using HaCaT cells revealed the non-toxicity of the nanofibers. SA/PVA/PLGA/Capparis sepiaria and SA/PVA/Capparis sepiaria nanofibers exhibited hemostatic properties, resulting from the synergistic effect of the plant extract and polymers. The in vitro scratch wound healing assay showed that the SA/PVA/Capparis sepiaria nanofiber wound-healing capability is more than the plant extract and a commercially available wound dressing. The wound-healing potential of SA/PVA/Capparis sepiaria nanofiber is attributed to the synergistic effect of the phytochemicals present in the extract, their porosity, and the ECM-mimicking structure of the nanofibers. The findings suggest that the electrospun nanofibers loaded with Capparis sepiaria extract are promising wound dressings that should be explored for burn wounds.
1. Introduction
Burn injuries are challenging to treat due to infections. There is a pressing need to explore new and effective wound dressings for burn wounds. Human skin is the first defence barrier, the body’s largest organ, and is crucial in controlling body temperature [Citation1,Citation2]. The skin is characterized by protective immune cells, the epidermis and dermis layers that are involved in the repair of tissue and the inhibition of infections [Citation3]. The wound healing process involves a combination of a series of biological events [Citation4], such as cell growth and differentiation, leading to accelerated healing [Citation5]. Some wound types display prolonged periods of healing [Citation6]. Non-healing wounds are still problematic and associated with prolonged treatment, despite the available wound dressings. Some of the non-healing wounds include burn wounds, chronic wounds, surgical wounds, and infected wounds [Citation7,Citation8]. Selecting appropriate wound dressing is crucial for accelerated wound healing. Using an unsuitable wound dressing can delay the healing process, causing a financial burden on the patient, and increasing morbidity [Citation9].
The available wound dressing suffers from shortcomings, including insufficient exudate absorption, poor mechanical and antibacterial properties, pain/discomfort upon removal, non-flexibility, unable to maintain a moist microenvironment, and can induce allergic reactions [Citation10–12]. To overcome these limitations, nanofibers have been widely developed using the electrospinning method, where synthetic and biopolymers are combined to form hybrid nanofibers wound dressing with enhanced properties [Citation13]. Sodium alginate-based nanofibers wound dressing encapsulated with plant-based bioactive agents displayed improved healing properties [Citation14–16]. An ideal wound dressing should be easily taken off without causing skin discomfort, biodegradable, biocompatible, accelerate wound healing, provide moisture to the wound bed, be cytocompatible, prevent microbial invasion, absorb exudate, and support cell proliferation, non-toxic, and promote skin regeneration [Citation17].
Nanofibers display some features of ideal wound dressings and they are widely prepared using the electrospinning method. They can be used for various applications, including tissue engineering, biosensors, drug delivery, adsorption, energy storage, food packaging, wound dressing, catalysis, electronic devices, facial masks, implants, etc. [Citation18]. The electrospinning method is cost-effective, simple to use, highly efficient and scalable, the diameters are controllable, and the excellent properties of nanofibers, include aspect ratio, uniform porosity, tunable wettability, high surface area, fine flexibility, and easy tunability [Citation19,Citation20]. The advantages of electrospun nanofibers that make them useful for wound dressings are their ability to act as the extracellular matrix (ECM), good haemostasis effect, and capability to prevent scarring and absorb large amounts of wound exudates [Citation21,Citation22]. Nanofibers-based wound dressings have been reported to display large water absorption relative to their mass, be biocompatible, and exhibit good oxygen permeability, which contributes to wound healing with reduced scarring [Citation23,Citation24].
In the preparation of nanofibers via electrospinning method, synthetic polymers that have been used in enhancing the spinnability of sodium alginate include, poly(lactic-co-glucolic) acid (PLGA), Polyvinyl alcohol (PVA), nylon, polyurethane, polyacrylonitrile, polyester, cellulose acetate, polycaprolactone, poly (lactic acid), etc. [Citation25]. PVA display properties including non-toxicity, biocompatibility, hydrophilicity, biodegradability, adhesion, and high mechanical properties [Citation26]. However, PVA’s non-bioactivity and poor exudate absorption capacity make it unsuitable to be used alone for the development of wound dressings [Citation27,Citation28]. PLGA, a copolymer of polyglycolic and polylactic acid, is a biodegradable polymer that is widely explored in the development of wound dressing to treat burn wounds [Citation29,Citation30]. Biopolymers have been widely explored for the development of wound dressing [Citation31]. Sodium alginate is a biopolymer characterized by outstanding features including high absorption capacity, non-toxicity, non-immunogenicity, biocompatibility, and affordability [Citation23,Citation32,Citation33]. Sodium alginate properties have been explored for the design of non-adherent and porous wound dressings [Citation34] to accelerate the wound healing process [Citation35,Citation36]. Apart from using biopolymers or synthetic polymers to design wound dressings, the loading of plant-based extracts into polymer-based wound dressings is a promising approach to be explored to enhance the biological activity of the wound dressings.
Plant-based extracts have been explored by few researchers in wound healing due to their limited allergic reaction and drug resistance, when compared to antibiotics, making them suitable bioactive agents for treating infected and burn wounds [Citation37]. Capparis Sepiaria, a medicinal plant from the genus Capparis, is well known for its antimicrobial, anti-inflammatory, wound healing, antifungal, antioxidant, and haemostatic properties, making them useful for treating burn wounds [Citation38,Citation39]. However, they have not been widely explored in wound dressings. There are only two reports on wound dressings loaded with other species of Capparis [Citation40,Citation41] and there is no report on wound dressings loaded with Capparis Sepiaria. The novelty of this research lies in the exploration of the aqueous root extract of Capparis Sepiaria which was loaded into the nanofibers to take advantage of its biological properties (i.e. antimicrobial, haemostatic, and wound-healing properties) together with the outstanding features of the polymers (i.e. SA, PLGA, and PVA)
In this study, SA/PVA/PLGA and SA/PVA nanofibers were electrospun and encapsulated with Capparis Sepiaria for wound healing. The aqueous root extract of Capparis Sepiaria was evaluated using Ultra-Performance Liquid Chromatography (UPLC) to identify the phytochemical constituents of the extract. The nanofibers were evaluated using FTIR and SEM, followed by in vitro biological evaluations (i.e. haemostasis, antibacterial, cytotoxicity, and wound healing potential employing scratch assay).
2. Materials and methods
2.1. Materials
Polyvinyl alcohol (PVA, Molecular weight (MW): 89 000-98 000), Sodium alginate (SA), Poly-(D, L-lactide)-co-glycolide (PLGA, MW: 7000-17000), N, N’–dimethylformamide (DMF), and chloroform were all purchased from Sigma Aldrich. Nanofibers were prepared using distilled water. The syringe pump employed for electrospinning came with adjustable speed, purchased from African Inclusive Microscopy (IFINMIC). The plant extract was supplied by Global Health Biotech (Pty) Ltd, South Africa.
2.2. Preparation of SA-based nanofibers
The electrospinning method addresses the key advantages of nanofibers, such as high surface area, tunable morphologies, and porosity which are influenced by polymer solution and processing settings [Citation42]. These features contribute to the nanofibers’ capability to mimic the natural extracellular matrix (ECM), providing a suitable environment that facilitates cell adhesion, growth, and proliferation, which are essential for wound healing [Citation43]. The spinning solutions which were prepared include sodium alginate (SA, 2% w/v), Polyvinyl alcohol (PVA, 10% w/v), Polyvinyl (PVA, 16% w/v), Polyvinyl (PVA, 8% w/v), and Poly-(D, L,-O-lactide)-co-glycoside (PLGA, 10% w/v). SA and PVA solutions were prepared by dissolving them in distilled water at room temperature and 80° C, respectively. PLGA solution was prepared by dissolving it in chloroform/DMF (8:2% w/v) ratio. The synthetic polymers, PVA and PLGA enhanced the viscosity of SA, therefore enabling electrospinning. The SA (2% w/v), PVA (10% w/v), and PLGA (10% w/v) spinning solutions were combined and loaded with the Capparis sepiaria extract. Then, SA (2% w/v)/PVA (16% w/v), and SA (2% w/v)/PVA (8% w/v) spinning solutions were also combined and loaded with Capparis sepiaria plant extract, respectively. The spinning solutions were prepared under an ambient temperature of 25 °C ().
Table 1. The composition of the nanofibers (NF7, NF10, and NF13).
The mixed spinning solutions were then electrospun, by placing them in a 20 mL syringe connected to a pipe that was connected to a needle tip with a 0.31 mm inner diameter spinate. The distance set for nanofiber collection was 15 cm from the tip of the needle to a collector, the collector used was an aluminium foil. The voltage applied between the needle tip and the aluminium foil during electrospinning was set to 15 kV, with a flow rate of 10 µL/min. To confirm the formation of nanofibers, SEM was employed.
2.3. Characterizations
2.3.1. FTIR
The FTIR analysis was carried out on the plant extract, and the nanofibers, NF7, NF10, and NF13 to evaluate the presence of characteristic functional groups and confirm the successful encapsulation of the plant extract into the nanofibers. A Perkin Elmer Spectrum 100 spectrometer (USA) was used in a wavenumber ranging from 4000 to 500 cm−1 and then plotted using Origin software.
2.3.2. SEM
The morphology of the NF7, NF10, and NF13 nanofibers, was evaluated using the SEM analysis. The SEM analysis was performed by taking a small amount of the nanofiber and placing it on aluminium stubs, coating them with gold before the analysis. SEM was performed at an accelerated voltage of 15 kV, using JSM-6390LV, purchased from Tokyo.
2.3.3. The in vitro antibacterial studies
The MICs of the nanofibers, including NF7, NF10, and NF13, were determined according to the procedure outlined in the study by Fonkui et al. [Citation44]. To prepare the stock solutions, 5 mg of each nanofiber was placed in individual tubes, containing 3 mL of DMSO. The resulting solutions were diluted with 100 µL of nutrient broth to reach the desired concentration in 96-well plates of 1.666, 0.833, 0.416, 0.208, 0.104, 0.052, and 0.026 mg/mL. Each solution was replicated and seeded overnight with 100 μL of bacterial culture that was brought to Farland of 0.5 Mc in nutrient broth. Streptomycin, nalidixic acid, and plant extract were employed as positive controls, while the negative control contained a nutrient broth of 50% in DMSO.
2.3.4. AFM
AFM was used to evaluate the surface roughness of the nanofibres. AFM of the nanofibers was performed under ambient conditions using a silicone ultrasharp cantilever Bruker under a non-contact mode. The AFM instrument tip had a radius of curvature. AFM spectra were captured at 50% light intensity, 45% light rotation, and light pitch. The AFM technique was employed to assess the Ra and Rq values, which pertain to the average surface roughness and root mean square roughness, respectively.
2.3.5. Water vapour transmission rate (WVTR)
The WVTR of the NF7, NF10, and NF13 nanofibers were evaluated following the American Society for Testing and Materials (ASTM) method [Citation45,Citation46]. A 15 ml plastic sample vial was employed with a diameter of 0.8 cm, and the nanofibers were placed over the circular opening of the sample vial with a radius of 1.6 cm. It was placed in a sample containing 5 mL of distilled water and sealed using parafilm. The entire setup was weighed and the initial mass was recorded. The whole setup for the nanofibers and control was placed in a warm bath shaker at 37 °C for 24 h. After 24 h, the setup was weighed again and the WVTR was evaluated using the following equation:
(1)
(1)
where Wi is the weight of the whole setup before placing it in a warm bath shaker, and WA after 24 h from the shaker. A represents the area of the sample from the mouth (πd2, where d is the diameter).
2.3.6. In vitro cell viability studies
The objective of evaluating the in vitro cytotoxicity of the nanofibers was to assess their biocompatibility using MTT assay. The nanofibers were tested against immortalized human keratinocyte cells (HaCaT), which were cultured at a density of 5x104 cells/mL at a volume of 90 μL/well in a 96-well plate. After 24 h, each sample was treated with 10 μL of the nanofiber solution, leading to final concentrations of 3.125, 12.5, 25, 50, 100, and 200 μg/mL. The positive and negative controls were cells that were treated with 10% DMSO and 1xPBS, respectively. MTT reagent was added to the 96-well plate and incubated for 48 h. The plates were further incubated for 4 h. The values of absorbance were obtained at 570 nm (wavelength) after conducting an overnight solubilization of the cells using a solubilization reagent [Citation47]. The analysis was conducted in triplicates, and the cell viability percentage of each nanofiber was compared to the untreated cells based on the equation below:
(1)
(1)
AN refers to the absorbance for the prepared nanofiber, while Ab represents the blank absorbance. On the other hand, the untreated sample absorbance is signified by Au.
2.3.7. The In vitro scratch wound healing assay
The in vitro scratch wound-healing study was performed on NF10 nanofiber, employing a known and reported procedure [Citation48–50]. The study utilized immortalized human keratinocyte (HaCaT) cells, which were grown in a humidified incubator that maintained a temperature of 37 °C and a CO2 level of 5% to reach 90% confluence in DMEM with 10% fetal bovine serum (FBS) and 1% antibiotic (Penstrep). Trypsinized cells, and then determined the number of viable cells by employing trypan blue dye exclusion procedure. The cell density was set to 2.5 x 105 cells/mL and seeded in 6-well plates and after 48 h a cell monolayer was formed. Scratch wounds were inflicted on each well by employing a 200 µL tip of a micropipette. To remove any cells that may have become dislodged, the wells were washed once with 2 mL of 1X phosphate-buffered saline (1X PBS), followed by the addition of serum-poor DMEM medium (containing 1% FBS) to the wells, where 1800 µL was added to each well. Furthermore, the cells were treated with 200 µL of NF10 at numerous concentrations which displayed significantly enhanced cell viability on the screen of MTT assay.
The positive control was represented by the untreated cells cultured in DMEM with 10% of FBS, while the negative control was those in DMEM with 1% of FBS. The scratch images were captured using a 4X objective lens and light microscope which is inverted with phase contrast feature (Olympus CKX53, Olympus, Tokyo, Japan) at 0, 24, 48, 72, and 96 h. Images were captured in triplicates, the cell migration was measured using ImageJ software, and wound closure (WC) was computed using the equation below:
(2)
(2)
2.3.8. In vitro haemostasis
The whole blood clot analysis was carried out according to a known method at a wavelength of 540 nm [Citation51,Citation52]. To assess statistical significance, a student’s t-test was employed to compare the standard deviation and average of tested samples to that of the negative control. About 2 mg of nanofiber was immersed in 200 µL of whole blood, followed by blood coagulation activation using CaCl2 (20 µL). The mixture was then incubated in a thermostatic incubator for 10 min with considerate shaking at 37 °C. Subsequently, about 6 mL of deionized water was then added to haemolyse the RBCs (cells of the red blood) by dripping. The samples of blood were diluted to a volume of 25 mL to determine their relative absorbance.
2.3.9. Statistical
The nanofiber’s biological studies were all performed in triplicates. The obtained data was displayed as mean ± SD. Also, to compare group differences, ANOVA was employed using the software known as SPSS. The spectrums were plotted using a software named Origin. Data is considered statistically significant when p < 0.05.
2.4. Plant extract
The plant extract was supplied by Global Health Biotech (Pty) Ltd, South Africa. It was characterized by FTIR, Ultraviolet-visible Spectroscopy (UV-vis), and Ultra-Performance Liquid Chromatography-Mass Spectrometry (UPLC-MS). The UPLC-MS chromatograms are shown in Supplementary Figures.
3. Results
The UPLC-MS chromatogram of the Capparis Sepiaria plant extract revealed signals at 195.0500, 191.0208, 289.0389, and 435.1317 for gluconic acid, citric acid, epicatechin, and phloretin-2-o-glucoside, respectively (Supplementary Figure). The structures are shown in .
3.1. FTIR
The FTIR spectrum of Capparis sepiaria showed peaks at 3300-2900 cm−1 for O-H stretch, C-H at 2918 cm−1, =C-H for aromatic stretching at 1598-1428 cm−1, C-N and C-O stretching at 1236 cm−1, C-O stretching at 1055 cm−1, C-H bending at 884 cm−1 for 1,2,4-trisubstituted, and at 783 cm−1 for C = C bending (, ). The nanofibers, NF7, NF10, and NF13 displayed similar peaks at 3303, 2949-2904, 1753, 1603-1420, 1140, 1090, 919, and 841 cm−1 for O-H, C-H, C = O, =C-H, C-N, C-O stretching, C-H bending, and C = C bending, respectively (). The C-O and the O-H stretching are attributed to the polymerization between the SA, PLGA or PVA. However, the intensity of the C-O stretch for N7 was significant when compared to N10 and N13, resulting from the absence of PLGA in N10 and N13. The peaks at 1753 cm−1 are due to the acetate C = O and O-H band was visible at 3303 cm−1.
Table 2. Characteristic peaks present on the nanofibers FTIR spectra of NF7, NF10, NF13, and capparis sepieria extract.
3.2. SEM
The obtained SEM morphologies determined the nanofiber’s porous nature and surface morphology of (NF7, NF10, and NF13) shown in . The SEM images of the NFs displayed smooth fibre surfaces. The NF10 and NF13 displayed beadless fibres when compared to NF7 with few and insignificant beaded fibres. The average diameter of the fibres NF7, NF10, and NF13 was 1.147 ± 2.730, 1.344 ± 2.288, and 1.852 ± 3.013 µm, respectively which was measured using ImageJ software [Citation53].
3.3. AFM
The surface roughness of the prepared NF7 and NF13 nanofibers is shown in and . The surface average roughness of NF7 and NF13 nanofibers were 214.33 ± 138.4, and 355 ± 242.935 nm, respectively. The degree of surface roughness of NF13 was significant when compared to NF7.
Table 3. Surface roughness parameters obtained from AFM analysis.
3.4. WVTR
WVTR was performed on the nanofibers NF7, NF10, and NF13 to evaluate if they can maintain a moisture microenvironment that is essential to promote cell granulation and epithelization. High WVTR leads to rapid wound dehydration, while low WVTR contributes to the accumulation of wound exudates, which delay the healing process and pose a huge risk of infection. The NF7, NF10, and NF13 nanofibers displayed WVTR values in the range of 193-319 g/m2.24h (), signifying that these prepared nanofibers will be suitable for exuding wounds. The prepared nanofibers showed high potential to prevent moisture accumulation and dehydration, with good capability to reduce the risk of bacterial invasion.
Table 4. WVTR of NF7, NF10, and NF13 nanofibers.
3.5. In vitro antibacterial studies
The antibacterial analysis was carried out to determine the antibacterial activity of NF7, NF10, and NF13 loaded with Capparis sepiaria plant extract. The antimicrobial activity of nanofibers against various bacterial strains, including Gram-positive and Gram-negative bacteria, was evaluated by assessing their MIC values in comparison with the controls (). The NFs that had the lowest MIC values compared with the controls (Capparis sepiaria plant extract, AMP, STM, and NLD), were declared to be effective. The antibacterial results showed that NF7, NF10, and NF13 nanofibers were selective towards several bacterial strains, including Pseudomonas aeruginosa, Enterococcus faecalis, Mycobaterium smegmatis, Escherischia coli, Enterobacter cloacae, Proteus vulgaris, and Staphylococcus aureus. The nanofibers showed significant antibacterial activity against the aforementioned bacterial strain than the plant extract, streptomycin, ampicillin, nalidixic acid, and controls.
Table 5. Minimum inhibition concentration (MIC) values of the nanofibers against selected strains of bacteria.
3.6. In vitro cell viability studies
In vitro cytotoxicity was performed on the nanofibers loaded with the Capparis sepiaria plant extract (i.e. NF7, NF10, and NF13) (). The cytotoxicity of the nanofibers was evaluated by screening at the concentrations of 3.125, 6.25, 12.5, 25, 50, 100, and 200 μM of nanofibers against cells of HaCaT. The evaluation of the cytotoxicity of the nanofibers (NFs) was based on the computed cell viability percentage of each NF compared to untreated cells. NF10 induced the highest % cell viability when compared to NF7, and NF13, in all the concentrations. The % cell viability of the nanofiber formulations was above 90% at all the concentrations, revealing their non-toxicity and excellent biocompatibility.
3.7. The In vitro scratch wound healing
In an in vitro scratch assay, the NF10 nanofiber that exhibited improved hemostatic effects and a higher percentage of cell viability than other nanofibers were evaluated. The scratch assay was performed at different time intervals, namely 0 h, 24, 48, 72, and 96 h, and the results were compared to the rate of wound closure and scratch area of the untreated samples, control (commercially available wound dressing, BURN-EAZ®), and treated cells (). The HaCaT cells treated with NF10 exhibited the highest area and wound closure than the untreated cells and the commercially available wound dressing, BURN-EAZ® at 96 h (). The cells treated with NF10 exhibited the highest closure reduction of 48 ± 151%, while BURN-EAZ® and untreated cells showed closure reduction of 21 ± 230%, and 17 ± 44.4% at 96 h, respectively.
Table 6. In vitro wound scratch assay results for untreated, control, and NF10.
3.8. In vitro haemostasis
The haemostasis analysis was evaluated for Capparis Sepiaria plant extract, NF7, NF10, and NF13 to observe their capability to control bleeding. A comparison between the nanofibers and the control whole blood (WB), as displayed in , demonstrated that the nanofibers had lower absorbances than the control WB and the plant extract, indicating good capability to control bleeding. NF10 (p < 0.05, statistically significant) displayed the highest blood clotting rate when compared to other nanofibers, NF7 and NF13. The commercial wound dressing displayed better capability to control bleeding than the nanofibers, which is attributed to the physical form (i.e. gel form) of the commercial wound dressings when compared to the nanofibers (p < 0.05).
4. Discussion
The chemical structures of some of the phytochemicals identified in the Capparis Sepiara aqueous root extract are illustrated in . These phytochemicals are crucial in wound healing. Epicatechin is a natural flavonoid known for its outstanding free radical scavenging properties, antioxidant, and anti-inflammatory activities [Citation54]. It inhibits the production of matrix metalloproteinases, inhibits the production of IL-8, induces free radical detoxification enzymes, and suppresses free radical production to balance the wound microenvironment, and is effective in the treatment of chronic wounds whereby the inflammatory phase results from an overproduction of ROS and biofilm microbial infection [Citation55,Citation56]. Phloretin is a hydrophobic flavonoid that possesses excellent anti-inflammatory and anti-oxidative properties [Citation57]. It has been reported to possess an antibacterial capacity [Citation58,Citation59]. Citric acid has been reported to be effective for the treatment of chronic wound infections due to its antibacterial activity and lowering effect of the pH of the infected surfaces, inhibiting microbial growth [Citation60].
Capparis sepiara root extract was loaded into nanofiber-based dressings. Nanofibers are an important type of wound dressings due to their high wettability, antibacterial activity, anti-adherent characteristics, breathability, maintaining a moist wound environment, and ability to promote cell proliferation and support tissue repair and regeneration. These properties contribute to enhanced wound healing and patient comfort through their multifunctional properties, making nanofiber dressings a promising option in wound care management [Citation61,Citation62]. These properties are superior to those of conventional dressings, which may lack such advanced functionalities and the ability to actively respond to the wound healing process.
Electrospinning was performed at a voltage of 15 kV where the electrostatic forces are sufficient to overcome the surface tension of the polymer solution, enabling the formation of a stable jet that can be elongated and thinned into fibres. The flow rate of 10 μL/min was suitable for a steady supply of polymer solution to the tip of the needle, which is important in achieving uniform fibre diameter and preventing bead formation [Citation63,Citation64]. The voltage and flow rate directly influences the diameter and uniformity of the nanofibers. Higher voltages tend to reduce fibre diameter due to increased stretching forces, while lower flow rates can contribute to uniform fibre diameters by providing a consistent and controllable polymer jet [Citation64,Citation65]. A flow rate of 13 μL/min has been reported to be optimal, which is close to the 10 μL/min used in this study, indicating that such a flow rate is within the range that can produce fibres with desirable properties [Citation64].
The FTIR spectrum of Capparis sepiaria showed characteristic peaks similar to those reported by Saraswathi et al. [Citation66]. The FTIR of the nanofibers exhibited bands similar to PVA-SA nanofibres reported by Han et al. [Citation67] and PVA/PLGA nanofibres reported by Bootdee et al. [Citation68]. The peaks at 3303, 1603-1420, 1140 and 841 cm−1 are associated with the plant extract O-H, C-O, =C-H, and C-H, confirming the successful incorporation of the extract into the nanofibers. The nanofiber’s FTIR spectra for nanofibers incorporated with the plant extract did not reveal an interaction of the plant extract with the nanofibers.
The average diameter of the fibres NF7 was lower than NF10 and NF13. The addition of PLGA to the polymer solutions did not enhance the diameter of the N7 nanofibers. However, the addition of PLGA enhanced the thickness of the nanofibers. The addition of PLGA could have increased the surface charge density and conductivity of the polymer solution, increasing the stretching force of the jet resulting in nanofibre with a reduced diameter as seen in NF7 [Citation69]. The higher concentration of NF7 also contributed to the reduced diameter of NF7 nanofibers. Similar results were also reported by Kalluri et al. for PLGA-based nanofibers in which an increase of the polymer solution concentration to 14.5 wt% resulted in a decrease in mean fiber diameter and narrow fiber diameter distribution [Citation70]. The porous morphology of the nanofibers is crucial for cellular adhesion and proliferation, hemostasis, oxygen permeability, and absorption capability, favouring accelerated wound healing and skin regeneration [Citation19]. Surface roughness is a significant property of nanofiber mats which contributes to their cellular adhesion and proliferation capability [Citation71].
The degree of surface roughness of NF13 was higher than NF7. Asiri et al. reported a similar finding in which PVA-based nanofibers loaded with growth factor showed reduced surface roughness ranging from 418 ± 30 nm to 365 ± 11-295 ± 28 nm [Citation72]. The nanofiber’s average surface roughness range is suitable for cell attachment/adhesion to promote skin regeneration [Citation73].
The WVTR is one important feature of a wound dressing because it determines the moisture level of the wound. Wound dressings with low WVTR indicate low capacity to absorb exudates, and wound dressings with high WVTR indicate a higher absorption capacity of exudates or even dehydration [Citation74]. WVTR also provide information on water vapour permeation of wound dressing at specific relative humidity and temperatures. The WVTR range of healthy skin is 200-300 g/m2/24 h [Citation75,Citation76]. A moderate WVTR is crucial for a wound dressing because it provides a moist environment on the wound bed. High WVTR dehydrates the wound faster, causing scar formation, whereas low WVTR promotes exudate accumulation with a high risk of maceration and microbial invasion [Citation76]. The WVTR of the nanofibers was in the range of 193-319 g/m2.24h. The WVTR of commercially available wound dressings has been reported to range from 100 to 3300 g/m2/24 h [Citation77].
The antibacterial effect of the plant extract was preserved in the nanofiber formulations. The nanofibers were effective against Pseudomonas aeruginosa, Enterococcus faecalis, Mycobaterium smegmatis, Escherischia coli, Enterobacter cloacae, Proteus vulgaris, and Staphylococcus aureus strains of bacteria. These strains of bacteria have been reported to contribute to the chronicity of wounds [Citation78–82], leading to non-healing wounds. The reduced antibacterial activity of the nanofibers formulations when compared to the raw plant extract could be attributed to the release mechanism of the bioactive agent from the nanofibers and their different physical forms. However, it is important to note that incorporating plant extract into wound dressings protects the extract’s bioactive agents from degradation when in contact with environmental factors, such as heat, light, heat, etc., thereby retaining their therapeutic efficacy over a prolonged period [Citation83].
Non-toxicity and biocompatibility are crucial features of an ideal wound dressing. The nanofibers’ cytocompatibility on HaCaT was excellent, revealing their non-toxic effect. Sodium alginate has been reported to be non-immunogenic, non-toxic, and biocompatible [Citation84] which contributed to the non-toxic nature of the nanofibers. PVA and PLGA are also biocompatible, making them important polymers for the formulation of wound dressing [Citation85,Citation86]. The findings from the scratch wound healing assay revealed that NF10 loaded with Capparis Sepiaria plant extract demonstrated a substantial improvement in the rate of wound healing compared to both the control and untreated cells. The cell migration in the extract-loaded nanofiber, NF10 was significant. The wound healing capability of N10 was significant when compared to the commercially available wound dressing that is composed of a combination of tea tree leaf oil and Aloe ferox leaf extract. Cell adhesion, migration, and proliferation are crucial factors when designing wound dressings that can support wound healing and skin regeneration [Citation87]. Enhanced wound healing capability of the nanofiber, NF10 is attributed to its ability to promote cell adhesion, migration, and proliferation which was significant through the incorporation of plant extract, which lead to a conducive environment for cellular activities. The wound scratch assay showed that the NF10 wound dressing supports cell proliferation, a feature that is important in wound healing. The in vitro wound healing capability of the nanofiber is attributed to the presence of sodium alginate and the loaded plant extract in the wound dressings. The confirmed phytochemicals found in the aqueous extract of Capparis siepiara included gluconic acid, citric acid, epicatechin, and phloretin. Previous findings have illustrated that phytochemicals contribute greatly to wound healing, such as epicatechin and phloretin are known for their outstanding anti-oxidant and anti-inflammatory activities [Citation54,Citation57]. Wound dressings with anti-oxidant and anti-inflammatory activities are useful for the effective treatment of chronic wounds [Citation56]. Citric acid presence in the extract is known to lower the pH of the wound bed, inhibiting microbial growth [Citation52]. Gluconic has been identified as an antioxidant compound that contributes to the antioxidant activity in honey [Citation88]. The porous nature of nanofibers and their capability to mimic ECM have been reported to be an excellent feature that supports cell migration and proliferation [Citation89,Citation90].
The gel nature of the commercial wound dressing enhanced its capability to hold the red blood cells more than the nanofibers. Loading the plant extract into the nanofibers enhanced the capability of the nanofibers to control bleeding. The plant extract displayed a promising hemostasis effect in vitro. Hemostasis is crucial in the adhesion, activation, and accumulation of platelets, a first step in wound healing. This is also important for subsequent healing steps, such as the inflammatory phase [Citation91]. Alginate-based scaffolds have been explored as haemostatic agents due to the capability of calcium alginate to initiate an ion exchange process between the calcium ions in the alginate and the sodium ions in the blood, resulting in the activation of the platelets and blood coagulation activity [Citation92]. Nanofibers act as haemostatic agents due to their excellent antiplatelet adhesion capability induced by platelet adhesion and activation [Citation93]. Capparis extract has not been reported to exhibit a haemostatic effect but has been reported to promote wound healing [Citation94]. The significant haemostatic effect of NF10 is attributed to the high content of PVA. PVA has a significant haemostatic effect and is also safe, making it a promising material to be explored for the development of haemostatic agents [Citation95]. Wound healing is a complex biological process involving several crucial events such as inflammation, tissue formation, and remodelling. Nanofiber-based wound dressings have been reported to enhance these processes through various mechanisms such as mimicking the extracellular matrix (ECM), thereby supporting cell adhesion and proliferation, which are essential for tissue formation and wound closure [Citation96]. Additionally, the high porosity and permeability of nanofiber dressings allow for effective gas exchange and maintenance of a moist wound environment, which is beneficial for cell migration and angiogenesis [Citation97]. The hemostatic activity of the nanofibers together with their capability to inhibit bacterial invasion, makes them potential wound dressings for treating bleeding and infected wounds.
5. Conclusion
Capparis sepiaria plant extract’s therapeutic potential in wound healing has not yet been explored in wound dressings. SA/PVA/PLGA and the SA/PVA-based sodium alginate nanofibers loaded with aqueous root extract of Capparis sepiaria were synthesized using an electrospinning technique, and they exhibited smooth and porous surfaces. The nanofibers showed high % cell viability of 90% and above, revealing non-toxicity and biocompatibility. The In vitro scratch wound healing assay revealed their capability to support cell migration, a feature useful in determining wound dressings’ capability to accelerate wound closure and skin regeneration. The haemostatic effect and antimicrobial activity of the nanofibers are ideal features crucial in wound dressings for treating burn and bleeding wounds. Exploring plant-based extracts in wound dressings is an innovative approach to designing wound dressings for burn wounds to promote accelerated wound healing, treat microbial infections, and induce skin regeneration. Further research is required to comprehensively understand the mechanism of action of the prepared nanofibers loaded with Capparis sepiaria extract in wound healing.
Supplemental Material
Download MS Word (412.3 KB)Acknowledgements
The University of Fort Hare (UFH) and THRIP bursary. The opinions and views expressed in this manuscript are those of the authors and not UFH, SAMRC or THRIP.
Disclosure statement
The authors declare no conflicts of interest.
Additional information
Funding
References
- Ji X, Guo J, Guan F, et al. Preparation of electrospun polyvinyl alcohol/nanocellulose composite film and evaluation of its biomedical performance. Gels. 2021;7(4):223. doi:10.3390/gels7040223.
- Nqoro X, Taziwa R. Polymer-based functional materials loaded with metal-based nanoparticles polymer-based functional materials loaded with metal-based nanoparticles as potential scaffolds for the management of infected wounds. Pharmaceutics. 2024;16(2):155. doi:10.3390/pharmaceutics16020155.
- Mousavi SM, Hashemi SA, Zarei M, et al. Recent progress in chemical composition, production, and pharmaceutical effects of kombucha beverage: a complementary and alternative medicine. Evid Based Altern Complement Med. 2000;2000:1–14.
- Ndlovu SP, Ngece K, Alven S, et al. Gelatin-based hybrid scaffolds: promising wound dressings. Polymer. 2021;13(17):2959. doi:10.3390/polym13172959.
- Mokhena TC, Mochane MJ, Mtibe A, et al. Electrospun alginate nanofibers toward various applications: a review. Materials. 2020;13(4):934. doi:10.3390/ma13040934.
- Sun G, Zhang X, Shen Y, et al. Dextran hydrogel scaffolds enhance angiogenic responses and promote complete skin regeneration during burn wound healing. Proc Natl Acad Sci USA. 2011;108(52):20976–20981. doi:10.1073/pnas.1115973108.
- Al-Gharibi KA, Sharstha S, Al-Faras MA. Cost-effectiveness of wound care a concept analysis. Sultan Qaboos Univ Med J. 2019;18(4):e433–9–e439. doi:10.18295/squmj.2018.18.04.002.
- Rezvani GE, Khalili S, Nouri KS, et al. Wound dressings: current advances and future directions. J Appl Polym Sci. 2019;136:47738.
- Lu LC, Chang FY, Lv GZ, et al. Effectiveness and safety of compound polymyxin B ointment in treatment of burn wounds: a meta-analysis. J Burn Care Res. 2021;43(2):453–461. doi:10.1093/jbcr/irab099.
- Buyana B, Aderibigbe BA, Ray SS, et al. Development, characterization, and in vitro evaluation of water soluble poloxamer/pluronic-mastic gum-gum acacia-based wound dressing. J Appl Polym Sci. 2019;137:48728.
- Alven S, Aderibigbe BA. Hyaluronic acid-based scaffolds as potential bioactive wound dressings. Polymers (Basel). 2021;13(13):2102. doi:10.3390/polym13132102.
- Jeckson TA, Neo YP, Sisinthy SP, et al. Formulation and characterisation of deferoxamine nanofiber as potential wound dressing for the treatment of diabetic foot ulcer. J Drug Deliv Sci Technol. 2021;66:102751. doi:10.1016/j.jddst.2021.102751.
- Singh B, Kumar A, Singh B. Development of silver particle impregnated hydrogel by radiation induced cross-linking for wound dressing applications. Am J Drug Deliv Ther. 2011;5:1–7.
- Pandey VK, Ajmal G, Upadhyay SN, et al. Nano-fibrous scaffold with curcumin for anti-scar wound healing. Int J Pharm. 2020;589:119858. doi:10.1016/j.ijpharm.2020.119858.
- Hajiali H, Summa M, Russo D, et al. Alginate-lavender nanofibers with antibacterial and anti-inflammatory activity to effectively promote burn healing. J Mater Chem B. 2016;4(9):1686–1695. doi:10.1039/c5tb02174j.
- Rafiq M, Hussain T, Abid S, et al. Development of sodium alginate/PVA antibacterial nanofibers by the incorporation of essential oils. Mater Res Express. 2018;5(3):035007. doi:10.1088/2053-1591/aab0b4.
- Ndlovu SP, Fonkui TY, Ndinteh DT, et al. Dissolvable wound dressing loaded with silver nanoparticles together with ampicillin and ciprofloxacin. Ther Deliv. 2022;13(5):295–311. doi:10.4155/tde-2021-0087.
- Andleeb A, Yar M. Electrospun materials and their allied applications. In: Inamuddin, Boddula R, Ahamed MI, Asiri AM, editors. Application of electrospun materials in industrial applications. Austin, TX: Scrivener Publishing LLC; 2020. p. 215–242.
- Bi H, Feng T, Li B, et al. In vitro and in vivo comparison study of electrospun PLA and PLA/PVA/SA fiber membranes for wound healing. Polymers (Basel). 2020;12(4):839. doi:10.3390/polym12040839.
- Shahriari-Khalaji M, Hu G, Chen L, et al. Functionalization of aminoalkylsilane-grafted bacterial nanocellulose with ZnO-NPs-doped pullulan electrospun nanofibers for multifunctional wound dressing. ACS Biomater Sci Eng. 2021;7(8):3933–3946. doi:10.1021/acsbiomaterials.1c00444.
- Yao CH, Lee CY, Huang CH, et al. Novel bilayer wound dressing based on electrospun gelatin/keratin nanofibrous mats for skin wound repair. Mater Sci Eng C Mater Biol Appl. 2017;79:533–540. doi:10.1016/j.msec.2017.05.076.
- Liu Y, Zhou S, Gao Y, et al. Electrospun nanofibers as a wound dressing for treating diabetic foot ulcer. Asian J Pharm Sci. 2019;14(2):130–143. doi:10.1016/j.ajps.2018.04.004.
- Afrin A, Park M, Gwon J, et al. Alpha tocopherol-Nanocellulose loaded alginate membranes and Pluronic hydrogels for diabetic wound healing. Mater Des. 2022;224:111404. 2022 doi:10.1016/j.matdes.2022.111404.
- Pradeep HK, Patel DH, Onkarappa HS, et al. Role of nanocellulose in industrial and pharmaceutical sectors-a review. Int J Biol Macromol. 2022;207:1038–1047. doi:10.1016/j.ijbiomac.2022.03.171.
- Joshi M, Butola BS, Saha K. Advances in topical drug delivery system: micro to nanofibrous structures. J Nanosci Nanotechnol. 2014;14(1):853–867. doi:10.1166/jnn.2014.9083.
- Jin SG. Production and application of biomaterials based on polyvinyl alcohol (PVA) as wound dressing. Chem Asian J. 2022;17(21):e202200595. doi:10.1002/asia.202200595.
- Teodorescu M, Bercea M, Morariu S. Biomaterials of PVA and PVP in medical and pharmaceutical applications: perspectives and challenges. Biotechnol Adv. 2019;37(1):109–131. doi:10.1016/j.biotechadv.2018.11.008.
- Yerra ADM. Silk fibroin electrospun nanofiber blends with antibiotics and polyvinyl alcohol for burn wound healing. J Appl Polym Sci. 2022;139:51930.
- Stojko M, Włodarczyk J, Sobota M, et al. Biodegradable electrospun nonwovens releasing propolis as a promising dressing material for burn wound treatment. Pharmaceutics. 2020;12(9):883. doi:10.3390/pharmaceutics12090883.
- Hashemi SS, Saadatjo Z, Mahmoudi R, et al. Preparation and evaluation of polycaprolactone/chitosan/Jaft biocompatible nanofibers as a burn wound dressing. Burns. 2022;48(7):1690–1705. doi:10.1016/j.burns.2021.12.009.
- Kumar M, Kumar D, Garg Y, et al. Marine-derived polysaccharides and their therapeutic potential in wound healing application-a review. Int J Biol Macromol. 2023;253(Pt 6):127331. doi:10.1016/j.ijbiomac.2023.127331.
- Aderibigbe BA, Buyana B. Alginate in wound dressings. Pharmaceutics. 2018;10(2):42. doi:10.3390/pharmaceutics10020042.
- Klemm D, Cranston ED, Fischer D, et al. Nanocellulose as a natural source for groundbreaking applications in materials science: today’s state. Materials. 2018;21(7):720–748. doi:10.1016/j.mattod.2018.02.001.
- Zia T, Usman M, Sabir A, et al. Development of inter-polymeric complex of anionic polysaccharides, alginate/k-carrageenan bio-platform for burn dressing. Int J Biol Macromol. 2020;157:83–95. doi:10.1016/j.ijbiomac.2020.04.157.
- Wang Y, Ding C, Zhao Y, et al. Sodium alginate/poly(vinyl alcohol)/taxifolin nanofiber mat promoting diabetic wound healing by modulating the inflammatory response, angiogenesis, and skin flora. Int J Biol Macromol. 2023;252:126530. doi:10.1016/j.ijbiomac.2023.126530.
- Rezaei M, Nikkhah M, Mohammadi S, et al. Nano‐curcumin/graphene platelets loaded on sodium alginate/polyvinyl alcohol fibers as potential wound dressing. J Appl Polym Sci. 2021;138:50884. 2021
- Kumar M, Keshwania P, Chopra S, et al. Correction: therapeutic potential of nanocarrier-mediated delivery of phytoconstituents for wound healing: their current status and future perspective. AAPS PharmSciTech. 2023;24(7):206. doi:10.1208/s12249-023-02668-8.
- Tyavambiza C, Elbagory AM, Madiehe AM, et al. The antimicrobial and anti-inflammatory effects of silver nanoparticles synthesised from cotyledon orbiculata aqueous extract. Nanomater. 2021;11(5):1343. doi:10.3390/nano11051343.
- Biswas TK, Mukherjee B. Plant Medicines of Indian Origin for Wound Healing Activity: a Review. Int J Low Extrem Wounds. 2003;2(1):25–39. doi:10.1177/1534734603002001006.
- Zhu P, Zhang X, Wang Y, et al. Electrospun polylactic acid nanofiber membranes containing Capparis spinosa L. extracts for potential wound dressing applications. J Appl Polym Sci. 2021;138:50800.
- Zhu P, Yin H, Wei J, et al. A bilayer biocompatible polycaprolactone/zinc oxide/Capparis spinosa L. ethyl acetate extract/polylactic acid nanofibrous composite scaffold for novel wound dressing applications. Int J Biol Macromol. 2023;242(Pt 3):125093. doi:10.1016/j.ijbiomac.2023.125093.
- Wang C, Wang J, Zeng L, et al. Fabrication of electrospun polymer nanofibers with diverse morphologies. Molecules. 2019;24(5):834. doi:10.3390/molecules24050834.
- Bombin AD, Dunne NJ, McCarthy HO. Electrospinning of natural polymers for the production of nanofibres for wound healing applications. Mater Sci Eng C Mater Biol Appl. 2020;114:110994. doi:10.1016/j.msec.2020.110994.
- Fonkui TY, Ikhile MI, Njobeh PB, et al. Benzimidazole Schiff base derivatives: synthesis, characterization and antimicrobial activity. BMC Chem. 2019;13(1):127. doi:10.1186/s13065-019-0642-3.
- Ma R, Wang Y, Qi H, et al. Nanocomposite sponges of sodium alginate/graphene oxide/polyvinyl alcohol as potential wound dressing: in vitro and in vivo evaluation. Compos B Eng. 2019;167:396–405. doi:10.1016/j.compositesb.2019.03.006.
- Ahmed A, Boateng J. Calcium alginate-based antimicrobial film dressings for potential healing of infected foot ulcers. Ther Deliv. 2018;9(3):185–204. doi:10.4155/tde-2017-0104.
- Wang Y, Zhao B, Wang S, et al. Formulation and evaluation of novel glycyrrhizic acid micelles for transdermal delivery of podophyllotoxin. Drug Deliv. 2016;23(5):1623–1635. doi:10.3109/10717544.2015.1135489.
- Ma G, Fang D, Liu Y, et al. Electrospun sodium alginate/poly (ethylene oxide) core-shell nanofibers scaffolds potential for tissue engineering applications. Carbohydr Polym. 2013;87(1):737–743. doi:10.1016/j.carbpol.2011.08.055.
- Ranzato E, Patrone M, Mazzucco L, et al. Platelet lysate stimulates wound repair of HaCaT keratinocytes. Br J Dermatol. 2008;159(3):537–545. doi:10.1111/j.1365-2133.2008.08699.x.
- Strober W. Trypan blue exclusion test of cell viability. Curr Protoc Immunol. 2015;111(1):A3.B.1–A3.B.3. doi:10.1002/0471142735.ima03bs111.
- Catanzano O, D’Esposito V, Formisano P, et al. Composite alginate-hyaluronan sponges for the delivery of tranexamic acid in postextractive alveolar wounds. J Pharm Sci. 2018;107(2):654–661. doi:10.1016/j.xphs.2017.09.026.
- Seo YB, Lee OJ, Sultan MT, et al. In vitro and in vivo evaluation of the duck’s feet collagen sponge for hemostatic applications. J Biomater Appl. 2017;32(4):484–491. doi:10.1177/0885328217733338.
- Schneider CA, Rasband WS, Eliceiri KW. NIH Image to ImageJ: 25 years of image analysis. Nat Methods. 2012;9(7):671–675. doi:10.1038/nmeth.2089.
- Qu Z, Liu A, Li P, et al. Advances in physiological functions and mechanisms of (-)-epicatechin. Crit Rev Food Sci Nutr. 2021;61(2):211–233. doi:10.1080/10408398.2020.1723057.
- Viaña-Mendieta P, Sánchez ML, Benavides J. Rational selection of bioactive principles for wound healing applications: growth factors and antioxidants. Int Wound J. 2022;19(1):100–113. doi:10.1111/iwj.13602.
- Zawani M, Fauzi MB. Epigallocatechin gallate: the emerging wound healing potential of multifunctional biomaterials for future precision medicine treatment strategies. Polymers (Basel). 2021;13(21):3656. doi:10.3390/polym13213656.
- Habtemariam S. The molecular pharmacology of phloretin: anti-inflammatory mechanisms of action. Biomedicines. 2023;11(1):143. doi:10.3390/biomedicines11010143.
- Wang J, Yang R, Xiao Z, et al. Dihydrochalcones in Malus inhibit bacterial growth by reducing cell membrane integrity. Food Funct. 2020;11(7):6517–6527. doi:10.1039/d0fo00037j.
- Su H, Chen Y, Jing X, et al. Antimicrobial, antioxidant, and anti‐inflammatory nanoplatform for effective management of infected wounds. Adv Healthc Mater. 2023;13(5):e2302868. doi:10.1002/adhm.202302868.
- Hartalkar A, Nagoba B, Wadher B. Citric acid treatment of large non-healing ulcer in a patient with chronic liver disease. Eur J Gen Med. 2012;9:205–207.
- Jia J, Lin Z, Zhu J, et al. Anti-adhesive and antibacterial chitosan/PEO nanofiber dressings with high breathability for promoting wound healing. Int J Biol Macromol. 2024;261(Pt 1):129668. doi:10.1016/j.ijbiomac.2024.129668.
- Menclová K, Svoboda P, Hadač J, et al. Nanofiber wound dressing materials-a comparative study of wound healing on a porcine model. Mil Med. 2023;188(1-2):e133–e139. doi:10.1093/milmed/usab155.
- Kalluri L, Satpathy M, Duan Y. Effect of electrospinning parameters on the fiber diameter and morphology of PLGA nanofibers. Dent Oral Biol Craniofacial Res. 2021;4(2):1–19. doi:10.31487/j.dobcr.2021.02.04.
- Eroğlu AN, Tığlı Aydın RS, Karakeçili A, et al. Fabrication and process optimization of poly (2-hydroxyethyl methacrylate) nanofibers by response surface methodology. J Nanosci Nanotechnol. 2017;17(1):616–625. doi:10.1166/jnn.2017.12806.
- Feng SM, Liu XL, Qi J, et al. Effect of electrospinning parameters on morphology of polydioxanone nanofibers. Mater Res Express. 2019;6(12):125330. doi:10.1088/2053-1591/ab58e0.
- Saraswathi K, Sivaraj C, Jenifer A, et al. Antioxidant, antibacterial activities, GCMS and FTIR analysis of Ethanol bark extract of Capparis sepiaria L. Rese Jour of Pharm and Technol. 2020;13(5):2144–2150. doi:10.5958/0974-360X.2020.00385.6.
- Han X, Huo P, Ding Z, et al. Preparation of lutein-loaded PVA/sodium alginate nanofibers and investigation of its release behavior. Pharmaceutics. 2019;11(9):449. doi:10.3390/pharmaceutics11090449.
- Bootdee K, Nithitanakul M. Poly(d,l-lactide-co-glycolide) nanospheres within composite poly(vinyl alcohol)/aloe vera electrospun nanofiber as a novel wound dressing for controlled release of drug. Int J Polym Mater Polym Biomater. 2021;70(4):223–230. doi:10.1080/00914037.2019.1706512.
- Herrero-Herrero M, Gómez-Tejedor JA, Vallés-Lluch A. Role of electrospinning parameters on poly (lactic-co-glycolic acid) and poly (caprolactone-co-glycolic acid) membranes. Polymers (Basel). 2021;13(5):695. doi:10.3390/polym13050695.
- Duan Y, Kalluri L, Satpathy M, et al. Effect of electrospinning parameters on the fiber diameter and morphology of PLGA nanofibers. DOBCR. 2021;4:1–7. doi:10.31487/j.DOBCR.2021.02.04.
- Zamani F, Amani-Tehran M, Latifi M, et al. The influence of surface nanoroughness of electrospun PLGA nanofibrous scaffold on nerve cell adhesion and proliferation. J Mater Sci Mater Med. 2013;24(6):1551–1560. doi:10.1007/s10856-013-4905-6.
- Asiri A, Saidin S, Sani MH, et al. Epidermal and fibroblast growth factors incorporated polyvinyl alcohol electrospun nanofibers as biological dressing scaffold. Sci Rep. 2021;11(1):5634. doi:10.1038/s41598-021-85149-x.
- Ranjbar-Mohammadi M, Shakoori P, Arab-Bafrani Z. Design and characterization of keratin/PVA-PLA nanofibers containing hybrids of nanofibrillated chitosan/ZnO nanoparticles. Int J Biol Macromol. 2021;187:554–565. doi:10.1016/j.ijbiomac.2021.07.160.
- Nuutila K, Eriksson E. Moist wound healing with commonly available dressings. Adv Wound Care (New Rochelle). 2021;10(12):685–698. doi:10.1089/wound.2020.1232.
- Ribeiro AS, Costa SM, Ferreira DP, et al. Chitosan/nanocellulose electrospun fibers with enhanced antibacterial and antifungal activity for wound dressing applications. React Funct Polym. 2021;159:104808. doi:10.1016/j.reactfunctpolym.2020.104808.
- Naseri-Nosar M, Ziora ZM. Wound dressings from naturally-occurring polymers: a review on homopolysaccharide-based composites. Carbohydr Polym. 2018;189:379–398. doi:10.1016/j.carbpol.2018.02.003.
- Ahmad N, Tayyeb D, Ali I, et al. Development and characterization of hemicellulose-based films for antibacterial wound-dressing application. Polymers (Basel). 2020;12(3):548. doi:10.3390/polym12030548.
- Pouget C, Dunyach-Remy C, Magnan C, et al. Polymicrobial biofilm organization of Staphylococcus aureus and Pseudomonas aeruginosa in a chronic wound environment. Int J Mol Sci. 2022;23(18):10761. doi:10.3390/ijms231810761.
- Chong KKL, Tay WH, Janela B, et al. Enterococcus faecalis modulates immune activation and slows healing during wound infection. J Infect Dis. 2017;216(12):1644–1654. doi:10.1093/infdis/jix541.
- Oliveira A, Sousa JC, Silva AC, et al. Chestnut honey and bacteriophage application to control Pseudomonas aeruginosa and Escherichia coli biofilms: evaluation in an ex vivo wound model. Front Microbiol. 2018;9:1725. doi:10.3389/fmicb.2018.01725.
- Bennett W, Mende K, Campbell WR, et al. Enterobacter cloacae infection characteristics and outcomes in battlefield trauma patients. PLoS One. 2023;18(8):e0290735. doi:10.1371/journal.pone.0290735.
- Majtan J, Bohova J, Horniackova M, et al. Anti‐biofilm effects of honey against wound pathogens Proteus mirabilis and Enterobacter cloacae. Phytother Res. 2014;28(1):69–75. doi:10.1002/ptr.4957.
- McClements DJ. Advances in nanoparticle and microparticle delivery systems for increasing the dispersibility, stability, and bioactivity of phytochemicals. Biotechnol Adv. 2020;38:107287. doi:10.1016/j.biotechadv.2018.08.004.
- Ahmad A, Mubarak NM, Jannat FT, et al. Critical review on the synthesis of natural sodium alginate based composite materials: An innovative biological polymer for biomedical delivery applications. Processes. 2021;9(1):137. doi:10.3390/pr9010137.
- Sharma P, Kumar AP, Singh VK, et al. Comprehensive review on properties of polyvinyl alcohol (PVA) crosslinked with carboxylic acid. J Mater Environ Sci. 2023;10:1236–1252.
- Elmowafy EM, Tiboni M, Soliman ME. Biocompatibility, biodegradation and biomedical applications of poly (lactic acid)/poly (lactic-co-glycolic acid) micro and nanoparticles. J Pharm Investig. 2019;49(4):347–380. doi:10.1007/s40005-019-00439-x.
- Negut I, Dorcioman G, Grumezescu V. Scaffolds for wound healing applications. Polymers (Basel). 2020;12(9):2010. doi:10.3390/polym12092010.
- Wang L, Wang C, Zhou L, et al. Fabrication of a novel three-dimensional porous PCL/PLA tissue engineering scaffold with high connectivity for endothelial cell migration. Eur Polym J. 2021;161:110834. doi:10.1016/j.eurpolymj.2021.110834.
- Shamsudin S, Selamat J, Sanny M, et al. Integrated gas chromatography–mass spectrometry and liquid chromatography-quadruple time of flight-mass spectrometry-based untargeted metabolomics reveal possible metabolites related to antioxidant activity in stingless bee honey. Food Anal Methods. 2022;15(11):3209–3224. doi:10.1007/s12161-022-02271-w.
- Kennedy KM, Bhaw-Luximon A, Jhurry D. Cell-matrix mechanical interaction in electrospun polymeric scaffolds for tissue engineering: implications for scaffold design and performance. Acta Biomater. 2017;50:41–55. doi:10.1016/j.actbio.2016.12.034.
- Li R, Liu K, Huang X, et al. Bioactive materials promote wound healing through modulation of cell behaviors. Adv Sci. 2022;9:2105152.
- Ghimire S, Sarkar P, Rigby K, et al. Polymeric materials for hemostatic wound healing. Pharmaceutics. 2021;13(12):2127. doi:10.3390/pharmaceutics13122127.
- Wan LS, Xu Z. Polymer surfaces structured with random or aligned electrospun nanofibers to promote the adhesion of blood platelets. J Biomed Mater Res A. 2009;89(1):168–175. doi:10.1002/jbm.a.31907.
- Quadri S, Pant V, Kumar V. An introduction to ethnomedicinal and pharmacological activity of Capparis sepiaria L. Pharm Innovation. 2023;10:12–24.
- Dorkhani E, Faryabi A, Noorafkan Y, et al. Biomedical properties and hemostatic efficacy of polyvinyl alcohol (PVA) based hydrogel in experimental rat liver injury model. J Appl Biomater Funct Mater. 2023;21:1–8.
- Li T, Sun M, Wu S. State-of-the-art review of electrospun gelatin-based nanofiber dressings for wound healing applications. Nanomaterials. 2022;12(5):784. doi:10.3390/nano12050784.
- Liu S, Wu G, Wang W, et al. In situ electrospinning of “dry-wet” conversion nanofiber dressings for wound healing. Mar Drugs. 2023;21(4):241. doi:10.3390/md21040241.