ABSTRACT
In the last two decades nanogels have emerged as very promising and versatile biomaterials suitable for a wide range of applications. Their features such as large surface area, ability to hold molecules, flexibility in size and water-based formulations have ensured them great recognition as drug delivery systems with various in vivo applications which have confirmed their potential. On the other handnanogels have been investigated in recent years for applications in various fields other than biomedicine. Clear examples of this are represented by the possibility of employing nanogels as sensing materials, as catalysts or as adsorbents for environmental applications. In view of this variety of possible applications, in this work we extend our knowledge on the topic of their possible uses described in literature, taking stock of the state of the art for all possible nanogel employment and their synthesis methods.
Introduction
In recent decades, the old dream of natural science to manipulate matter locally on the atomic and molecular scale has become reality and many commercial products are also available on the market [Citation1–3]. The introduction of nanotechnology into many different fields allows the development of alternative tools to achieve specific goals and implement strategies, accepting the very complex scientific, societal and ethical challenges [Citation4]. Nanotechnology is defined by the US National Nanotechnology Initiative as it is concerned with materials and systems whose structures and components exhibit novel and significantly improved physical, chemical and biological properties, phenomena and processes due to their nanoscale size. This means that qualitatively new and advantageous properties emerge from the materials at the nanoscale. Indeed nanomaterials possess unique physical and chemical properties such as thermal or electrical conductivity, strength, durability and chemical reactivity [Citation5]. In the last two decades, nanogels have emerged as very promising and versatile biomaterials suitable for a wide range of applications. Their features such as large surface area, ability to hold molecules, flexibility in size and water-based formulations have ensured them great recognition as drug delivery systems with various in vivo applications which have confirmed their potential [Citation6–8]. On the other hand, because of their tuneable and versatile characteristics, nanogels have been explored in recent years for various applications in very different fields [Citation9–11]. A clear example of this is represented by the possibility of employing nanogels as sensing materials. In fact, one of the most important properties of nanogels is their ability to respond to external stimuli such as temperature or pH variations, but also recognition of specific molecules [Citation12,Citation13]. This behaviour can be tuned by working on the formulation and the functionalization of nanogels and consequently this kind of strategy can represent a versatile approach to face many challenges in the sensors field. Another possible and valuable application of nanogels is represented by their functionalization to mimic natural enzymes that can be used as catalysts [Citation14,Citation15].
Novel artificial enzymes are now attracting great interest because of their high efficiency and controllability, properties that can be useful to overcome drawbacks of many conventional catalytic systems [Citation16]. Without doubt nanogels can be very useful in this innovative strategy thanks to their easy production and functionalization method [Citation17–19]. Besides the large surface area, porosity and encapsulation ability, nanogels have been found to be very useful in environmental applications for the removal of organic contaminants. These molecules can be entrapped inside the nanogel framework thanks to the aforementioned properties and subsequently be removed from a certain environment [Citation20,Citation21]. The versatility and flexibility of nanogels guarantee their employment in a very different context with various types of contaminants. Moreover, during the last year nanogels have been exploited even in the controlled release of agrochemicals such as pesticides or fungicides, combining the knowledge concerning these devices derived from the drug delivery fields with innovative environmental applications [Citation22,Citation23]. In view of this variety of possible applications of functionalized nanogels, we have decided to extend our knowledge on their possible uses described in the literature and to write this review to take stock of the state of the art for all possible uses of functionalized nanogels and their synthesis methods together with all the challenges that should be addressed before reaching the final market.
Nanogel definition and properties
The name ‘nanogel’ was introduced for the first time by Vinogradov et al. in 1999 to describe colloidal systems consisting of a hydrophilic polymeric network, synthesized by cross-linking polyethylene glycol (PEG) and polyethyleneimine (PEI), which can be used as drug delivery systems [Citation24]. From then on, many different developments took place in this field, various formulations have been developed and multiple in vitro and in vivo applications have been tested [Citation25,Citation26]. Moreover, over the years, the employment of nanogels has spread across various areas and working fields thanks to their multiple properties [Citation27].
Nowadays nanogels are commonly identified as aqueous dispersions of hydrogel particles, either chemically or physically cross-linked, made of polymeric chains in the nanoscale range [Citation28]. Their formulation makes them usually biocompatible and biodegradable and their ability to absorb and release molecules guarantees their employment in multiple fields such as drug delivery, but also water decontamination, catalysis and sensors [Citation29,Citation30]. In the following list, we point out the key features of nanogels, to highlight the reasons behind the widespread recognition and attention they are acquiring in today’s world.
Nanometric dimensions: this is, without doubt, one of the most important characteristics of nanogels that enhances the surface area to volume ratio. Moreover, in many drug delivery applications, nanometric dimensions are required to cross biological barriers, penetrate tissues and perform in situ drug delivery [Citation27].
Loading and absorbent ability: nanogels can easily encapsulate drugs and active compounds exploiting covalent conjugation, chemical linking or physical entrapment [Citation28]. This feature is extremely important both for drug encapsulation in drug delivery applications and for the employment of these materials as absorbent systems for organic dyes or metal ions [Citation20].
Molecule release: the encapsulated molecules and drugs can be properly released using various mechanisms such as diffusional release, release driven by polymer degradation or as a response to an external stimulus like temperature or pH [Citation31].
This feature is obviously of great interest for drug delivery applications and the design of nanogels can be tuned according to the desired behaviour.
Swelling behaviour: when the inner polymeric framework of nanogels comes in contact with the solvent, it enlarges due to solvent molecule penetration and the system undergoes a subsequent volume increase [Citation32]. The swelling and de-swelling process can be tuned by working on the structural parameters of the system, such as the cross-linking degree, mesh size, or presence of proper functional groups and it can be influenced by external stimuli such as pH, temperature, light stimuli or interactions with specific molecules [Citation33]. Therefore, this feature can be exploited extensively and investigated for nanogel sensing applications.
Viscoelasticity: the structure of nanogels is commonly highly solvated and therefore this material usually exhibits both viscous and elastic characteristics guaranteeing flow through needles and extracellular matrix [Citation34].
Colloidal stability: the three-dimensional framework of nanogels has been demonstrated to be highly stable even in biological fluids and therefore their employment is often preferred to micelles and other colloidal systems [Citation34].
Tuneable behaviour: as partially mentioned already in this section, nanogels are extremely versatile and tuneable materials [Citation35]. Their design can be modified according to the desired employment, the core formulation or the surface functionalization of the material can be properly changed, obtaining proper responsive behaviour or biological features that are essential for the intended applications [Citation18].
Nanogel classification
Nanogels are generally classified into different categories depending on their polymeric composition, structure, type of linkage and finally even depending on their ability to respond to external stimuli. Here we report the main divisions that can be found in literature [Citation34].
Polymer-based classification
Starting from the first-mentioned classification (polymeric composition), nanogels can be divided according to the polymers that constitute their framework [Citation36]. A clear example of these is polyether – and polyester-based nanogels, which are those employed most in the pharmaceutical field thanks to their biodegradability and biocompatibility. Their chains are formed by covalent linkage of monomers to aether (R–O–R) and ester (R–C–O–O–R) groups that can be found in the structure [Citation25].
In recent years polyethylene glycol (PEG) drew great interest in the area of drug delivery thanks to its high biocompatibility, high water solubility and biological inertness and it has been employed extensively in the synthesis of nanogels [Citation37]. Moreover, PEG can also reduce endocytosis, phagocytosis and hepatic uptake thanks to its hydrophilicity which makes it possible to increase the circulation time and the stability of those systems [Citation37]. Another very important family of polymers used in nanogel synthesis are the polypeptides, biodegradable polymers with amino acid sequences which are altered during synthesis. The nanogels formulated based on these chains showed important loading and delivery ability of low molecular weight agents [Citation38]. Moreover, many polypeptides, such as poly-L-lysine or water-soluble polyglutamic acid exist in D/L optically active forms. Polyacrylates are another example of building material for nanogels. They are acrylic acid-based polymers with a change in their vinyl or carboxyl hydrogens. Commonly these systems are negatively charged when exposed to a specific temperature, pH conditions and charges, and because of the interchange of counterions with the external medium, they have shown the ability to swell. Because of these features, polyacrylate nanogels are commonly involved in responsive applications [Citation34]. Other examples of nanogel classification based on the constituent polymers are poloxamer-based nanogels, which employ this kind of blocked copolymers consisting of both hydrophilic and hydrophobic chains or polysaccharide-based polymers, formed by the linkage of long-chain monosaccharide units or glycosidic bonds whose derivatives show structural and functional diversity due to their multiple reactive groups such as –OH, –NH2 and COOH [Citation36,Citation39].
In the following we report the chemical structure of the most common polymers employed in nanogel synthesis.
Linkage based classification
Based on the type of linkages present in the polymeric network of the system, nanogels can be classified into two main groups, namely non-covalent linkage and covalent linkage nanogels [Citation40,Citation41]. Those characterized by non-covalent linkages, which are also called physically cross-linked nanogels, can be formed by van der Waals forces, hydrogen or hydrophobic bonding and electrostatic interactions [Citation42]. These types of systems are synthesized with various procedures in which operations such as the conjunction of amphiphilic blocks, self-assembly, polymeric chain agglomeration and oppositely charged polymeric chain incorporation are conducted. Liposome-modified nanogels are examples of physically cross-linked nanogels that can be produced by linkage between copolymers and liposomes which enable them to respond to pH and temperature stimuli [Citation43].
These systems have been studied both in transdermal drug delivery applications and as sensing devices. Similarly, micellar nanogels are another valuable example of physically cross-linked nanogels [Citation44]. They are usually produced with linked copolymers in aqueous solution or by self-assembly of hydrophilic and hydrophobic blocks. The presence of a hydrophilic shell and hydrophobic core is extremely important for drug delivery. On the other hand, those nanogels produced by chemical reactions with the formation of covalent bonds thanks to the action of cross-linking agents, are commonly named covalent linkage nanogels [Citation45]. The use of properly selected cross-linking agents, the tuning of their quantities and the ratio with respect to the reagents allow tuning the mesh size and cross-linking degree of the particles and, their morphology and dimensions which are pivotal parameters for any application [Citation46].
Structure-based classification
Another very important way to classify nanogels considers the structural peculiarities of the system. Nanogels in fact are often identified as particles with a spherical shape but using specific modifications on the synthesis and the formulation of the particles, different shapes and characteristics can be obtained [Citation47]. A clear example of that is represented by hollow nanogels which are systems with a hollow cavity in their gel [Citation48–50] They are commonly prepared in two stages. First, the polymers are cross-linked with core–shell particles like silicon dioxide, gold or hydroxyl propyl cellulose, and then, the core–shell moieties are carefully removed by pH gradient or by anti-solvent associate precipitation techniques. A precise removal is pivotal to obtain the desired cavity in the system [Citation51]. This specific structure enhances the surface area in nanogels and makes them suitable for various applications both in drug delivery and in water decontamination or catalysis where properly designed cavities are essential. Another category of nanogels with specific structures is the multi-layered type. These are mostly used in delivering highly toxic drugs or body fluid sensitive drugs as well as in sensing applications and exploit the multiple structures of the system [Citation52].
These devices are usually formed by a single polymer or multiple polymers chemically cross-linked. The presence of multiple layers guarantees the possibility of combining different chemical structures in the same device that can be properly set during the synthesis layer by layer [Citation53]. Core–shell nanogels without doubt represent another important family of these kinds of systems [Citation33]. They are characterized by the presence of an inner core, generally made of metallic or bimetallic materials, nanorods, or carbon dots, with an external shell able to cover and hold the inner moieties. The external portion can present different natures, such as polymeric chains but also organic structures and the positioning over the system can be achieved by chemical bonding, such as covalent linkages, but also by physical entrapment [Citation54]. The possibility of tuning the system according to the desired application is extremely effective and can be the turning point for many applications both in the drug delivery field where proper release of payloads can be achieved [Citation55]. This can also represent a winning point in sensors, where a properly designed external shell can induce precise responsive behaviour to the system [Citation56].
Nanogel synthesis
A key aspect for nanogel use in commercial products is also related to their large-scale production that is pivotal for their reproducible and consistent production. It is well-known that nano-object formation is much easier at the laboratory scale than at large scale, where bulk properties disfavour the formation of new surfaces. Consequently, pilot technologies should be thoroughly investigated starting from the method of synthesis and then considering aggregation mechanisms that will compromise all the production efforts. The design and synthesis of nanogels are crucial steps in the development of effective systems that can be employed in various applications. Commonly, as reported by Kabanov et al, the synthesis methods for nanogels are divided into four main categories: (i) physical self-assembly, (ii) polymerization of monomers in homogenous phase or nanoscale heterogeneous system, (iii) cross-linking of preformed polymeric chains and (iv) template-assisted nanofabrication [Citation25].
Physical self-assembly is certainly one of the most versatile and employed procedures for synthesizing nanogels. Commonly, hydrophilic polymers are assembled in aqueous systems exploiting hydrophobic or electrostatic interactions or using hydrogen bonding between polymeric chains [Citation27]. The tuning of the ratio between the molar quantities of the polymers and the reaction parameters (e.g. temperature, pH value) guarantees the possibility of tuning many features of nanogels, such as dimensions, mesh size and structures. On the other hand, the polymerization of monomers in the homogenous phase or nanoscale heterogeneous phase has been largely discussed in the literature and represents another valuable synthesis method for nanogels [Citation9,Citation46]. This method exploits bifunctional monomers as cross-linking systems to guarantee stability of the final nanogel matrix. The features of the final gel structure can be tuned by working on the reaction system, and this certainly represents a great advantage compared to other methodologies and it guarantees great versatility for the desired system. A clear example of this is represented by the possibility of introducing specific bonds inside the nanogels [Citation57]. Such bonds can be stronger or weaker depending on the final application of the system and the proper design of these moieties guarantees specific behaviour of the nanogel (e.g. possibility of dissolution in specific ions or pH conditions) that can be effective both for drug delivery applications and for sensors [Citation33]. Another effective synthesis strategy for nanogels is the covalent cross-linking of preformed polymers [Citation36]. This technique is definitely one of the most employed, as widely reported in the literature, thanks to its versatility and the multiple formulations that can be obtained. Moreover, the polymeric chains involved can be functionalized with chemical groups both before and after the formation of the gel. This is certainly one of the most important characteristics of this technique since it guarantees the possibility of conferring on nanogels the desired features for their intended applications.
Another novel nanogel fabrication method uses supramolecular host–guest interactions [Citation58]. This strategy has been reported to be extremely effective for the synthesis of responsive nanogels that can be employed both in targeted drug delivery applications and in sensors, thanks to their binding ability and in specific recognition thanks to the key feature of host–guest chemistry [Citation59].
Finally, in recent years, innovative and extremely precise technology for nanoparticle and nanogel production has been developed, which is commonly called particle replication in nonwetting templates (PRINT technology) [Citation60]. In this method, monodisperse particles are obtained starting from various different material matrixes. Moreover, precise control of various parameters such as size, shape, structure, composition, cross-linking degree and functional moieties is possible and similarly, precise encapsulation of drugs and active molecules can be performed during the synthesis [Citation61]. shows the chemical and physical crosslinking reactions for the synthesis of nanogels.
Figure 2. Illustration of chemical (A) and physical (B) crosslinking reactions for the synthesis of nanogels. (A) The formation of covalent bonds (crosslinking point, in red) is visible between the reactive moieties (yellow and green) of polymers X and Y. (B) The physical interactions between polymers W and Z ensure the formation of the nanosystems. Reprinted from Ref. [Citation27] with permission from MDPI.
![Figure 2. Illustration of chemical (A) and physical (B) crosslinking reactions for the synthesis of nanogels. (A) The formation of covalent bonds (crosslinking point, in red) is visible between the reactive moieties (yellow and green) of polymers X and Y. (B) The physical interactions between polymers W and Z ensure the formation of the nanosystems. Reprinted from Ref. [Citation27] with permission from MDPI.](/cms/asset/8d16fcaa-7695-4be6-8281-e177f253d3da/yimr_a_2026864_f0002_oc.jpg)
Nanogel functionalization
As already mentioned, one of the most important features of nanogels is the possibility of functionalizing their structure, exploiting specific molecules, polymers or organic systems to obtain specific behaviour for the final particle. This characteristic is certainly one of the greatest benefits of nanogels and one of the explanations for their widespread dissemination now [Citation18]. Nanogel functionalization can be performed in two main ways; nano-systems working with previously functionalized polymers can be synthesized or the surface or the structure of the nanoparticles can be functionalized once they have been synthesized [Citation62]. This step is often a pivotal point to obtain an effective system for the intended application: proper biological behaviour for drug delivery systems, specific responsive behaviour for sensors applications or the selective binding ability for devices employed in environmental uses are all affected considerably by nanogel functionalization [Citation63,Citation64]. Functionalization after nano-object formation guarantees the absence of conflicts during nanogel production on one hand, but it requires a further step on the other hand. This can complicate the colloid scale up that, as said above, is a key step in reaching the market with commercial products. So in theory, functionalization during nanogel formation presents many advantages (single step, easy production) but the need to avoid the use of groups that can compete or compromise colloid formation is pivotal. In literature, various techniques are reported and detailed reviews on the work are available [Citation65,Citation66]. In this section, we are going to give an overview of the main functionalization strategies that can be employed. First, polymeric functionalization represents an effective and well-known strategy for nanogel functionalization. Polymers present long chains with various chemical groups that can be employed to introduce specific functional groups in the structure of the nanogels to determine the desired behaviour of the final system [Citation37]. The presence of polymeric chains over the surface of the system prevents surface agglomeration of nanoparticles and they can increase their stability.
Natural polymers are preferred in the case of biomedical applications to ensure biocompatibility, while conductive and responsive polymers are employed, both during synthesis and as functional moieties, especially for sensing applications [Citation67]. Similar strategies can be employed with other molecules, exploiting for example nucleophilic substitution over the constituent polymeric chains of the nanogels, introducing specific chemical groups in the framework [Citation68]. This permits the tuning of many features of nanogels, such as hydrodynamic diameter or zeta potential, and obtains specific responsive behaviour for example by introducing labile bonds or moieties responsive to specific external conditions. On the other hand, the presence of certain functional groups is pivotal for guaranteeing the employability of final devices for example in environmental applications, to modulate and increase the binding ability of the system with specific molecules and pollutants [Citation69]. Cell membrane coating is another surface functionalization technique that can be very useful especially in biomedical applications [Citation70,Citation71]. This technique uses natural membranes from cells that are fixed on pre-synthesized nanoparticles. It is clear how the use of a specific membrane strongly influences the characteristics of the final system and determines its application. This strategy is commonly employed for drug delivery applications of nanogels thanks to the biocompatibility of these systems and the strong influence that can be created working with this kind of functionalization on the in vivo behaviour of these devices [Citation72].
Surface functionalization with antibodies, thanks to their structure and biological role, and hybridized DNA structures functionalization, considering their specificity and non-immunogenicity, are certainly alternative effective strategies that can be employed for targeted drug delivery applications which can improve the selectivity and the efficacy of these systems [Citation18]. Very often the encapsulation of external systems inside nanogels before their real use is regarded as a typology of functionalization. In the context of interest of this work, metallic nanoparticles encapsulated inside nanogels are extensively employed in catalysis and gel frameworks have been demonstrated useful to support the catalyst and promote transport phenomena which will be discussed in detail in the next section [Citation73].
In the following we have summed up the previously explained strategies, highlighting their advantages and disadvantages.
Table 1. Advantages and disadvantages of the functionalization strategies for nanogels.
A schematic illustration of possible nanogel functionalization strategies is shown in with a consequent specific property (here cell selectivity) added to the neat colloidal system.
Figure 3. Schematic illustration of various nanogel functionalization strategies and their use in different applications. Reprinted from Ref. [Citation18] with permission from MDPI.
![Figure 3. Schematic illustration of various nanogel functionalization strategies and their use in different applications. Reprinted from Ref. [Citation18] with permission from MDPI.](/cms/asset/fcc66ad2-934e-4d53-ab04-39ee2f2213f2/yimr_a_2026864_f0003_oc.jpg)
Applications of functionalized nanogels
In this section we give an overview of the dissemination acquired by nanogels, focusing on their applications, starting from the biomedical field and then moving on to innovative employment such as uses in the world of catalysis, water purification and sensors.
Biomedical applications of nanogels
As already mentioned in the previous section, nanogels have come to the fore in recent years as innovative drug delivery systems to overcome the limitations of conventional methods of drug administration [Citation33]. One of the most serious diseases of the human body is without doubt cancer. Various approaches have been developed to treat this pathology, such as surgery, radiotherapy and targeted therapy [Citation74,Citation75]. Here nanogels have attracted great attention for their employment as targeted drug delivery systems. Proper formulation and functionalization of the particles can be a successful strategy for targeting cancer cells in the body, avoiding many side effects of other treatments [Citation76]. Breast cancer is an example of a pathology that can be treated working with functionalized nanogel as drug delivery systems.
In this direction dextrin nanogel functionalization with AMD3100 (plerixafor) guarantees high cell targeting through the CXCR4 chemokine receptor. Then the ability of nanogels to carry and release doxorubicin showed high efficacy of this system with promising anticancer and anti-metastatic effects [Citation77]. Similarly, dual-targeted systems can be employed in this kind of application. Indeed an epidermal growth factor receptor and protein CD44 dual-targeted hyaluronic acid nanogel were successfully synthesized with saporin loaded inside their structure [Citation78]. In vivo tests were successfully employed in breast cancer to inhibit tumour metastasis limiting the side effects as reported in .
Figure 4. In vivo inhibition of 4T1-luc breast cancer metastasis by Saporin-EGFR/CD44-Nanogels. (A) Bioluminescence imaging of the tumour metastasis in vivo. (B) Quantification of the average luminescence levels of mice treated with different formulations on different days (5, 9 and 13). (C) Mean number of metastatic nodules on the lung tissue of mice treated with different formulations. (D) Total weight of lung and heart of mice treated with different strategies. Reprinted from Ref. [Citation78] with permission from Elsevier.
![Figure 4. In vivo inhibition of 4T1-luc breast cancer metastasis by Saporin-EGFR/CD44-Nanogels. (A) Bioluminescence imaging of the tumour metastasis in vivo. (B) Quantification of the average luminescence levels of mice treated with different formulations on different days (5, 9 and 13). (C) Mean number of metastatic nodules on the lung tissue of mice treated with different formulations. (D) Total weight of lung and heart of mice treated with different strategies. Reprinted from Ref. [Citation78] with permission from Elsevier.](/cms/asset/c1fd2053-a41c-4c8e-b5bb-9eda14e398a1/yimr_a_2026864_f0004_oc.jpg)
Other kinds of cancer diseases have been successfully treated using nanogels. For example, cholesterol-bearing pullulan-based nanogels loaded with IL-12 were seen to induce growth retardation of fibrosarcoma [Citation79]. Similarly, the possibility of suppressing neovascularization and growth of renal cell carcinoma in a mouse model was found working with a cholesterol-bearing cycloamylose using a spermine group linked to nanogel surface for the delivery of vascular endothelial growth factor-specific siRNA [Citation80].
The spermine functionalization permits excellent performance in terms of selectivity towards renal cell carcinoma. It is clear how in each case the proper formulation of the nanogel is essential to obtain the desired delivery effect. Zwitterionic polysulphamide nanogels modified with transferrin for the loading of doxorubicin are another effective example of this. In vivo assays demonstrated the targeting properties of this formulation and the beneficial effects on cancer chemotherapy [Citation81]. Similarly, the targeting delivery ability of nanogels can be used for localized delivery to glioblastoma cells for immunotherapy. This can be achieved using thermoreversible poly(ethylene glycol)-g-chitosan nanogels optimized for the release of T lymphocytes [Citation82]. During biological assays these systems demonstrated great ability in killing glioblastoma cells and considerable stability, both properties that represent valuable tools for localized immunotherapy in brain cancer.
Cancer is not the only pathology that can be treated with functionalized nanogels. In fact, these innovative delivery systems have found great applications in all those cases in which a precise delivery is required and conventional routes of administration of drugs are not effective. One of these applications is certainly represented by spinal cord injury and, in general, all those diseases that involve the central nervous systems [Citation64]. This very complex and essential part of the human body is characterized by many barriers (e.g. blood–brain barrier) that limit the molecules and substances that can penetrate through them. Studies have now been conducted on active molecules and innovative drug delivery strategies that can act as trojan horses, thus penetrating inside the central nervous systems and performing as selective in situ delivery of the required drugs [Citation83].
In this context in recent years, polyethylene-polyethylenimine nanogels, coated with primary amines loaded with anti-inflammatory drug (rolipram) have been synthesized and tested for the selective targeting of activated astrocytes [Citation84]. In vivo tests showed the efficacy of functionalized devices in the selective targeting of those cells, improving motor performance after the lesion and reducing the subsequent inflammation state. Alzheimer’s disease represents another neurodegenerative disease (chronic) and therefore another serious pathology that involves the brain.
In recent years the insulin dysfunction has been correlated in some cases with Alzheimer, and because of this, the administration of insulin can be employed today in Alzheimer treatment [Citation85]. Carbonyl-functionalized poly(N-vinyl pyrrolidone) nanogel, obtained through ionizing radiation then conjugated with insulin, is a valuable example of this. This formulation can bind to insulin receptors, activating insulin signalling and consequently promoting its transport across the blood–brain barrier so that insulin can be delivered for Alzheimer treatment [Citation86].
Another important application of nanogels is the delivery of urokinase, a serine protease extremely effective in thrombolytic therapies. pH-sensitive PEG-conjugated urokinase nanogels have been developed for thrombolytic treatment [Citation87]. The design of the system guarantees the release of urokinase when the pH decreases, a condition that occurs in thrombolytic events due to lack of oxygen caused by microcirculatory clots. In particular, the role of surface functionalization is to improve the ischaemic brain tissue and protect the blood–brain barrier by inhibiting apoptosis and decreasing neurotoxicity of the entire system. Nanogel formulations can also be employed in the treatment of cardiovascular diseases. In this direction N-Isopropylacrylamide-methyl methacrylate nanogel were developed for the delivery of N, α-L-rhamnopyranosyl vincosamide with important cardioprotective properties, reducing cardiac toxicity in doxorubicin-induced toxicity models [Citation88].
Similarly, a cationic cholesteryl-group-bearing pullulan (cCHP) nanogel loaded with angiotensin II type 1 receptor (AT1R) and pneumococcal surface protein A (PspA) has been developed to treat hypertension and pneumococcal infections [Citation89].
The biological assays demonstrated the efficacy of AT1R to reduce the blood pressure in rat models, while the PspA was able to induce immunization against Streptococcus pneumoniae. These results confirmed the efficacy of this vaccine in protecting from this infection and attenuating hypertension [Citation89]. Wound healing is a biological process that guarantees the replacement of destroyed or damaged tissue in the human body with a new one. This process can very often be improved with drugs and active molecules to promote fast regeneration or to prevent infections and it is clear how their precise delivery is essential to guarantee greater effectiveness [Citation90].
Chitosan-based nanogels, loaded with antibacterial drug (silver sulphadiazine), have been effectively employed in healing burn wounds. Similarly, lysine-based nanogels, loaded with chlorhexidine diacetate (antiseptic molecule) were encapsulated inside a hydrogel based on aminoethyl methacrylate hyaluronic acid and methacrylate methoxy polyethylene glycol [Citation91]. In vivo tests confirmed the antibacterial efficacy of those systems and their benefits when employed in wound healing as reported in the following .
Figure 5. (A) Schematization of the encapsulation of the lysine-based nanogels, loaded with chlorhexidine diacetate, inside an aminoethyl methacrylate hyaluronic acid and methacrylate methoxy polyethylene glycol hydrogel. The antibacterial effect of the system, with its ability in control of bleeding and wound healing is shown. (B) Photographs of wounds treated by the control, Gel-1, CHX-loaded Gel-1, and Gel-1@CLN hydrogel samples (scale bar of 5 mm). (C) Assessment of the wound size reduction. Reprinted from Ref. [Citation91] with permission from American Chemical Society. CHX, chlorhexidine diacetate, Gel-1, hybrid hydrogels with different Mw values of polyethylene glycol hydrogel, CLN, CHX-loaded nanogels.
![Figure 5. (A) Schematization of the encapsulation of the lysine-based nanogels, loaded with chlorhexidine diacetate, inside an aminoethyl methacrylate hyaluronic acid and methacrylate methoxy polyethylene glycol hydrogel. The antibacterial effect of the system, with its ability in control of bleeding and wound healing is shown. (B) Photographs of wounds treated by the control, Gel-1, CHX-loaded Gel-1, and Gel-1@CLN hydrogel samples (scale bar of 5 mm). (C) Assessment of the wound size reduction. Reprinted from Ref. [Citation91] with permission from American Chemical Society. CHX, chlorhexidine diacetate, Gel-1, hybrid hydrogels with different Mw values of polyethylene glycol hydrogel, CLN, CHX-loaded nanogels.](/cms/asset/0fae5e80-632f-47a5-a956-a193e1fc0299/yimr_a_2026864_f0005_oc.jpg)
In this section, the main roles that underlined the need to introduce nanogel functionalization are related to the need to increase cell selectivity, avoid macrophage uptake, reduce toxic effects and interact with biological barriers. In recent years researchers have discovered that surface decoration of nanogels can also have a deep impact on the physical and releasing properties of these nano-objects [Citation92,Citation93].
In particular, starting from the same nanogel system and changing the chemical groups that decorate their surface, it is possible to tune and so control and sustain drug delivery rates [Citation37]. Besides their use in drug encapsulation and release, functionalized nanogels showed promising applications also in the bioimaging field. Indeed the need to follow the fate of nanogels in biological systems underlined the need to be functionalized with specific tracking moieties [Citation94,Citation95]. The grafting should be stable under a wide range of biological environments and generally, pH stability is the most important. In this direction thiol-maleimide nanogels decorated with hydrophobic dye (BODIPY-SH) and N-(fluoresceinyl)maleimide, then functionalized with cyclic peptide, can improve cell uptake and bioimaging, thus building a high-performance theranostic device [Citation96]. Despite many different examples of functionalization with dyes and chromophores [Citation97,Citation98] one interesting alternative strategy is the use of quantum dots where the device takes advantage of their high imaging performances together with their reduced toxicity [Citation99]. Despite the good results already obtained in pre-clinical studies, further steps should be taken in order to finally reach the market. In particular considerable attention should be devoted to the different directions of production, final fate and medical performances. Considering the production issues, much attention should be given not only to easy scalable procedures but also to production reliability in order to have exact and homogeneous properties between different batches. In this direction process intensification that considers microfluidic approaches is extremely promising [Citation100]. Future studies should be also dedicated to the full understanding of the toxic effects of all the degradation products released by nanogels during their permanence within the body. Finally, a better amelioration of the performance in terms of selectivity and drug release rates should also be taken into consideration in order to optimize pharmaceutical treatment and reduce side effects maintaining the drug level within the desired range for a long period. It is well known that drugs not only physically entrapped, but linked to polymeric chains with cleavable bonds, are able to guarantee this performance.
However, structural modification of the active principles can represent a big issue in terms of pharmacokinetics and pharmacodynamics so future studies should be dedicated to non-covalent strategies able to ameliorate release rates without affecting the molecular structure of drugs.
Responsive nanogels: sensors
In the previous sections, we have already discussed extensively the features and unique characteristics of nanogels. Their biocompatibility, large surface area, stability and swelling behaviour have ensured them great recognition and employment in the biomedical and drug delivery field. Moreover, nanogels have emerged in recent years as smart materials with unique responsive features to an exogenous or internal trigger by changing their structural properties [Citation101]. Nanogels can present responsive ability to biological environments such as pH, bioreduction or biomolecule recognition, as well as to exogenous stimuli such as temperature and light. This characteristic can of course be modulated through proper synthesis methods and functionalization strategies and it has been applied, starting with the biomedical field, for imaging, targeted delivery and general employment as sensors [Citation102,Citation103]. An introduction scheme to a typology of stimuli-responsive nanogels is reported in the following .
Figure 6. Schematic representation of stimuli-responsive nanogels in response to enzyme, temperature, magnetic field and pH for drug delivery applications. Reprinted from Ref. [Citation104] with permission from Elsevier.
![Figure 6. Schematic representation of stimuli-responsive nanogels in response to enzyme, temperature, magnetic field and pH for drug delivery applications. Reprinted from Ref. [Citation104] with permission from Elsevier.](/cms/asset/07fca88b-77fb-48ff-9c47-5d8e8605e939/yimr_a_2026864_f0006_oc.jpg)
pH-responsive nanogels are one of the most widespread and employed types of this kind of system. They were employed initially in biomedical applications by making use of the pH gradients of the physiological systems, but now they are largely employed for multiple uses [Citation72]. This kind of system is generally obtained working with responsive polymers, which are able to maintain their properties even in the final framework of the system. A clear example of this has been provided by a ratio-metric fluorescent nanogel, obtained by a modified reprecipitation method, capable of sensing pH values in the physiological range [Citation105]. This nanogel was obtained from inert and biocompatible polyurethane polymer that was made pH-sensitive through functionalization with the bromothymol blue, a pH indicator. Furthermore, it was functionalized with two fluorophores (coumarin 6, C6 and Nile Red) in order to obtain the final fluorescent product.
The sensing mechanism of the whole system is based on the spectral overlap of the absorption of the pH indicator bromothymol blue with the dual emission of the fluorophores C6 and NR. The system presents a pH-dependent fluorescence resonance energy transfer (predominantly red fluorescence at pH 7) that takes place between two fluorophores in an aqueous suspension of nanogels, but not in aqueous solution alone where the two molecules are too far apart. This phenomenon can be explained on the basis of the spectra. Up photoexcitation of fluorophore C6 at 440 nm, in the system green fluorescence is induced and it presents a peak at 520 nm. In any case, a portion of the emission is transferred to Nile Red by the dependent fluorescence resonance energy transfer effect and because of this the resulting red fluorescence of Nile Red has a peak at 620 nm. In these conditions, the sensitivity to pH is obtained because bromothymol blue has a yellow colour at pH values of less than 6 with an absorption peak at 435 nm. On the other hand, at pH values above 8, bromothymol blue is blue, and it presents an absorption peak at 628 nm that overlaps the red emission of Nile Red. Because of this, in the fluorescence of the nanogels the green fluorescence of C6 prevails. The authors demonstrated the responsivity and sensitivity of these devices, thanks to their small size and high hydrophilicity.
Moreover, their easy fabrication method and tunability make it possible to synthesize similar sensing nanogels for specific ions by replacing the indicator dyes with appropriate ones [Citation106]. Another valuable example of pH-responsive nanogels has been provided by polysaccharide-based hybrid nanogels that integrate many features of smart nanogels including optical pH-sensing [Citation107]. In particular, the system was prepared by immobilization in situ of CdSe quantum dots inside a pH and temperature-responsive hydroxypropylcellulose-poly(acrylic acid) semi-interpenetrating polymer networks. The pH-sensitive poly(acrylic acid) network chains were designed to induce a pH-responsive volume phase transition. In fact, at pH < pKa of poly(acrylic acid), the dimensions of the nanogels remains constant due to the strong hydrogen bonding between the protonated poly(acrylic acid) chains and hydroxypropylcellulose chains.
On the other hand, when pH > pKa of poly(acrylic acid), the –COOH groups inside the poly(acrylic acid) chains gradually dissociate and weaken the hydrogen bond between the chains of hydroxypropylcellulose and poly(acrylic acid) inducing coulombic repulsion between the ionized –COO- groups, which result in a gradual increase in the size of the nanogels until the complete dissociation of all –COOH groups that takes place at pH > 6.5 resulting in maximum swelling for the system. This sensing ability can be largely useful and employed for controlled release regulated by the degree of swelling of nanogels. The employment of responsive polymers in the development of nanogels is a very valuable strategy for various purposes. For example, the same authors of the work just presented, reported the construction of hybrid nanogels by coating a Ag–Au bimetallic nanoparticle core with a thermo-responsive nonlinear poly(ethylene glycol)-based hydrogel as shell [Citation108]. This coating exhibits reversible thermo-responsive volume phase transition upon temperature changes that resulted in a significant influence on the size of the system. Moreover, this characteristic has been demonstrated to be influenced by the thickness of the layer and its sensitivity is in the range of 1–5°C. This approach is very interesting since it represents a clear example of the functionalization of a nanogel to obtain a final responsive device that can be employed for drug delivery and cell imaging.
As extensively discussed, pH and temperature-sensitive nanogels represent a very important family of nanodevices. Similarly, nanogels for ion detection are attracting growing interest because of their versatility and employability in various fields. The development of an innovative device for easy detection of traces of Pb2+ ions based on capsule membranes encapsulated with smart nanogels goes in this direction [Citation109]. The system was created with a semi-permeable calcium alginate membrane, with encapsulated poly(N-isopropylacrylamide-co-acryloylamidobenzo-18-crown-6) nanogels. The membrane allows Pb2+ ions and water to pass through but rejects the encapsulated nanogels. When the ions enter the capsule, they are recognized by nanogels because of the formation of 18-crown-6/Pb2+ ions that cause a Pb2+-induced phase transition of the nanogels from a hydrophobic to a hydrophilic state.
Because of this, the osmotic pressure inside the capsule membrane increases and then the elastic membrane swells upon the presence of Pb2+ ions in the environmental aqueous solution. The degree of swelling depends on the concentration of ions in the solution and because of this their quantity can be easily evaluated by directly measuring the Pb2+-induced isothermal swelling ratio of the capsule membrane. These swelling behaviours, reported in the following , can be easily observed with the naked eye and the detection limit for these ions has been demonstrated to be 10−9 mol/L.
Figure 7. Schematic representation of a capsule membrane system (A) for the detection of Pb2+ ions in water. This system consists of a semi-permeable membrane (I) and encapsulated nanogels (II) functionalized with crown aether units (III) for specific recognition of Pb2+ ions. In the system isothermal swelling of the capsule membrane (B) is induced due to the Pb2+ ions in solution increasing the osmotic pressure inside the system (IV). The nanogels encapsulated exhibit a Pb2+-induced isothermally hydrophobic to hydrophilics phase change (II to V) with the formation of host guest complexes (III to VI) leading to the increase of osmotic pressure inside the capsule. Because of this Pb2+ ions in water can be directly observed through the swelling of the capsule diameter. Reprinted from Ref. [Citation109] with permission from Elsevier.
![Figure 7. Schematic representation of a capsule membrane system (A) for the detection of Pb2+ ions in water. This system consists of a semi-permeable membrane (I) and encapsulated nanogels (II) functionalized with crown aether units (III) for specific recognition of Pb2+ ions. In the system isothermal swelling of the capsule membrane (B) is induced due to the Pb2+ ions in solution increasing the osmotic pressure inside the system (IV). The nanogels encapsulated exhibit a Pb2+-induced isothermally hydrophobic to hydrophilics phase change (II to V) with the formation of host guest complexes (III to VI) leading to the increase of osmotic pressure inside the capsule. Because of this Pb2+ ions in water can be directly observed through the swelling of the capsule diameter. Reprinted from Ref. [Citation109] with permission from Elsevier.](/cms/asset/8cf5204f-d57e-435d-a514-89c076216e3d/yimr_a_2026864_f0007_oc.jpg)
Nanogels can be properly functionalized even to guarantee the sensing of specific molecules or compounds. A clear example of this is represented by glucose sensing [Citation110] where a hybrid nanogel, made of small Ag nanoparticles coated with poly(4-vinylphenylboronic acid-co-2-(dimethylamino)ethyl acrylate) (named p(VPBA-DMAEA)) gel shell, used a boronate derivative employed as glucose recognition element. In fact, this p(VPBA-DMAEA) shell undergoes a volume phase transition after glucose concentration variations and shows great sensitivity (0–30 mM) at physiological pH and temperature. This feature is exploited to modify the fluorescence intensity of nanogels obtaining a final optical glucose sensor. Similarly, a polyacrylamide-based nanogel sensor was developed for spectral and colorimetric measurement of ionizing radiation doses [Citation111]. The system was created working with coumarin-3-carboxylic acid, as an IR-sensitive fluorescent probe, and with 5(6)-carboxytetramethylrhodamine as an IR inert probe. When exposed to IR the coumarin-3-carboxylic acid molecule moieties react with hydroxyl radicals obtained from water radiolysis and the transition to 7-hydroxyl-coumarin-3-carboxylic acid emits a strong blue fluoresnce (λem = 450 nm). due to π-π* transitions under UV excitation (λex = 400 nm). In contrast, 5(6)-carboxytetramethylrhodamine emits stable red fluorescence (λem = 580 nm) under UV irradiation (λex = 530 nm). Coupled with fluorescent probes, the nanogels also exhibit colorimetric fluorescence as a function of varying IR doses. The synthetic route of those devices, together with their working principle is reported in the following .
Figure 8. (A) Synthetic route for polyacrylamide-based nanogels and their working principle for measurement of ionizing radiation doses (B) Fluorescence emission spectra (λex1 = 400 nm, λex2 = 530 nm) (C) photographs of the X-ray-irradiated nanogels sensor under UV excitation (λex = 365 nm) with their dose range 0–20 Gy. APMA: N-(3-aminopropyl)methacrylamide; TAMRA: 5(6)-carboxytetramethylrhodamine; PAAT: poly(AAm-co-APMA-co-TAMRA); AAm: Acrylamide; BIS: N,N′-methylene – bisacrylamide; PAATC: poly-(AAm-co-APMA-co-TAMRA)/CCA. Reprinted from Ref. [Citation111] with permission from Royal Society of Chemistry.
![Figure 8. (A) Synthetic route for polyacrylamide-based nanogels and their working principle for measurement of ionizing radiation doses (B) Fluorescence emission spectra (λex1 = 400 nm, λex2 = 530 nm) (C) photographs of the X-ray-irradiated nanogels sensor under UV excitation (λex = 365 nm) with their dose range 0–20 Gy. APMA: N-(3-aminopropyl)methacrylamide; TAMRA: 5(6)-carboxytetramethylrhodamine; PAAT: poly(AAm-co-APMA-co-TAMRA); AAm: Acrylamide; BIS: N,N′-methylene – bisacrylamide; PAATC: poly-(AAm-co-APMA-co-TAMRA)/CCA. Reprinted from Ref. [Citation111] with permission from Royal Society of Chemistry.](/cms/asset/ea10bf0b-a198-4bee-8278-c9391e064136/yimr_a_2026864_f0008_oc.jpg)
In this section, various responsive nanogels employable as sensors have been presented and different strategies can be employed, involving responsive polymer either as core material or as functionalization chains [Citation112]. Moreover, the combination of these biomaterials with the proper membrane or designed layer can guarantee high sensitivity and employability in different applications. All these works testify to the importance of responsive nanogels not only as controlled drug delivery systems but also as sensing materials for different applications. Future studies in this field are necessary not only to guarantee easy scalable and reliable production, as in the case of drug delivery systems, but also the possibility of reuse. Therefore a great deal of attention should be devoted not only to selective adsorption but also to desorption and ability to be used again.
Nanogels in catalysis
The design and synthesis of innovative catalytic systems, capable of overcoming some of the limitations of conventional catalysts, is an attractive challenge for many researchers regarding various field of applications such as biocatalysts [Citation113,Citation114]. In fact, great attention is now being given to the possibility of employing biological living systems, such as enzymes, to speed up chemical reactions with high efficiency, selectivity and low environmental impact. A crucial point for this kind of application is the incorporation of the enzymes in a support or carrier that can guarantee the catalytic activity of the system [Citation115,Citation116]. Nanostructured materials and nanogels, have come to the fore in recent years as a valuable tool for the incorporation of enzymes thanks to their exceptional surface area, great stability under various conditions and the marginal increase in mass-transfer resistances [Citation117,Citation118]. A clear example of the potential of nanogels in this kind of application is represented by the encapsulation of lipase into an interpenetrating polymer matrix made of polyacrylamide and poly(N-isopropylacrylamide) nanogel [Citation16].
The system was synthesized to obtain enhanced stability and activity in both polar and non-polar organic solvents and a three-step method, using acryloylation, polymerization with acrylamide and sequential polymerization with N-isopropyl acrylamide, was employed to obtain a temperature-sensitive interpenetrating polymer network. This matrix was demonstrated to form a more hydrophobic environment, compared to polyacrylamide alone because of the penetration of N-isopropyl acrylamide into the network through hydrogen bonding. This feature is essential for the uptake of hydrophobic substrates to enhance the rate of enzymatic catalysis. The efficacy of the synthesized device was confirmed through the hydrolysis reaction of p-nitrophenylpalmitate in an aqueous environment and the esterification reaction of ibuprofen in isooctane. The residual activity of lipase nanogel maintains a value of 70% at 60°C for 4 h, much more than the free lipase in the same condition. Moreover, the possibility of reusing the system for more cycles without losses in activity has been confirmed and even the yield of the reaction considered (33%) was significantly increased compared to the reaction using only free lipase (22%). Because of all mentioned features, this kind of system has been recognized as a valuable enzymatic catalytic system in non-polar solvents. Another valuable example of this kind of system [Citation119], commonly identified as single-enzyme nanoparticles, is represented by the preparation of phenoxazinone synthase nanogels, obtained through in situ aqueous polymerization. Nanogels were synthesized working with N-acryloxysuccinimide in a phenoxazinone solution and then, after purification with dialysis, acrylamide, ammonium persulphate and tetramethyl ethylenediamine were added to the system, under N2 purging, to promote the polymerization. The nanogels were finally recovered with dialysis and lyophilization and characterized through dynamic light scattering analysis, confirming their formation. The enzyme was demonstrated to successfully retain more than 80% of its initial activity and to exhibit the most activity at pH 4.0–4.5 and 50°C. In addition, phenoxazinone nanogels showed great thermal and solvent resistance and because of this, they represent a promising biocatalyst in the industry. In recent years, this working strategy regarding the encapsulation of individual enzymes inside thin hydrogel has been widely studied.
Beloqui and co-workers succeeded in developing a simple one-step synthesis for the fabrication of single enzyme nanogels [Citation120]. They showed how the presence of additives, such as sucrose, during the polymerization makes it possible to prepare stable single-enzyme nanogels avoiding the modification of the enzyme to introduce polymerizable groups. Another important strategy regarding the employment of nanogels in catalysis is represented by the use of core–shell nanogels as stabilizers and supports for catalytically active metallic nanoparticles [Citation121]. In fact, in recent years the possibility of employing nanostructured polymeric architectures as metal supports for applications in catalysis has been widely studied. The most important advantage of this innovative approach is the possibility of developing the proper design for the polymeric support and tuning their responsive behaviour. A clear example of this is represented by nanogels obtained using RAFT radical polymerization with a stabilizing shell of poly(N,N-dimethylacrylamide) and a N,N-dimethylaminoethyl acrylate core and employed to provide the amines required for Pd coordination [Citation73]. The Pd0NPs were embedded in the nanogels by the addition of a Pd2 salt to be coordinated by the nitrogen functions of the N,N-dimethylaminoethyl acrylate units, followed by metal reduction with ethanol. Then a solution of the nanogels and palladium acetate in dichloromethane was stirred for 24 h to promote the diffusion of the metal salt into the nanogel. Dynamic light scattering confirmed the integrity of these nano systems, and the potential of these devices in catalysis was investigated through their application as catalysts for the Mizoroki-Heck reaction between n-butyl acrylate and a series of bromo- and iodoarenes. The scheme of this process is reported in the following , the yields were very good with values over 90% and catalyst recycling was demonstrated to be feasible up to three times without loss inactivity.
Figure 9. (A) Schematic representation of embedding of Pd0 nanoparticles in nanogels and (B) the use of this device as catalyst, together with its lifecycle, for the Mizoroki-Heck reaction in organic solvents. Reprinted from Ref. [Citation73] with permission from Wiley.
![Figure 9. (A) Schematic representation of embedding of Pd0 nanoparticles in nanogels and (B) the use of this device as catalyst, together with its lifecycle, for the Mizoroki-Heck reaction in organic solvents. Reprinted from Ref. [Citation73] with permission from Wiley.](/cms/asset/bccf57bd-5139-4b43-a6cc-3c62d5988179/yimr_a_2026864_f0009_oc.jpg)
A similar working strategy for the development of gold nanoparticles inside a chitosan-poly(methacrylic acid) polymeric network has been employed [Citation122]. They proposed this synthesis starting from the polymerization of methacrylic acid in chitosan solution with chloroauric acid, N, N′-methylenebisacrylamide cross-linker and ammonium persulfate initiator. This device exhibits great electrocatalytic activities towards 5-fluorouracil, because of the strong H-bonding interaction between the –OH and –COOH groups of chitosan and poly(methacrylic acid) with this kind of molecule.
Another example of this employment of a nanogel-based system as an electrocatalyst is provided by the formation of a self-assembled macroion nanogel-based complex using sustainable cellulose nanocrystals and raw cloisite-Na+ with platinum nanoparticles [Citation123]. The encapsulation of the metal was realized working with dried gels dispersed in water and adding in the system PtCl2 with HCl and with the subsequent addition of sodium borohydride to promote the reduction of PtCl2 to Pt. The physicochemical characterization of nanogels confirmed the formation of the particles and these systems showed good activity towards methanol oxidation in an alkaline medium.
Nanogels can be successfully employed in the field of catalysis even as support materials for other molecules. An example of this is represented by the L-Proline functionalized poly(methyl methacrylate) nanogels prepared via emulsion polymerization [Citation63]. They demonstrated the influence on the catalytic efficiency of the cross-linking density and the degree of catalyst functionalization. The aldol reaction of 4-nitrobenzaldehyde and cyclohexanone was used as model reaction with the functionalized nanogel at 1 mol% catalyst loading, and it was found that after 24 h the reaction catalyzed by nanogels with high degree of catalyst functionalization is less efficient than those with low degree of catalyst functionalization. Moreover, nanogels characterized by the crosslinking density (CD) in the range 0.5–10 wt% were found to have the higher conversions and the changes in this range of CD were demonstrated to have low effect on the catalyst activity. Similarly, nanogels can be properly synthesized and functionalized to simulate artificial enzymes for efficient and controllable catalysis. In this direction poly(N-isopropyl acrylamide) matrix is able to provide a temperature-responsive and size-controllable scaffold, with 1-vinyl imidazole moieties stabilizing the enzymatic centres of the system through coordination interaction with hemin [Citation124]. The final system was quite stable and its catalytic activity was maintained in a broad range of heat and pH. These devices were active for catalytic oxidation for various azo compounds and their activity was efficiently tuned by temperature variations.
As widely discussed in this section, nanogels are acquiring great importance in the field of catalysis through various employments thanks to their tuneable features, stability and low mass-transfer resistance [Citation27]. They can be used as an encapsulant for enzymes, guaranteeing their activity and selectivity, but also as a support for metallic nanoparticles promoting their catalytic efficiency. Finally, the possibility of using properly formulated and functionalized nanogels as a mimic for natural enzymes or catalysts is gaining ground as an environmentally friendly strategy to catalyze chemical reactions. In this field future studies should be devoted not only to physical stability, thus avoiding aggregation with consequent decrease of surface area (key property in catalysis) in the presence of reactants. Moreover, their ability to be inert and their easy recovery from the reacting mixture should also be guaranteed. Several strategies such as the use of external fields, such as magnetic fields, were found to be promising in lab scale and so their capability at the large scale should be tested. Moreover, as for the other applications, reliable production is also fundamental in order to have properties and performance that do not change from batch to batch.
Environmental applications of nanogels
Focus on the environment and its care are essential themes for society today and in this field all chemical-related science can do a great job [Citation125]. Among them, a growing interest in innovative soft and smart devices that can be employed in filtration and the removal of contaminants has been reported in the last decade [Citation126,Citation127]. Natural polymer-based materials are emerging as potential adsorbents for different kinds of molecules and pollutants thanks to their non-toxicity, biodegradability, high affinity for binding with a wide variety of compounds and low costs [Citation128–130]. In this context, nanogels came out as valuable adsorbents thanks to their large surface area to volume ratio, mechanical stability, ability to swell and hold substantial volumes of liquids. In this context, both the possibility of adsorbing dyes and heavy metal ions or similar compounds have been extensively investigated and their mechanism of action has been schematized in .
Figure 10. Schematization of nanogel synthesis (a) for dye removal together with their action (b) in a contaminated water solution. The presence of hydrophobic sites in the nanogel formulation is extremely important for the adsorption of a wide variety of pollutants.
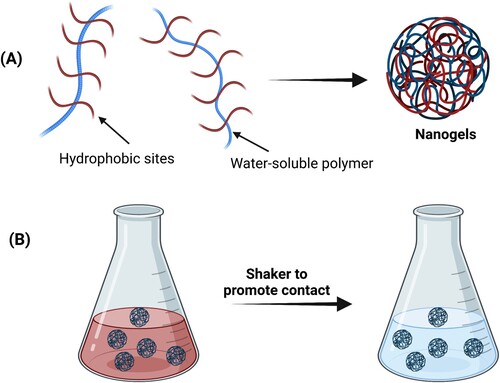
A clear example of the potential of nanogels even in this kind of application is provided by poly vinyl phosphonic acid nanogels where the monomer is used in a process of microemulsion polymerization with cetyl triethylammonium bromide as cationic surfactant [Citation131]. The inclusion of silica-coated Fe3O4 nanoparticles in the system during polymerization makes it possible to render the system responsive to a magnetic field. These materials were tested as adsorbents for the removal of 4-nitrophenol, 1-1′-Dimethyl-4,4′-bipyridinium dichloride, methylene blue and rhodamine 6G from aqueous media. The contaminated water was treated with nanogels for only five minutes and the absorbed amounts of compounds were estimated using a UV-Vis spectrometer. The results obtained were very interesting, demonstrating how the nanogel particles were able to absorb more than 10 milligrams per gram of particles of each analyzed compound in a very short time.
These results confirm the efficacy of these materials as fast absorbents, which is very often a key factor for the environmental applications of those systems (e.g. rapid removal process for a water stream), together with magnetic properties which can very often be useful for recovering the absorbent systems. Another important work on this topic has been reported using a novel composite nanogel of gellan and pullulan as absorbent material for methylene blue [Citation132]. The system was synthesized by mixing two solutions of gellan (0.5%) and pullulan (2%) and, after stirring, adding 2,2-diphenyl-1-picrylhydrazyl to the system promoting chemical crosslinking. The final product was recovered by centrifugation, filtration and concentration under vacuum and its adsorption capacity was evaluated by fitting various isotherm models to understand the thermodynamics and the kinetics of the process. Various formulations of nanogels were tested, using only gellan, only pullulan and a proper combination of both. In each case, all the isotherms were conformed to the type-L isotherm which confirms that the adsorbate has a high affinity for adsorption on the synthetized nanogels. The authors explained this behaviour considering the electrostatic attraction between the cationic dye and negative charge of the adsorbent material. Different solutions can be employed in order to adsorb commercial dyes from aqueous solutions, employing different polymers, working conditions and synthesis procedures [Citation20,Citation133]. In this context, great attention is now being given even to the employment of natural polymers for the synthesis of nanogels to obtain biocompatible cost effective systems as potential adsorbents for water contaminants [Citation133]. An example of this kind of device is the microcrystalline cellulose-based nanogel grafted with acrylamide and acrylic acid in the presence of a crosslinking agent (methylene bisacrylamide) and initiator (potassium persulphate) [Citation30]. These devices were applied to remove both dyes (reactive red 195) and cadmium ions (Cd(II)) from an aqueous medium and they performed well. Different operational conditions were employed and it was demonstrated how the feed concentration of monomers has a significant effect on the removal efficacy of reactive red 195 which showed the best performance with 10 min of contact time and at pH 2, with a dose of 1.5 g/L.
On the other hand, efficacy in the removal of Cd(II) was not influenced by the feed concentration of monomers and the best performance was obtained at pH 6 and a dose of 0.5 g/L. The adsorption equilibrium data were described using the Freundlich model for reactive red 195 and the Languimir model for cadmium (II). Another valuable solution in the field of water treatment using absorbent nanogels is represented by core–shell nanogels which are very often able to guarantee absorbent properties both for dyes and for heavy metal pollutants. A valuable example of this kind of strategy is represented by the development of core–shell smart ionic nanogels working with poly(vinyl alcohol) core and poly(N-isopropyl acrylamide/acrylic acid) shell particles using a one-step surfactant-free emulsion polymerization [Citation134]. The performance of these devices as Copper (II) removal systems have been evaluated considering various parameters such as pH, contact time, temperature and so on. As expected, the nanogel performance improved at the longer contact time, and its adsorbent properties decreased with higher temperature and lower pH because of the responsive characteristic of the constituent polymeric chains. The Cu2+ sorption process was successfully described with the Freundlich adsorption model and the maximum sorption capacity was estimated at 94 mg/g, indicating this material as an effective and practical polymeric adsorbent. The possibility of using the same system to remove dyes and heavy metals is of clear utility and is attracting growing interest for possible multiple and combined applications. Another example of nanogels with these features considered the preparation of super-amphiphilic silica-nanogels composites to reduce the contact angle of water and increase the diffusion of pollutants into the adsorbent materials [Citation135]. These devices were synthesized by a dispersion polymerization technique encapsulating silica nanoparticles inside polyacrylamides nanogels guaranteeing special wettability of the final system. The presence of sulphonate groups in the chemical structure of nanogels guarantees high surface activity and wetting characteristics at the interfaces and these kinds of composites were successfully employed to remove both heavy metals and dyes from wastewater.
During the tests with this kind of devices the sorption equilibria were reached rapidly (10–25 min) and the adsorption process of dyes (e.g. methylene blue) was successfully described by the Langmuir model, achieving a maximum adsorption capacity of over 400 mg/g, which is considerably high. As presented in this section, nanogels have now become extremely versatile and employable even in adsorbent applications. Their potential is confirmed by their employment in decontamination processes where the use of smart sustainable systems for different molecules and compounds is frequently required. In another work, multifunctional magnetic nanogels were prepared by in-situ co-precipitation method in the presence of branched polyethyleneimine (bPEI) nanogels for use in dye adsorbent and catalyst support (A). It was reported that the Fe3O4/bPEI magnetic nanogel employed as efficient and selective adsorbents toward Congo red dye ( B-F). In addition, Fe3O4/bPEI magnetic nanogel was also shown to be excellent catalyst supports for Pd nanoparticles in the reduction of nitrophenols [Citation136]. In order to arrive at industrial application future studies should be devoted not only to easy, scalable and reliable production, as on the other applications together with the absence of aggregation in the working environment, but also to the toxicity of nanogels and of their degradation products in the environment. Furthermore, much consideration should be given to the ability to be reused for many cycles in order to guarantee on one hand the recovery of their content and on the other hand their landfill disposal that would negatively affect their environmental impact. Concluding this overview on the applications of nanogels, we report here in the general characteristics and main applications of each type of nanogels described.
Figure 11. (A) Schematic illustration of the preparation of Fe3O4/bPEI and Pd-Fe3O4/bPEI nanocomposites, digital photos of dye solution before and after absorptions by the Fe3O4/bPEI nanocomposite, (B) 80 mg/L of CR, (C) 80 mg/L of MB, and (D) the mixture of CR (53.3 mg/L) and MB (26.7 mg/L), (E) adsorption curves of Fe3O4/bPEI nanocomposites toward CR and MB in neutral medium (pH 7). (F) adsorption curves of Fe3O4/bPEI nanocomposite toward CR and MB in different mediums. Reprinted from Ref. [136] with permission from Springer.
![Figure 11. (A) Schematic illustration of the preparation of Fe3O4/bPEI and Pd-Fe3O4/bPEI nanocomposites, digital photos of dye solution before and after absorptions by the Fe3O4/bPEI nanocomposite, (B) 80 mg/L of CR, (C) 80 mg/L of MB, and (D) the mixture of CR (53.3 mg/L) and MB (26.7 mg/L), (E) adsorption curves of Fe3O4/bPEI nanocomposites toward CR and MB in neutral medium (pH 7). (F) adsorption curves of Fe3O4/bPEI nanocomposite toward CR and MB in different mediums. Reprinted from Ref. [136] with permission from Springer.](/cms/asset/a9a34fb2-40e2-4ece-a98d-9386064600e6/yimr_a_2026864_f0011_oc.jpg)
Table 2. Characteristics and general applications of different types of nanogels.
Challenges and future perspectives
Polymer-based nano-networks were found to be extremely promising in many different fields, from medicine to catalysis and environmental applications. The advantages that make them extremely promising derive from their C-based chemistry, ability to swell or deswell in response to external stimuli together with biocompatibility and high surface area. However, generally, the easy combination of natural or synthetic macromolecules that can build nano-objects is not enough to guarantee proper performance.
For this reason, their functionalization is fundamental to obtain colloid particles that can work as smart drug delivery systems, sensors, catalysts or adsorbents as summarized in .
Table 3. Overview on the main nanogel applications presented in the present work, together with their characteristics and advantages compared to conventional systems employed in those uses.
Even if very good results were obtained, in recent years some challenges have remained unsolved and this represents the real obstacle to their use in common practice.
Starting from basic sciences, a better material understanding on how these nano-systems behave in the presence of complex biological fluids, together with the mechanisms behind cell uptake, is pivotal not only for biomedical applications but also in other fields. In particular, the role of functionalization can ameliorate the level of knowledge and distinguish the properties related to the core (nanogel) from the shell (external functionalization). In parallel, safety studies must be conducted well to avoid any kind of risk. More thorough investigations in nanotoxicology should be conducted to understand the possible negative effects of nanogel administration in the human body and in particular their accumulation and elimination together with long-term effects. The factors that can influence toxicity should be identified considering that nanogels can be ingested, inhaled and/or adsorbed through the skin. The easy penetration of physiobiological barriers that is generally an advantage that makes these devices extremely promising can represent a serious drawback. Furthermore, potential toxic effects were studied, also considering the environmental point of view, using life cycle assessment (LCA), taking account of the possible accumulation that can cause pollution in water for example and then contaminate again biological systems which raises nanotoxicology issues once more. Life cycle assessment would also study the entire manufacturing process, something that represents a big challenge and not only from LCA point of view. Indeed it is well known that scaling up the production of nano-systems compared to bulk systems is extremely difficult due to the fact that surface properties are favoured only at the small scale, generally lab scale. Furthermore, the presence of surface functionalization, fundamental as said for their performance, complicates the entire manufacturing process and the quality assays of the final products. In this direction, in recent years many studies have been conducted not only in scaling up but mostly in scaling down and microfluidic processes that now represent the most promising approach. Particular attention should be also devoted to proper nanogel formation at low concentration, stability and reliability of the functionalization steps together with the right conditions to delay phenomena of flocculation and aggregation that can reduce the lifetime of the colloids.
Lastly, economic and financial barriers are important and can be an impediment in nanogel use, especially in fields other than medicine. Indeed the use of functionalized nanogels as catalysts or adsorbent materials can be economically convenient only if we focus our attention not only on their use but mostly on their recovery and reuse many times without losing their initial performances.
Disclosure statement
No potential conflict of interest was reported by the authors.
Additional information
Funding
References
- Jeevanandam J, Barhoum A, Chan YS, et al. Review on nanoparticles and nanostructured materials: history, sources, toxicity and regulations. Beilstein J Nanotechnol. 2018;9:1050–1074. doi:10.3762/bjnano.9.98.
- Sharifi M, Attar F, Saboury AA, et al. Plasmonic gold nanoparticles: optical manipulation, imaging, drug delivery and therapy. J Control Release. 2019;311–312:170–189. doi:10.1016/j.jconrel.2019.08.032.
- Moshirpanahi A, Etemadi Haghighi S, Imam A. Dynamic modeling of a cylindrical nanoparticle manipulation by AFM. Eng Sci Technol Int J. 2021;24:611–619. doi:10.1016/j.jestch.2020.12.013.
- Nasrollahzadeh M, Sajadi SM, Sajjadi M, et al. Chapter 4 – applications of nanotechnology in daily life. In: M Nasrollahzadeh, SM Sajadi, M Sajjadi, Z Issaabadi, M Atarod, editors. An introd. to Green nanotechnol. City: Elsevier; 2019. p. 113–143. https://doi.org/10.1016/B978-0-12-813586-0.00004-3.
- Downey ML, Moore DT, Bachula GR, et al. National nanotechnology initiative – leading to the next industrial revolution. Microscale Thermophysic Eng.. 2000;4:205–212. doi:10.1080/10893950050148160.
- Zhang Q, Chen X, Geng S, et al. Nanogel-based scaffolds fabricated for bone regeneration with mesoporous bioactive glass and strontium: in vitro and in vivo characterization. J Biomed Mater Res – Part A. 2017;105:1175–1183. doi:10.1002/jbm.a.35980.
- Seok HY, Sanoj Rejinold N, Lekshmi KM, et al. CD44 targeting biocompatible and biodegradable hyaluronic acid cross-linked zein nanogels for curcumin delivery to cancer cells: In vitro and in vivo evaluation. J Control Release. 2018;280:20–30. doi:10.1016/j.jconrel.2018.04.050.
- Laha B, Das S, Maiti S, et al. Novel propyl karaya gum nanogels for bosentan: In vitro and in vivo drug delivery performance. Colloids Surf B Biointerfaces. 2019;180:263–272. doi:10.1016/j.colsurfb.2019.04.064.
- Karg M, Pich A, Hellweg T, et al. Nanogels and microgels: from model colloids to applications, recent developments, and future trends. Langmuir. 2019;35:6231–6255. doi:10.1021/acs.langmuir.8b04304.
- El-Naggar ME, Radwan EK, El-Wakeel ST, et al. Synthesis, characterization and adsorption properties of microcrystalline cellulose based nanogel for dyes and heavy metals removal. Int J Biol Macromol. 2018;113:248–258. doi:10.1016/j.ijbiomac.2018.02.126.
- Jiang L, Li W, Nie J, et al. Fluorescent nanogel sensors for X-ray dosimetry. ACS Sensors. 2021;6:1643–1648. doi:10.1021/acssensors.1c00204.
- Wei P, Gangapurwala G, Pretzel D, et al. Smart pH-sensitive nanogels for controlled release in an acidic environment. Biomacromolecules. 2019;20:130–140. doi:10.1021/acs.biomac.8b01228.
- Zhang Y, Zhang D, Wang J-T, et al. Fabrication of stimuli-responsive nanogels for protein encapsulation and traceless release without introducing organic solvents{,} surfactants{,} or small-molecule cross-linkers. Polym Chem. 2021;12:554–563. doi:10.1039/D0PY01600D.
- Shi H, Liu Y, Qu R, et al. A facile one-pot method to prepare peroxidase-like nanogel artificial enzymes for highly efficient and controllable catalysis. Colloids Surf B Biointerfaces. 2019;174:352–359. doi:10.1016/j.colsurfb.2018.11.021.
- Zou B, Yan Y, Xia J, et al. Enhancing bio-catalytic activity and stability of lipase nanogel by functional ionic liquids modification. Colloids Surf B Biointerfaces. 2020;195:111275, doi:10.1016/j.colsurfb.2020.111275.
- Du M, Lu D, Liu Z. Design and synthesis of lipase nanogel with interpenetrating polymer networks for enhanced catalysis: molecular simulation and experimental validation. J Mol Catal B Enzym. 2013;88:60–68. doi:10.1016/j.molcatb.2012.11.017.
- Bin Hamzah Y, Hashim S, Rahman WAWA. Synthesis of polymeric nano/microgels: a review. J Polym Res. 2017;24:134. doi:10.1007/s10965-017-1281-9.
- Pinelli F, Perale G, Rossi F. Coating and functionalization strategies for nanogels and nanoparticles for selective drug delivery. Gels. 2020;6; doi:10.3390/gels6010006.
- Lu K. Hybrid materials – a review on co-dispersion, processing, patterning, and properties. Int Mater Rev. 2020;65:463–501. doi:10.1080/09506608.2019.1653569.
- Shoueir KR, Sarhan AA, Atta AM, et al. Macrogel and nanogel networks based on crosslinked poly (vinyl alcohol) for adsorption of methylene blue from aqua system. Environ Nanotechnol Monit Manag. 2016;5:62–73. doi:10.1016/j.enmm.2016.03.001.
- Shah MT, Alveroglu E. Facile synthesis of nanogels modified Fe3O4@Ag NPs for the efficient adsorption of bovine & human serum albumin. Mater Sci Eng C. 2021;118:111390. doi:10.1016/j.msec.2020.111390.
- Yiamsawas D, Kangwansupamonkon W, Kiatkamjornwong S. Lignin-based nanogels for the release of payloads in alkaline conditions. Eur Polym J. 2021;145:110241. doi:10.1016/j.eurpolymj.2020.110241.
- Anandharamakrishnan C. 3.31 – Trends and impact of Nanotechnology in agro-Food sector. In: K Knoerzer, K Muthukumarappan, editors. Innov. food process. technol. Oxford: Elsevier; 2021. p. 523–531. https://doi.org/10.1016/B978-0-12-815781-7.00005-6.
- Vinogradov S, Batrakova E, Kabanov A. Poly(ethylene glycol)-polyethyleneimine NanoGel(TM) particles: novel drug delivery systems for antisense oligonucleotides. Colloids Surf B Biointerfaces. 1999;16:291–304. doi:10.1016/S0927-7765(99)00080-6.
- Kabanov AV, Vinogradov SV. Nanogels as pharmaceutical carriers: finite networks of infinite capabilities. Angew Chemie – Int. Ed. 2009;48:5418–5429. doi:10.1002/anie.200900441.
- Pinelli F, Ortolà ÓF, Makvandi P, et al. In vivo drug delivery applications of nanogels: a review. Nanomedicine (Lond). 2020;15:2707–2727. doi:10.2217/nnm-2020-0274.
- Mauri E, Giannitelli SM, Trombetta M, et al. Synthesis of nanogels: current trends and future outlook. Gels. 2021;7:1–23. doi:10.3390/gels7020036.
- Zhang H, Zhai Y, Wang J, et al. New progress and prospects: the application of nanogel in drug delivery. Mater Sci Eng C. 2016;60:560–568. doi:10.1016/j.msec.2015.11.041.
- Yin Y, Hu B, Yuan X, et al. Nanogel: a versatile nano-delivery system for biomedical applications. Pharmaceutics. 2018;12. doi:10.20959/wjpps20189-12364.
- El-Naggar ME, Radwan EK, El-Wakeel ST, et al. Synthesis, characterization and adsorption properties of microcrystalline cellulose based nanogel for dyes and heavy metals removal. Int J Biol Macromol. 2018;113:248–258. doi:10.1016/j.ijbiomac.2018.02.126.
- Sultana F, Manirujjaman I-U-H, Arafat M, et al. An overview of nanogel drug delivery system. J Appl Pharm Sci. 2013;3:95–105. doi:10.7324/JAPS.2013.38.S15.
- Sharma A, Garg T, Aman A, et al. Nanogel – an advanced drug delivery tool: current and future. Artif Cells Nanomed Biotechnol. 2016;44:165–177. doi:10.3109/21691401.2014.930745.
- Qureshi MA, Khatoon F. Different types of smart nanogel for targeted delivery. J Sci Adv Mater Devices. 2019;4:201–212. doi:10.1016/j.jsamd.2019.04.004.
- Shah S, Rangaraj N, Laxmikeshav K, et al. Nanogels as drug carriers – introduction, chemical aspects, release mechanisms and potential applications. Int J Pharm. 2020;581:119268. doi:10.1016/j.ijpharm.2020.119268.
- Mauri E, Chincarini GMF, Rigamonti R, et al. Modulation of electrostatic interactions to improve controlled drug delivery from nanogels. Mater Sci Eng C. 2017;72:308–315. doi:10.1016/j.msec.2016.11.081.
- Pinelli F, Pizzetti F, Fullana Ó, et al. Influence of the core formulation on features and drug delivery ability of carbamate-based nanogels. Int J Mol Sci. 2020;21.
- Mauri E, Cappella F, Masi M, et al. PEGylation influences drug delivery from nanogels. J Drug Deliv Sci Technol. 2018;46:87–92. doi:10.1016/j.jddst.2018.05.003.
- Wang J, Xu W, Zhang N, et al. X-ray-responsive polypeptide nanogel for concurrent chemoradiotherapy. J Control Release. 2021;332:1–9. doi:10.1016/j.jconrel.2021.02.003.
- Hussain MA, Abbas K, Jantan I, et al. Polysaccharide-based materials in macromolecular prodrug design and development. Int Mater Rev. 2017;62:78–98. doi:10.1080/09506608.2016.1209617.
- Morimoto N, Endo T, Ohtomi M, et al. Hybrid nanogels with physical and chemical cross-linking structures as nanocarriers. Macromol Biosci. 2005;5:710–716. doi:10.1002/mabi.200500051.
- Chacko RT, Ventura J, Zhuang J, et al. Polymer nanogels: a versatile nanoscopic drug delivery platform. Adv Drug Deliv Rev. 2012;64:836–851. doi:10.1016/j.addr.2012.02.002.
- Ekkelenkamp AE, Elzes MR, Engbersen JFJ, et al. Responsive crosslinked polymer nanogels for imaging and therapeutics delivery. J Mater Chem B. 2018;6:210–235. doi:10.1039/C7TB02239E.
- Kazakov S. Liposome-nanogel structures for future pharmaceutical applications: an updated review. Curr Pharm Des. 2016;22:1391–1413. doi:10.2174/1381612822666160125114733.
- Chen D, Yu H, Sun K, et al. Dual thermoresponsive and pH-responsive self-assembled micellar nanogel for anticancer drug delivery. Drug Deliv. 2014;21:258–264. doi:10.3109/10717544.2013.838717.
- Zhang X, Malhotra S, Molina M, et al. Micro- and nanogels with labile crosslinks – from synthesis to biomedical applications. Chem Soc Rev. 2015;44:1948–1973. doi:10.1039/C4CS00341A.
- Sanson N, Rieger J. Synthesis of nanogels/microgels by conventional and controlled radical crosslinking copolymerization. Polym Chem. 2010;1:965–977. doi:10.1039/c0py00010 h.
- Shah S, Rangaraj N, Laxmikeshav K, et al. Nanogels as drug carriers – introduction, chemical aspects, release mechanisms and potential applications. Int J Pharm. 2020;581:119268. doi:10.1016/j.ijpharm.2020.119268.
- Hajebi S, Abdollahi A, Roghani-Mamaqani H, et al. Hybrid and hollow poly(N,N-dimethylaminoethyl methacrylate) nanogels as stimuli-responsive carriers for controlled release of doxorubicin. Polymer (Guildf). 2019;180:121716. doi:10.1016/j.polymer.2019.121716.
- Richtering W, Potemkin II, Rudov AA, et al. Could multiresponsive hollow shell–shell nanocontainers offer an improved strategy for drug delivery? Nanomedicine. 2016;11:2879–2883. doi:10.2217/nnm-2016-0327.
- Schmid AJ, Dubbert J, Rudov AA, et al. Multi-shell hollow nanogels with responsive shell permeability. Sci Rep. 2016;6:1–13. doi:10.1038/srep22736.
- Dubbert J, Honold T, Pedersen JS, et al. How hollow are thermoresponsive hollow nanogels? Macromolecules. 2014;47:8700–8708. doi:10.1021/ma502056y.
- Zhang J, Jia J, Kim JP, et al. Construction of versatile multilayered composite nanoparticles from a customized nanogel template. Bioact Mater. 2018;3:87–96. doi:10.1016/j.bioactmat.2017.06.003.
- Zavgorodnya O, Carmona-Moran CA, Kozlovskaya V, et al. Temperature-responsive nanogel multilayers of poly(N-vinylcaprolactam) for topical drug delivery. J Colloid Interface Sci. 2017;506:589–602. doi:10.1016/j.jcis.2017.07.084.
- Park CW, Yang H-M, Lee HJ, et al. Core–shell nanogel of PEG–poly(aspartic acid) and its pH-responsive release of rh-insulin. Soft Matter. 2013;9:1781–1788. doi:10.1039/C2SM26865E.
- Sahiner N, Alb AM, Graves R, et al. Core-shell nanohydrogel structures as tunable delivery systems. Polymer (Guildf). 2007;48:704–711. doi:10.1016/j.polymer.2006.12.014.
- Pinelli F, Sacchetti A, Perale G, et al. Is nanoparticle functionalization a versatile approach to meet the challenges of drug and gene delivery? Ther Deliv. 2020;11; doi:10.4155/tde-2020-0030.
- Stanislawska I, Liwinska W, Lyp M, et al. Recent advances in degradable hybrids of biomolecules and NGs for targeted delivery. Molecules. 2019;24; doi:10.3390/molecules24101873.
- Ding YF, Wei J, Li S, et al. Host-guest interactions initiated supramolecular chitosan nanogels for selective intracellular drug delivery. ACS Appl Mater Interfaces. 2019: 5–10. doi:10.1021/acsami.9b09059.
- Wei P, Gangapurwala G, Pretzel D, et al. Tunable nanogels by host–guest interaction with carboxylate pillar[5]arene for controlled encapsulation and release of doxorubicin. Nanoscale. 2020;12:13595–13605. doi:10.1039/D0NR01881C.
- Wallis M, Al-Dulimi Z, Tan DK, et al. 3D printing for enhanced drug delivery: current state-of-the-art and challenges. Drug Dev Ind Pharm. 2020;46:1385–1401. doi:10.1080/03639045.2020.1801714.
- Cho H, Jammalamadaka U, Tappa K. Nanogels for pharmaceutical and biomedical applications and their fabrication using 3D printing technologies. Materials (Basel). 2018;11. doi:10.3390/ma11020302.
- Mauri E, Perale G, Rossi F. Nanogel functionalization: a versatile approach to meet the challenges of drug and gene delivery. ACS Appl Nano Mater. 2018;1:6525–6541. doi:10.1021/acsanm.8b01686.
- Lu A, Moatsou D, Longbottom DA, et al. Tuning the catalytic activity of l-proline functionalized hydrophobic nanogel particles in water. Chem Sci. 2013;4:965–969. doi:10.1039/c2sc21300a.
- Mauri E, Veglianese P, Papa S, et al. Chemoselective functionalization of nanogels for microglia treatment. Eur Polym J. 2017;94:143–151. doi:10.1016/j.eurpolymj.2017.07.003.
- Raemdonck K, Demeester J, De Smedt S. Advanced nanogel engineering for drug delivery. Soft Matter. 2009;5:707–715. doi:10.1039/B811923F.
- Neamtu I, Rusu AG, Diaconu A, et al. Basic concepts and recent advances in nanogels as carriers for medical applications. Drug Deliv. 2017;24:539–557. doi:10.1080/10717544.2016.1276232.
- Ferguson CTJ, Huber N, Landfester K, et al. Dual-responsive photocatalytic polymer nanogels. Angew Chemie Int Ed. 2019;58:10567–10571. doi:10.1002/anie.201903309.
- Pinelli F, Pizzetti F, Rossetti A, et al. Effect of surface decoration on properties and drug release ability of nanogels. Colloids Surf A Physicochem Eng Asp. 2021;614; doi:10.1016/j.colsurfa.2021.126164.
- Escobedo HD, Stansbury JW, Nair DP. Photoreactive nanogels as versatile polymer networks with tunable in situ drug release kinetics. J Mech Behav Biomed Mater. 2020;108:103755. doi:10.1016/j.jmbbm.2020.103755.
- Gao C, Lin Z, Jurado-Sánchez B, et al. Stem cell membrane-coated nanogels for highly efficient in vivo tumor targeted drug delivery. Small. 2016;12:4056–4062. doi:10.1002/smll.201600624.
- Fang RH, Kroll AV, Gao W, et al. Cell membrane coating nanotechnology. Adv Mater. 2018;30:1–34. doi:10.1002/adma.201706759.
- Deng J, Xu W, Wang J, et al. Cancer cell membrane-coated nanogels as redox/pH dual-responsive drug carrier for tumor-targeted therapy. J Mater Chem B. 2021;XX. doi:10.1039/D1TB00788B.
- A. Pontes da Costa, D.R. Nunes, M. Tharaud, J. Oble, G. Poli, J. Rieger. Palladium(0) nanoparticles embedded in core–shell nanogels as recoverable catalysts for the Mizoroki–Heck reaction. Chem Cat Chem. 2017;9:2167–2175. doi:10.1002/cctc.201601645.
- Zhang Y, Li M, Gao X, et al. Nanotechnology in cancer diagnosis: progress, challenges and opportunities. J Hematol Oncol. 2019;12:137. doi:10.1186/s13045-019-0833-3.
- Barani M, Bilal M, Sabir F, et al. Nanotechnology in ovarian cancer: diagnosis and treatment. Life Sci. 2021;266:118914. doi:10.1016/j.lfs.2020.118914.
- Yang M, Li J, Gu P, et al. The application of nanoparticles in cancer immunotherapy: targeting tumor microenvironment. Bioact Mater. 2021;6:1973–1987. doi:10.1016/j.bioactmat.2020.12.010.
- Zhang F, Gong S, Wu J, et al. CXCR4-targeted and redox responsive dextrin nanogel for metastatic breast cancer therapy. Biomacromolecules. 2017;18:1793–1802. doi:10.1021/acs.biomac.7b00208.
- Chen J, He H, Deng C, et al. Saporin-loaded CD44 and EGFR dual-targeted nanogels for potent inhibition of metastatic breast cancer in vivo. Int J Pharm. 2019;560:57–64. doi:10.1016/j.ijpharm.2019.01.040.
- Shimizu T, Kishida T, Hasegawa U, et al. Nanogel DDS enables sustained release of IL-12 for tumor immunotherapy. Biochem Biophys Res Commun. 2008;367:330–335. doi:10.1016/j.bbrc.2007.12.112.
- Fujii H, Shin-Ya M, Takeda S, et al. Cycloamylose-nanogel drug delivery system-mediated intratumor silencing of the vascular endothelial growth factor regulates neovascularization in tumor microenvironment. Cancer Sci. 2014;105:1616–1625. doi:10.1111/cas.12547.
- Peng S, Wang H, Zhao W, et al. Zwitterionic polysulfamide drug nanogels with microwave augmented tumor accumulation and on-demand drug release for enhanced cancer therapy. Adv Funct Mater. 2020;2001832:1–12. doi:10.1002/adfm.202001832.
- Tsao C-T, Kievit FM, Ali Ravanpay MZ, et al. Thermoreversible poly(ethylene glycol)-g-chitosan hydrogel as a therapeutic T lymphocyte depot for localized glioblastoma immunotherapy. Biomacromolecules. 2014;15:2656–2662.
- Dhoke DM, Basaiyye SS, Khedekar PB. Development and characterization of L-HSA conjugated PLGA nanoparticle for hepatocyte targeted delivery of antiviral drug. J Drug Deliv Sci Technol. 2018;47:77–94. doi:10.1016/j.jddst.2018.06.006.
- Papa S, Veneruso V, Mauri E, et al. Functionalized nanogel for treating activated astrocytes in spinal cord injury. J Control Release. 2021;330:218–228. doi:10.1016/j.jconrel.2020.12.006.
- Giacomazza D, Picone P, Ditta L, et al. Biodistribution of insulin-nanogels in mouse: a preliminary study for the treatment of Alzheimer’s disease. Biophys J. 2017;112:137a. doi:10.1016/j.bpj.2016.11.757.
- Picone P, Ditta LA, Sabatino MA, et al. Ionizing radiation-engineered nanogels as insulin nanocarriers for the development of a new strategy for the treatment of Alzheimer’s disease. Biomaterials. 2016;80:179–194. doi:10.1016/j.biomaterials.2015.11.057.
- Cui W, Liu R, Jin H, et al. PH gradient difference around ischemic brain tissue can serve as a trigger for delivering polyethylene glycol-conjugated urokinase nanogels. J Control Release. 2016;225:53–63. doi:10.1016/j.jconrel.2016.01.028.
- Cheraghi M, Namdari M, Daraee H, et al. Cardioprotective effect of magnetic hydrogel nanocomposite loaded N,α-L-rhamnopyranosyl vincosamide isolated from Moringa oleifera leaves against doxorubicin-induced cardiac toxicity in rats: in vitro and in vivo studies. J Microencapsul. 2017;34:335–341. doi:10.1080/02652048.2017.1311955.
- Azegami T, Yuki Y, Hayashi K, et al. Intranasal vaccination against angiotensin II type 1 receptor and pneumococcal surface protein A attenuates hypertension and pneumococcal infection in rodents. J Hypertens. 2018;36:387–394. doi:10.1097/HJH.0000000000001519.
- Dreiss CA. Hydrogel design strategies for drug delivery. Curr Opin Colloid Interface Sci. 2020;48:1–17. doi:10.1016/j.cocis.2020.02.001.
- Zhu J, Li F, Wang X, et al. Hyaluronic acid and polyethylene glycol hybrid hydrogel encapsulating nanogel with hemostasis and sustainable antibacterial property for wound healing. ACS Appl Mater Interfaces. 2018;10:13304–13316. doi:10.1021/acsami.7b18927.
- Kurt SB, Ayyala RS, Sahiner N. Versatile poly(maltose) micro/nanoparticles with tunable surface functionality as a biomaterial. J Appl Polym Sci. 2021;138:1–13. doi:10.1002/app.49906.
- Sahiner N. Hydrogel nanonetworks with functional core-shell structure. Eur Polym J. 2007;43:1709–1717. doi:10.1016/j.eurpolymj.2007.01.046.
- Wang H, Chen Q, Zhou S. Carbon-based hybrid nanogels: a synergistic nanoplatform for combined biosensing, bioimaging, and responsive drug delivery. Chem Soc Rev. 2018;47:4198–4232. doi:10.1039/C7CS00399D.
- Gyawali D, Kim JP, Yang J. Highly photostable nanogels for fluorescence-based theranostics. Bioact Mater. 2018;3:39–47. doi:10.1016/j.bioactmat.2017.03.001.
- Aktan B, Chambre L, Sanyal R, et al. ‘Clickable’ nanogels via thermally driven self-assembly of polymers: facile access to targeted imaging platforms using thiol–maleimide conjugation. Biomacromolecules. 2017;18:490–497. doi:10.1021/acs.biomac.6b01576.
- Krasia-Christoforou T, Georgiou TK. Polymeric theranostics: using polymer-based systems for simultaneous imaging and therapy. J Mater Chem B. 2013;1:3002–3025. doi:10.1039/C3TB20191 K.
- Mauri E, Veglianese P, Papa S, et al. Double conjugated nanogels for selective intracellular drug delivery. RSC Adv. 2017;48:30345–30356. doi:10.1039/c7ra04584k.
- Park JS, Yi SW, Kim HJ, et al. Sunflower-type nanogels carrying a quantum dot nanoprobe for both superior gene delivery efficacy and tracing of human mesenchymal stem cells. Biomaterials. 2016;77:14–25. doi:10.1016/j.biomaterials.2015.11.002.
- Whiteley Z, Ho HMK, Gan YX, et al. Microfluidic synthesis of protein-loaded nanogels in a coaxial flow reactor using a design of experiments approach. Nanoscale Adv. 2021;3:2039–2055. doi:10.1039/D0NA01051 K.
- Shu T, Shen Q, Zhang X, et al. Stimuli-responsive polymer/nanomaterial hybrids for sensing applications. Analyst. 2020;145:5713–5724. doi:10.1039/D0AN00686F.
- Vicario-de-la-Torre M, Forcada J. The potential of stimuli-responsive nanogels in drug and active molecule delivery for targeted therapy. Gels. 2017;3; doi:10.3390/gels3020016.
- Preman NK, Barki RR, Vijayan A, et al. Recent developments in stimuli-responsive polymer nanogels for drug delivery and diagnostics: a review. Eur J Pharm Biopharm. 2020;157:121–153. doi:10.1016/j.ejpb.2020.10.009.
- Ghaeini-Hesaroeiye S, Bagtash HR, Boddohi S, et al. Thermoresponsive nanogels based on different polymeric moieties for biomedical applications. Gels. 2020;6.
- Peng HS, Stolwijk JA, Sun LN, et al. A nanogel for ratiometric fluorescent sensing of intracellular pH values. Angew Chemie – Int Ed. 2010;49:4246–4249. doi:10.1002/anie.200906926.
- Wu W, Zhou S. Hybrid micro-/nanogels for optical sensing and intracellular imaging. Nano Rev. 2010;1:5730. doi:10.3402/nano.v1i0.5730.
- Wu W, Aiello M, Zhou T, et al. In-situ immobilization of quantum dots in polysaccharide-based nanogels for integration of optical pH-sensing, tumor cell imaging, and drug delivery. Biomaterials. 2010;31:3023–3031. doi:10.1016/j.biomaterials.2010.01.011.
- Wu W, Shen J, Banerjee P, et al. Core-shell hybrid nanogels for integration of optical temperature-sensing, targeted tumor cell imaging, and combined chemo-photothermal treatment. Biomaterials. 2010;31:7555–7566. doi:10.1016/j.biomaterials.2010.06.030.
- Liu WY, Ju XJ, Faraj Y, et al. Capsule membranes encapsulated with smart nanogels for facile detection of trace lead(II) ions in water. J Memb Sci. 2020;613:118523. doi:10.1016/j.memsci.2020.118523.
- Wu W, Mitra N, Yan ECY, et al. Multifunctional hybrid nanogel for integration of optical glucose sensing and self-regulated insulin release at physiological pH. ACS Nano. 2010;4:4831–4839. doi:10.1021/nn1008319.
- Li W, Nie J, Hu R, et al. A nanogel sensor for colorimetric fluorescence measurement of ionizing radiation doses. Chem Commun. 2019;55:9614–9617. doi:10.1039/C9CC03680F.
- Liu J, Liu L, Li S, et al. Self-assembled nanogels of luminescent thiolated silver nanoclusters and chitosan as bactericidal agent and bacterial sensor. Mater Sci Eng. C. 2021;118:111520, doi:10.1016/j.msec.2020.111520.
- Navalón S, García H. Nanoparticles for catalysis. Nanomater. (Basel), Switzerland). 2016;6:123. doi:10.3390/nano6070123.
- Shi J, Wu Y, Zhang S, et al. Bioinspired construction of multi-enzyme catalytic systems. Chem Soc Rev. 2018;47:4295–4313. doi:10.1039/C7CS00914C.
- Cuenca T, Filice M, Palomo JM. Palladium nanoparticles enzyme aggregate (PANEA) as efficient catalyst for Suzuki–Miyaura reaction in aqueous media. Enzyme Microb Technol. 2016;95:242–247. doi:10.1016/j.enzmictec.2016.01.014.
- Jana BA, Shinde U, Wadhwani A. Synthetic enzyme-based nanoparticles act as smart catalyst for glucose responsive release of insulin. J Biotechnol. 2020;324:1–6. doi:10.1016/j.jbiotec.2020.09.023.
- Resmini M, Flavin K, Carboni D. Microgels and nanogels with catalytic activity. Top Curr Chem. 2012;325:307–342. doi:10.1007/128_2010_93.
- Astruc D. Introduction: nanoparticles in catalysis. Chem Rev. 2020;120:461–463. doi:10.1021/acs.chemrev.8b00696.
- Jia H, Gao Z, Ma Y, et al. Preparation and characterization of a highly stable phenoxazinone synthase nanogel. Chem Cent J. 2016;10:1–7. doi:10.1186/S13065-016-0178-8.
- Beloqui A, Kobitski AY, Nienhaus GU, et al. A simple route to highly active single-enzyme nanogels. Chem Sci. 2018;9:1006–1013. doi:10.1039/c7sc04438k.
- Fratoddi I, Venditti I, Battocchio C, et al. Core shell hybrids based on noble metal nanoparticles and conjugated polymers: synthesis and characterization. Nanoscale Res Lett. 2011;6:1–8. doi:10.1186/1556-276X-6-98.
- Vishnu S. K D, Ranganathan P, Rwei SP, et al. New reductant-free synthesis of gold nanoparticles-doped chitosan-based semi-IPN nanogel: a robust nanoreactor for exclusively sensitive 5-fluorouracil sensor. Int J Biol Macromol. 2020;148:79–88. doi:10.1016/j.ijbiomac.2020.01.108.
- Gaur SS, Dhar P, Sakurai S, et al. Cellulose nanocrystal/clay based macroion nanogel as support for stable platinum catalyst for electrochemical oxidation of methanol in alkaline medium. Appl Clay Sci. 2019;182:105277. doi:10.1016/j.clay.2019.105277.
- Shi H, Liu Y, Qu R, et al. A facile one-pot method to prepare peroxidase-like nanogel artificial enzymes for highly efficient and controllable catalysis. Colloids Surf B Biointerfaces. 2019;174:352–359. doi:10.1016/j.colsurfb.2018.11.021.
- Garcia-Martinez J. Chemistry 2030: a roadmap for a new decade. Angew Chemie. 2021;133:5008–5012. doi:10.1002/ange.202014779.
- Sahiner N, Butun S, Ilgin P. Hydrogel particles with core shell morphology for versatile applications: environmental, biomedical and catalysis. Colloids Surf A Physicochem Eng Asp. 2011;386:16–24. doi:10.1016/j.colsurfa.2011.06.023.
- Cringoli MC, Marchesan S, Melchionna M, et al. Nanostructured gels for energy and environmental applications. Gels. 2020;25. doi:10.3390/molecules25235620.
- Demirci S, Sahiner N. PEI-based ionic liquid colloids for versatile use: biomedical and environmental applications. J Mol Liq. 2014;194:85–92. doi:10.1016/j.molliq.2014.01.015.
- Sahiner N, Yasar AO. The generation of desired functional groups on poly(4-vinyl pyridine) particles by post-modification technique for antimicrobial and environmental applications. J Colloid Interface Sci. 2013;402:327–333. doi:10.1016/j.jcis.2013.03.032.
- Mahmud HNME, Kamal SJ, Mohamad N, et al. Nanoconducting polymer: an effective adsorbent for dyes. Chem Pap. 2021; doi:10.1007/s11696-021-01665-0.
- Sengel SB, Sahiner N. Poly(vinyl phosphonic acid) nanogels with tailored properties and their use for biomedical and environmental applications. Eur Polym J. 2016;75:264–275. doi:10.1016/j.eurpolymj.2016.01.007.
- Richa, A. Roy Choudhury. Synthesis of a novel gellan-pullulan nanogel and its application in adsorption of cationic dye from aqueous medium. Carbohydr Polym. 2020;227:115291. doi:10.1016/j.carbpol.2019.115291.
- Hassanpour S, Farshi Azhar F, Bagheri M. Novel nanogels based on hydroxypropyl cellulose–poly(itaconic acid) for adsorption of methylene blue from aqueous solution: process modeling and optimization using response surface methodology. Polym Bull. 2019;76:933–952. doi:10.1007/s00289-018-2419-6.
- Akl MA, Sarhan AA, Shoueir KR, et al. Application of crosslinked ionic poly(vinyl alcohol) nanogel as adsorbents for water treatment. J Dispers Sci Technol. 2013;34:1399–1408. doi:10.1080/01932691.2012.742791.
- Atta AM, Al-Lohedan HA, Tawfik AM, et al. Application of super-amphiphilic silica-nanogel composites for fast removal of water pollutants. Molecules. 2016;21:1–14. doi:10.3390/molecules21101392.
- Wen X, Qiao X, Han L, et al. Multifunctional magnetic branched polyethylenimine nanogels with in-situ generated Fe3O4 and their applications as dye adsorbent and catalyst support. Journal of Materials Science. 2016;51:3170–3181. doi:10.1007/s10853-015-9627-3.