Abstract
Immune-mediated platelet refractoriness (PR) remains a significant problem in the setting of platelet transfusion and is predominantly caused by the presence of alloantibodies directed against class I human leukocyte antigens (HLA). Opsonization of donor platelets with these alloantibodies can result in rapid clearance after transfusion via multiple mechanisms, including antibody dependent cellular phagocytosis (ADCP). Interestingly, not all alloimmunized patients develop PR to unmatched platelet transfusions, suggesting variation in HLA-specific IgG responses between patients. Previously, we observed that the glycosylation profile of anti-HLA antibodies was highly variable between PR patients, especially with respect to Fc galactosylation, sialylation and fucosylation. In the current study, we investigated the effect of different Fc glycosylation patterns, with known effects on complement deposition and FcγR binding, on phagocytosis of opsonized platelets by monocyte-derived human macrophages. We found that the phagocytosis of antibody- and complement-opsonized platelets, by monocyte derived M1 macrophages, was unaffected by these qualitative IgG-glycan differences.
Introduction
Platelet transfusions are a frequently administered therapy to reduce mortality and hemorrhagic complications in patients with thrombocytopenia. A major problem in the setting of platelet transfusions is platelet refractoriness (PR), which refers to a recurring inadequate platelet count increment after multiple platelet transfusions. The incidence of PR ranges from 5% to 15% and varies depending on both patient characteristics and platelet product preparation [Citation1–4]. In approximately 20% of PR cases platelet clearance is immune mediated, predominantly caused by the presence of alloantibodies directed against class I human leukocyte antigens (HLA) and occasionally human platelet antigens (HPA) [Citation5–9]. Opsonization of donor platelets with these alloantibodies can result in clearance shortly after transfusion, via antibody dependent cellular cytotoxicity (ADCC), complement-dependent cytotoxicity (CDC) and/or antibody dependent cellular phagocytosis (ADCP) [Citation10–16]. The major management strategy currently available for heavily transfused alloimmunized patients is extensive matching of platelet transfusion products to prevent PR and subsequent worse clinical outcomes [Citation7–9]. Interestingly, not all alloimmunized patients develop PR to unmatched platelet transfusions [Citation17,Citation18], suggesting variation in qualitative traits of the HLA specific IgG responses between patients.
All IgG molecules contain a conserved N-linked glycan at position 297, located in the Fc-region, affecting the antibody’s structure and function. The glycan is composed of a biantennary core structure of N-acetylglucosamines (GlcNAc) and mannose residues, which can be elongated by a fucose, bisecting GlcNAc and up to two galactose residues, which both can be capped by a sialic acid residue. Antibody Fc glycosylation is highly variable, and altered patterns have previously been described in settings of infection and alloimmunization, which are known to affect antibody effector functions and thereby the progression of associated immune responses [Citation19–27]. For example, the absence of the fucose residue leads to an increased binding affinity of the antibody to FcγRIIIa/b, which can result in increased associated effector functions, such as ADCC and ADCP [Citation19–22,Citation25,Citation28,Citation29]. In addition, galactosylation is especially relevant for complement activation [Citation21,Citation24,Citation30,Citation31]. We and others have recently shown that galactosylation increases the antibody’s capacity to activate the classical complement pathway, through enhanced hexamerization, which in turn increases complement deposition and CDC activity [Citation23,Citation30]. Sialylation slightly enhances complement activation even further [Citation21,Citation23,Citation32], while the bisecting GlcNAc has no effect on either complement activation nor FcγR binding [Citation21].
Previously, we characterized the glycosylation profile of anti-HLA Class I antibodies, detected in hemato-oncological patients receiving platelet transfusions [Citation33] and in patients diagnosed with PR [Citation20]. The anti-HLA IgG-specific glycosylation profile was highly variable between patients. For the majority of patients, we observed an increase in Fc galactosylation and sialylation of HLA-specific IgG in comparison to total IgG. Additionally, a few patients, 2 out of 35, also developed anti-HLA antibodies with extremely low fucosylation levels [Citation33].
In the context of alloimmunization and PR, transfused platelets are mainly thought to be cleared by mononuclear macrophages in the spleen after opsonization with anti-HLA or -HPA alloantibodies [Citation8,Citation10,Citation11,Citation13,Citation34,Citation35]. Several pathways exist in which phagocytes can detect their targets for phagocytosis, via non-opsonic and opsonic receptors. The non-opsonic receptors are essential for the recognition of pathogen-associated molecular patterns (PAMPs) and apoptotic cells, whereas the opsonic receptors recognize cells targeted for clearance with opsonins, such as IgG and complement components. The most important and efficient phagocytic receptors for recognizing IgG are FcγRI (CD64), FcγRII (CD32), and FcγRIII (CD16) and Complement Receptor 3 (CR3 or CD11b/CD18) for the recognition of iC3b and C3d [Citation36,Citation37]. Splenic macrophages have a high expression of CR3, FcγRI, FcγRII, and FcγRIII [Citation14,Citation38–41]. Upon opsonization of platelets by IgG or complement components, platelets become susceptible to bind phagocytic receptors expressed by splenic macrophages, which leads to subsequent phagocytosis and destruction. This process is further enhanced by the slow passage of platelets through splenic sinusoids [Citation7,Citation11,Citation14]. However, involvement of the complement system as well as platelet-intrinsic factors, such as platelet apoptosis and activation upon opsonization, have also recently been suggested to be involved in reduced platelet survival in PR [Citation12,Citation15,Citation35,Citation42–45].
Interestingly, very little is known about the effect of antibody Fc glycosylation on platelet clearance by macrophages upon opsonization with differently glycosylated anti-HLA alloantibodies. Especially in light of recent findings regarding effects of antibody Fc glycosylation on both FcγR binding and complement deposition, it is important to gain more insight into effects of antibody Fc glycosylation on clearance mechanisms involved in PR upon alloimmunization. In the current study, we investigated the influence of different Fc glycosylation patterns that are known to affect complement deposition and FcγR affinity on opsonized platelet phagocytosis by monocyte-derived human macrophages. Monocyte-derived M1 macrophages were used due to their high expression levels of FcγRs as well as CR3, which are also highly expressed by human splenic macrophages. We found that an increase in complement deposition and/or FcγRIIIa/b affinity via altered Fc glycosylation profiles did not affect phagocytosis of IgG opsonized platelets by human monocyte-derived macrophages in this in vitro model.
Material and methods
Human blood samples
Monocytes were isolated from buffy coats obtained from anonymized Sanquin blood donors after written informed consent. Platelets were isolated from citrated whole blood from anonymous, healthy volunteers with informed written consent. Monocytes and platelets were not obtained from the same individuals. All procedures were approved by the Sanquin Ethical Advisory Board and are in accordance with the declaration of Helsinki and Dutch regulations.
Production of recombinant glycoengineered anti-HLA monoclonal antibodies
The production and glycoengineering techniques of the anti-HLA mAbs used in this study have been described in detail [Citation46–52]. In brief, the protein sequences of the variable regions of all anti-HLA mAbs were used to assemble pcDNA3.1 expression vectors encoding for full human IgG1 and PG LA LA Fc mutants (P329 G, L234A, and L235A), which are incapable of binding complement and FcγRs [Citation53]. The expression vectors were used for the production of recombinant antibodies in our in-house HEK Freestyle system. For obtaining antibodies with certain desired glycan-profiles, the chemical inhibitor 2-deoxy-2-fluoro-L-fucose (2FF, Carbosynth) was used to decrease fc fucosylation and 5 mM D-galactose (Sigma Aldrich) and the constructs coding for the enzymes β-1,4 galactosyltransferase 1 (B4GALT1) and β-galactoside alpha-2,6-sialyltransferase 1 (ST6GALT1) were used prior/during transfection to increase galactosylation and sialylation. Monoclonal antibodies were purified 6 days post-transfection and subjected to liquid chromatography–mass spectrometry-based IgG Fc glycosylation analysis [Citation46,Citation47,Citation54].
Surface Plasmon Resonance (SPR)
The antibody binding to human FcγR classes was assessed by surface plasmon resonance (SPR) on the IBIS M×96 (IBIS technologies) as previously described [Citation55,Citation56]. All C-terminally biotinylated hFcγRs were spotted using a Continuous Flow Microspotter (Wasatch Microfluidics) onto a single SensEye G-streptavidin sensor (Ssens), which allows binding affinity measurements for each antibody to all hFcγR simultaneously. The biotinylated hFcγRs were spotted in threefold dilutions, ranging from 30 nM to 1 nM for hFcγRIIa-H131, hFcγRIIIa-F158, hFcγRIIIb-NA1 and hFcγRIIIb-NA2, from 10 nM to 0.3 nM for hFcγRIIa-R131 and hFcγRIIb, and ranging from 100 nM to 3 nM for hFcγRIIIa-V158 in PBS supplemented with 0.075% Tween-80 (VWR, M126–100 ml), pH 7.4. Regeneration was performed after each sample with 10 nM Gly-HCl, pH 2.0. The dissociation constant (KD) was calculated using equilibrium fitting to Rmax = 500. Analysis and calculation of all binding data was done with Scrubber software version 2 (Biologic Software) and Excel.
Monocyte isolation and differentiation to monocyte-derived macrophage
Monocytes were isolated from buffy-coat derived PBMCs using CD14+ magnetic microbead separation (Miltenyi Biotec) and subsequently frozen until further use as previously described [Citation44,Citation57]. Flow cytometry was used to determine monocyte purity, which was >90%. Monocytes were differentiated to monocyte-derived macrophages as described [Citation58]. Briefly, monocytes were thawed on day 0 and differentiated in a 24-well culture plate (0.25x106 monocytes per well) in the presence of 10 ng/mL granulocyte-macrophage colony-stimulating factor (GM-CSF, CellGenix) in IMDM 1640 (Lonza) containing 10% fetal calf serum (Bodinco) 100 U/mL penicillin and 100 U/mL streptomycin (both Gibco) at 5% CO2 37°C. Cells were cultured for a total of 9 days and fresh medium and GM-CSF were added on day 3 of culture.
Platelet isolation, labeling and opsonization
Platelets from healthy volunteers with known HLA typing were isolated from Platelet Rich Plasma (PRP), by centrifuging citrated whole blood at 125 g for 20 minutes, optimized with methods described previously to avoid platelet activation [Citation12,Citation16]. Hereafter, 10 vol% ACD (acid citrate dextrose, 85 mM Na3-citrate·2 H2O, 71 mM citric acid·H2O and 111 mM D-glucose) was added. The PRP was centrifuged (850 g for 8 min) and washed 2 times with wash buffer (WB; 36 mM citric acid·H2O, 103 mM NaCl, 5 mM KCl, 5 mM EDTA, 5.6 mM D-glucose, pH 6.5). The platelets were set to a concentration of 6 × 108 cells/mL in PBS and incubated for 20 min at RT with 3.75 µM PKH26 (Sigma Aldrich) on a roller bank. 10 vol% FCS was added to terminate the labeling process and the labeled platelets were washed with WB and resuspended in PBS +0.5% BSA. 5 × 106 platelets were incubated with equal volumes of recombinant anti-HLA antibodies and pooled complement sufficient human serum, for 30 min at RT. For some conditions, the serum was pre-incubated for 30 min at 56°C to inactivate complement. The platelets were washed 3 times with PBS +0.5% BSA +5 mM EDTA and resuspended in macrophage culture medium. Complement deposition (C3b) was assessed by staining a small fraction of the platelets with anti-complement C3b/iC3b-APC Antibody Clone: 3E7/C3b (1/250, Biolegend)
Phagocytosis of opsonized platelets by macrophages
For the phagocytosis assay, opsonized platelets were incubated for 30 min at 37°C with allogeneic macrophages at a 1:40 macrophage:platelet ratio. For some conditions, the macrophages were pre-incubated for 30 min at RT with 10 µg/mL FcγR blocking antibodies (anti-CD16, anti-CD32, and/or anti-CD64) and isotype control. Anti-CD64 (clone 10.1, blocking FcγRI) was ordered as f(ab’)2 fragments from Ancell Corporation, whereas the anti-CD32 (clone AT10, blocking FcγRIIa/b/c), anti-CD16 (clone 3G8, blocking FcγRIIIa/b) and isotype (anti-biotin) were cloned and produced as hIgG1 N297A P329 G, L234A, and L235A, making them incapable of binding C1q and FcγRs [Citation53]. Hereafter, cells were washed with PBS and harvested using 130 mM lidocaine (Sigma Aldrich) with 10 mM EDTA (Merck). After harvesting, macrophages were kept on ice and washed with ice-cold PBS +0.5% bovine serum albumin (BSA, Sigma Aldrich) + 2 mM EDTA (Merck) and subsequently with ice-cold PBS. After harvesting, cells were fixed with 3.7% paraformaldehyde (Sigma Aldrich) in PBS for 15 min at RT. Next, cells were washed with PBS +0.5% BSA and stained using APC-labeled anti-HLA-DR (clone L243, BD Biosciences) and BV421-labeled anti-CD42a (clone ALMA.16, BD Biosciences) for 20 min at RT in PBS +0.5% BSA. Cells were washed with PBS +0.5% BSA and analyzed using BD LSR II flow cytometer and imaging flow cytometry (ImageStreamX Mark II Imaging Flow Cytometry, Merck Millipore). Flow cytometry data was analyzed using Flowjo v 10.8.1; cells were gated based on FSC/SSC, single cells, and HLA-DR+, after which cells were gated on positivity for PKH26-labeled platelets and anti-CD42a-BV421 (PKH26+ CD42a-: phagocytosed; PKH+ CD42a+: bound platelets; Supplementary Figure S3A). Imaging flow cytometry data was analyzed using IDEAS v6 software and involved gating on Aspect Ratio Intensity/Area Ch01 to gate cells, and subsequently cells in focus (Gradient RMS Ch01) and HLA-DR+ cells to gate macrophages, after which cells were gated on positivity for PKH26-labeled platelets and anti-CD42a-BV421 (Supplementary Figure S3B).
Statistics
Statistical analyses were executed within GraphPad Prism 8.02 (263) for Windows. Bar graphs were analyzed using ordinary one-way ANOVAs with Dunnett multi-comparison test. The level of significance was set at p ≤ .05. *, **, *** and **** denote a statistical significance of p < .05, ≤.01, ≤.001 and ≤.0001, respectively.
Results
In order to determine the effect of Fc glycosylation of anti-HLA alloantibodies on complement and/or FcγR-mediated phagocytosis of platelets, a system was set up to monitor platelet phagocytosis by human monocyte-derived macrophages. Anti-HLA monoclonal antibodies (mAbs) with altered glycosylation profiles (Supplemental Figure S1A-C), were produced as described [Citation46,Citation47] and subjected to liquid chromatography–mass spectrometry based IgG Fc glycosylation analysis to confirm the intended glycosylation profiles (Supplemental Figure S1D).
Platelets were incubated with unmodified and glycoengineered anti-HLA hIgG1 mAbs (SN230G6, SN607D8 and W6/32) in presence of complement sufficient serum, enabling antibody, and complement opsonization. Only combinations of SN230G6 and SN607D8 antibodies resulted in strong C3b deposition, although the pan-HLA class I-recognizing W6/32 (Supplemental Figure S1B) caused complement deposition on its own (Supplemental Figure S1E), in line with our previous observations [Citation47]. Antibodies with elevated galactosylation and sialylation significantly enhanced complement deposition. Afucosylation of the mAbs had no effect on complement deposition (Supplemental Figure S1E). Neither the PG LALA Fc mutant, incapable of binding C1q and FcγRs [Citation53], nor heat inactivated serum (HI serum) resulted in C3b deposition (Supplemental Figure S1F). Binding of these glycoengineered antibodies to human FcγRs was evaluated by SPR array, which confirmed the increase in affinity of the afucosylated variants for FcγRIIIa/b, whereas no affinity differences were observed for FcγRIIa/b (Supplemental Figure S2).
Opsonized PKH26-labeled platelets were co-incubated with monocyte-derived M1-like macrophages (). These differentiated macrophages express all classes of FcγR (FcγRI (CD64), FcγRII (CD32), FcγRIII (CD16)) as well as CR3 (CD11b/CD18) (), all crucial receptors involved in phagocytosis [Citation8,Citation11,Citation36,Citation37,Citation59]. Internalization of platelets was determined employing imaging and conventional flow cytometry (gating strategies are depicted in Supplemental Figure S3A and 3B, respectively). The platelet-specific marker CD42a was used to detect platelets on the exterior of the macrophages (). The majority of platelet positive (PKH+) macrophages was CD42a-. Moreover, using imaging flow cytometry the majority of PKH+ CD42a+ events was shown to be comprised of macrophages that had both phagocytosed (PKH+ CD42a-) and bound (PKH+ CD42a+) platelets simultaneously, indicating the total PKH+ compartment to be relevant for the quantification of platelet phagocytosis ( and Supplementary Figure S3A, lower panel). Therefore, the total compartment of PKH+ macrophages was analyzed by conventional flow cytometry to assess platelet phagocytosis as exclusion of PKH+ CD42a+ events would lead to an underestimation of phagocytosis efficiency.
Figure 1. A) Schematic overview of the experimental set-up to monitor phagocytosis of opsonized platelets: CD14+ monocytes were cultured for 9 days with GM-CSF to differentiate into monocyte-derived macrophages (MQ M1). Hereafter, the macrophages were pre-incubated with and without FcγR-blockers. Freshly isolated platelets from HLA-A2+ donors were labeled with PKH26 and pre-incubated with unmodified and glycoengineered anti-HLA monoclonal antibodies in the presence of complement sufficient or heat-inactivated (HI) serum, for antibody and complement opsonization. The macrophages and platelets were washed and co-incubated for 30 minutes at 37°C and analyzed by flow cytometry and Imagestream B) Expression levels of complement receptor 3 (Cd11b/cd18) and FcγR’s (FcγRI, FcγRII, FcγRIII) on the surface of the monocyte-derived macrophages as analyzed by flow cytometry. C-E) Internalization of platelets was determined employing C) conventional and D-E) imaging flow cytometry. Anti-CD42a-BV421 staining, in combination with PKH26, was used to identify platelets attached to the exterior of the cell. Representative images are shown for indicated quadrants obtained by imaging flow cytometry.
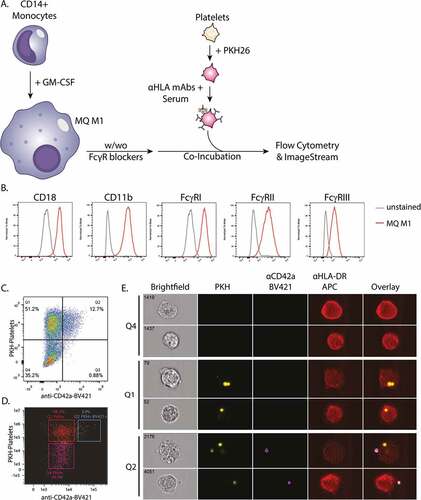
Platelet phagocytosis was significantly increased when macrophages were incubated with platelets opsonized with unmodified anti-HLA mAbs SN230G6+SN607D8, compared to unopsonized platelets (). Phagocytosis was observed in an antibody concentration-dependent manner with the maximum % of phagocytic MQ at 1 µg/mL. Although the glycoengineered mAbs with elevated galactosylation and sialylation levels significantly enhanced complement deposition on platelets (Supplemental Figure S1E), these did not result in higher levels of subsequent phagocytosis (). Also, opsonization with afucosylated IgG, which increases the affinity to FcγRIII ([Citation21] and Supplemental Figure S2), did not enhance phagocytosis compared to the unmodified antibodies (). Moreover, incubating the platelets with the separate unmodified anti-HLA mAbs SN230G6 or SN607D8 resulted in enhanced phagocytosis (Supplementary Figure S4A-B) and was not affected by antibody Fc glycan modifications (Supplementary Figure S4C-D). Similar to the combination SN230G6 + SN607D8, incubating the platelets with the pan-HLA Class I antibody W6/32 resulted in a significantly increased phagocytosis compared to unopsonized platelets (). Phagocytosis was observed in an antibody concentration-dependent manner and was unaffected by any of the antibody glycan modifications (). These results suggested that the observed phagocytosis was not primarily mediated through either complement receptors or FcγRIIIa/b.
Figure 2. Phagocytosis of complement and antibody opsonized platelets by monocyte-derived macrophages A) Absolute and B) relative levels of phagocytosis of platelets incubated with various concentrations of unmodified hIgg1 anti-HLA monoclonal antibodies (mAb) SN230G6+SN607D8 in the presence of complement sufficient serum C) Differences in phagocytosis between platelets pre-incubated with unmodified and glycoengineered hIgg1 SN230G6+SN607D8 mAbs in the presence of complement sufficient serum. D) Absolute and E) relative levels of phagocytosis of platelets pre-incubated with various concentrations of unmodified pan-anti-HLA Class I hIgg1 mAb W6/32 in the presence of complement sufficient serum F) Differences in phagocytosis between platelets pre-incubated with unmodified and glycoengineered hIgg1 W6/32 mAb in the presence of complement sufficient serum. G-H) Relative phagocytosis levels of platelets pre-incubated with 1 µg/ml unmodified or PG LA LA Fc mutant anti-HLA mAbs (SN230G6+SN607D8 or W6/32) in the presence of complement sufficient or heat-inactivated (HI) Serum A-H) the level of phagocytosis (%) was defined as the percentage of the PKH26+ macrophage fraction (Q1+Q2). The data represents the mean and SD of 2 technical replicates of 2–5 different monocyte donors used in 2–4 independent experiments, for each independent experiment also a different platelet donor was used. The color of the data points indicates the different monocyte donors. For normalization, the level of phagocytosis of platelets incubated with 1 µg/ml unmodified anti-HLA mAbs was set at 1, as indicated. An ordinary one-way ANOVA with Dunnet’s multicomparison test was performed for the statistical analysis. *p ≤ .05, ****p ≤ .0001 and ns = non-significant .
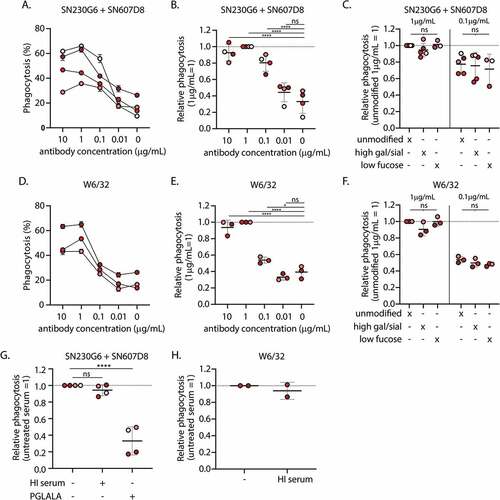
In line with this, platelets opsonized with HI sera did not result in diminished phagocytosis by macrophages (). However, platelet opsonization with PG LA LA Fc mutants significantly abrogated phagocytosis (), suggesting phagocytosis to be FcγR dependent but complement independent. The same trend was observed for W6/32, for which phagocytosis appeared to be unaffected by HI serum (). However, it has to be noted that incubating platelets with the unmodified W6/32 antibody in presence of complement sufficient serum only leads to low C3b deposition (Supplemental Figure S1E).
To preliminarily assess which FcγR may be involved in the phagocytosis of the anti-HLA opsonized platelets, FcγR-blocking antibodies were used. While blocking either FcγRII or FcγRIII resulted in a negligible decrease in phagocytosis, blocking FcγRI alone or in combination with FcγRII and FcγRIII, resulted in reduced phagocytosis of opsonized platelets by monocyte-derived macrophages (Supplemental Figure S4E-F). We hypothesized that upon blockade of FcγRI, increased affinity for FcγRIII may become apparent for platelets opsonized with low fucosylated antibodies. Strikingly, the presence of the FcγRI blocking antibody did not seem to affect the phagocytosis of low fucosylated W6/32 antibodies (Supplemental Figure S4G).
Discussion
Alloimmunization to HLA or HPA antigens is associated with a higher incidence of platelet refractoriness (PR). However, not all alloimmunized patients develop PR upon transfusion with unmatched platelet products, which may be explained by variations in the quality of allospecific IgG responses between patients as well as the differential immunogenicity of non-matched transfusion products [Citation1–4,Citation17,Citation18]. Previously, we reported on differences in Fc glycosylation between total IgG and anti-HLA specific IgG detected in patients receiving platelet transfusions [Citation33] and patients diagnosed with PR [Citation20]. We hypothesized that these differences in Fc glycosylation might explain the observed inter-donor variation in immune responses. Observed differences in glycosylation included both increased sialylation/galactosylation of anti-HLA specific IgG, for the majority of the patients, as well as a severe decrease in fucosylation in a few patients [Citation33]. These differences in antibody Fc glycosylation can affect antibody effector functions, including ADCC, ADCP, and/or CDC, and thereby platelet clearance upon opsonization of platelets with differently glycosylated anti-HLA alloantibodies [Citation22,Citation23]. Previously, we have shown that anti-HLA mAbs with different specificities, binding simultaneously to the same HLA-molecules interact synergistically to activate complement on platelets [Citation16,Citation47]. Also, elevated galactosylation and sialylation of anti-HLA mAbs enhanced complement deposition and CDC activity even further [Citation47]. However, the effect of anti-HLA antibody Fc glycosylation on platelet phagocytosis by macrophages has not been studied before.
Here, we used an in vitro system to investigate the effect of Fc glycosylation of anti-HLA antibodies on phagocytosis of antibody and complement opsonized platelets by monocyte-derived macrophages. Anti-HLA mAbs were glycoengineered with increased levels of galactosylation and sialylation or decreased fucosylation levels, as observed in patients receiving platelet transfusions and with PR. We found that phagocytosis of opsonized platelets by monocyte-derived macrophages was unaffected by differential Fc glycosylation of anti-HLA antibodies and resulting differences in complement deposition or increased FcγRIII affinity, possibly because phagocytosis appeared primarily mediated through FcγRI.
Few studies have looked into the effect of antibody Fc glycosylation on platelet phagocytosis. Previously, we observed an increase of platelet phagocytosis upon anti-TNP opsonization of TNP haptenized platelets with low fucosylated antibodies by neutrophils and FcγRIIIa+ monocytes, whereas no effect of decreased Fc fucosylation was found on phagocytosis by FcγRIIIa- monocytes [Citation20]. In the context of ITP, phagocytosis of anti-GPIIb/IIIa opsonized platelets by splenic macrophages was found to be mediated primarily through FcγRI and FcγRIII [Citation38]. Another study by Bruggeman et al., observed no effect of low Fc fucosylation on anti-RhD opsonized erythrocyte phagocytosis by human monocyte-derived macrophages, whereas NK-cell mediated ADCC was affected [Citation60]. These studies have indicated varying effects of antibody Fc glycan structures on the clearance of platelets and red blood cells by different effector cells, which may largely be explained by the effector cell FcγR expression profile (e.g. expression of high-affinity receptor FcγRI). In line with the literature, we found that monocyte-derived macrophages expressed both CR3 and FcγRI-III [Citation60,Citation61] similarly to ex vivo splenic macrophages [Citation14,Citation38]. However, it would be interesting to directly compare the FcγR expression levels and phagocytosis efficiency of both in vitro generated and ex vivo isolated splenic macrophages to gain more insight on the role of splenic clearance in the setting of PR.
Furthermore, in vivo phagocytosis may also differ from our observations due to the presence of aspecific antibodies saturating the high affinity FcγRI, thereby allowing for effects of increased affinity for FcγRIII upon low antibody Fc fucosylation. Interestingly, we did not observe increased platelet phagocytosis upon opsonization by low fucosylated antibodies in conditions in which FcγRI was blocked. More research exploring in vivo clearance dynamics are warranted, for example through in vitro phagocytosis in the presence of serum or aspecific antibodies or through animal experiments as a proxy for PR in patients.
Next to FcγR-mediated phagocytosis, platelet clearance upon transfusion leading to PR can also be mediated (in part) by complement activation on the platelet surface [Citation8,Citation15]. It can occur directly through the classical complement pathway, leading to the formation of a membrane attack complex (MAC) [Citation35] or indirectly by targeting the platelets with complement deposition for phagocytosis, through binding of iC3b to CR3 on splenic macrophages [Citation36,Citation37,Citation62]. Complement deposition on platelets upon opsonization with anti-HLA alloantibodies has previously been described [Citation16] and shown to be affected by increased levels of galactosylation and sialylation [Citation23,Citation24]. In our current setup, we found no evidence for complement mediated phagocytosis by monocyte-derived macrophages, most likely due to the high affinity of FcγRI. Moreover, platelets were opsonized at room temperature to avoid direct complement mediated lysis, which may play an important role in clearance of transfused platelets in vivo in the setting of PR. Furthermore, next to increased complement deposition, platelets may also become activated or damaged due to either opsonization by anti-HLA antibodies or through platelet storage lesion. Apoptosis and subsequent phosphatidylserine exposure have been described to result in increased phagocytosis of platelets by dendritic cells and macrophages [Citation10,Citation12,Citation44]. In addition, platelet activation can occur after binding of some anti-HLA mAbs via crosslinking between HLA and FcγRIIa, through Syk signaling, leading to aggregation and increased phagocytosis by monocyte-derived macrophages [Citation12]. The protocols used in the current study were optimized for minimal platelet activation by means of the addition of calcium chelators during isolation; although baseline activation cannot be completely avoided when handling platelets and can be induced upon opsonization by anti-HLA antibodies and complement deposition [Citation12,Citation16]. However, the introduced Fc glycosylation changes of the anti-HLA mAbs have no effect on FcγRIIa affinity/binding [Citation21] and thereby are unlikely to affect platelet activation. Therefore, we do not consider it likely that differences in platelet activation due to antibody Fc glycosylation changes influence our observed findings.
In summary, we have shown that phagocytosis of anti-HLA opsonized platelets by monocyte-derived macrophages appeared primarily mediated by FcγRI, and differential antibody Fc glycosylation did not affect phagocytosis efficiency in the current in vitro setup. However, future studies are warranted, especially to account for the multiple pathways potentially involved in platelet clearance upon alloimmunization in vivo in the setting of PR, which can all be affected by the glycosylation profile of alloantibodies. Detailed understanding of platelet clearance upon alloimmunization is important to gain more insight in the pathophysiology of PR and the potential use of complement inhibitors or other immune suppressors in the treatment of PR upon platelet transfusion.
Authorship contributions
T.L.J.v.O., J.S., J.V., S.M.v.H., M.W., A.t.B. and G.V. designed and supervised experimental work. T.L.J.v.O., J.S., J.N., C.A.M.K., A.E.H.B. performed experiments and collected data. S.H. and A.M. developed vital reagents. T.L.J.v.O, J.S., A.t.B. and G.V. wrote the manuscript, which was edited by all authors. All authors analyzed and interpreted data and approved the manuscript.
Supplemental Material
Download PDF (5.9 MB)Acknowledgements
The authors would like to thank Rick Kapur, Leendert Porcelijn, C. Ellen van der Schoot and Masja de Haas for constructive feedback during discussions and the Sanquin Research Central Facility for their technical assistance with conventional and imaging flow cytometry.
Disclosure statement
No potential conflict of interest was reported by the authors.
Supplemental material
Supplemental data for this article can be accessed online at https://doi.org/10.1080/09537104.2022.2129604.
Additional information
Funding
References
- Bub CB, Gonçalez AC, Barjas-Castro ML, Castro V. Prospective evaluation of platelet refractoriness in haematological patients in a single Brazilian institution. ISBT Sci Ser. 2021;16(1):1–9.
- Hu X, Cai H, Zheng L, Luo Y, Zhou J, Hui Y, Dai Z, Lin H, Li D, Xiao Y, et al. Clinical and immunological features of platelet transfusion refractoriness in young patients with de novo acute myeloid leukemia. Cancer Med. 2020;9(14):4941–4948. doi: 10.1002/cam4.3140.
- Comont T, Tavitian S, Bardiaux L, Fort M, Debiol B, Morère D, Bérard E, Delabesse E, Luquet I, Martinez S, et al. Platelet transfusion refractoriness in patients with acute myeloid leukemia treated by intensive chemotherapy. Leuk Res. 2017;61:62–67. doi: 10.1016/j.leukres.2017.08.015.
- Hess JR, Trachtenberg FL, Assmann SF, Triulzi DJ, Kaufman RM, Strauss RG, Granger S, Slichter SJ. Clinical and laboratory correlates of platelet alloimmunization and refractoriness in the PLADO trial. Vox Sang. 2016;111(3):281–291. doi: 10.1111/vox.12411
- Novotny VM, van Doorn R, Witvliet MD, Claas FH, Brand A. Occurrence of allogeneic HLA and non-HLA antibodies after transfusion of prestorage filtered platelets and red blood cells: a prospective study. Blood. 1995;85(7):1736–1741.
- Jia Y, Li W, Liu N, Zhang K, Gong Z, Li D, Wang L, Wang D, Jing Y, Wang J, et al. Prevalence of platelet-specific antibodies and efficacy of crossmatch-compatible platelet transfusions in refractory patients. Transfus Med. 2014;24(6):406–410. doi: 10.1111/tme.12157.
- Pavenski K, Freedman J, Semple JW. HLA alloimmunization against platelet transfusions: pathophysiology, significance, prevention and management. Tissue Antigens. 2012;79(4):237–245.
- Saris A, Pavenski K. Human leukocyte antigen alloimmunization and alloimmune platelet refractoriness. Transfus Med Rev. 2020;34(4):250–257.
- Vassallo RR. Recognition and management of antibodies to human platelet antigens in platelet transfusion-refractory patients. Immunohematology. 2009;25(3):119–124
- Badlou BA, Ya PW, Smid WM, Akkerman JWN. Platelet binding and phagocytosis by macrophages. Transfusion. 2006;46(8):1432–1443.
- Grozovsky R, Hoffmeister KM, Falet H. Novel clearance mechanisms of platelets. Curr Opin Hematol. 2010;17(6):585–589.
- Rijkers M, Saris A, Heidt S, Mulder A, Porcelijn L, Claas FH, Bierings R, Leebeek FW, Jansen AG, Vidarsson G, et al. A subset of anti-HLA antibodies induces Fcγriia-dependent platelet activation. Haematologica. 2018;103(10):1741–1752.
- Aslam R, Kapur R, Segel GB, Guo L, Zufferey A, Ni H, Semple JW. The spleen dictates platelet destruction, anti-platelet antibody production, and lymphocyte distribution patterns in a murine model of immune thrombocytopenia. Exp Hematol. 2016;44(10):924–930.e1. doi: 10.1016/j.exphem.2016.07.004.
- Audia S, Santegoets K, Laarhoven AG, Vidarsson G, Facy O, Ortega-Deballon P, Samson M, Janikashvili N, Saas P, Bonnotte B, et al. Fcγ receptor expression on splenic macrophages in adult immune thrombocytopenia. Clin Exp Immunol. 2017;188(2):275–282. doi: 10.1111/cei.12935.
- Meinke S, Karlström C, Höglund P. Complement as an immune barrier in platelet transfusion refractoriness. Transfus Med Rev. 2019;33(4):231–235.
- Rijkers M, Schmidt D, Lu N, Kramer CS, Heidt S, Mulder A, Porcelijn L, Claas FH, Leebeek FW, Jansen AG, et al. Anti-HLA antibodies with complementary and synergistic interaction geometries promote classical complement activation on platelets. Haematologica. 2019;104(2):403–416.
- Rebulla P. Refractoriness to platelet transfusion. Curr Opin Hematol. 2002;9(6):516–520.
- Group T to RA to PS. Leukocyte reduction and ultraviolet B irradiation of platelets to prevent alloimmunization and refractoriness to platelet transfusions. N Engl J Med. 1997;337(26):1861–1870.
- Wuhrer M, Porcelijn L, Kapur R, Koeleman CAM, Deelder AM, de Haas M, Vidarsson G. Regulated glycosylation patterns of IgG during alloimmune responses against human platelet antigens. J Proteome Res. 2009;8(2):450–456. doi: 10.1021/pr800651j.
- Kapur R, Kustiawan I, Vestrheim A, Koeleman CAM, Visser R, Einarsdottir HK, Porcelijn L, Jackson D, Kumpel B, Deelder AM, et al. A prominent lack of IgG1-Fc fucosylation of platelet alloantibodies in pregnancy. Blood. 2014;123(4):471–480. doi: 10.1182/blood-2013-09-527978.
- Dekkers G, Treffers L, Plomp R, Bentlage AEH, de Boer M, Koeleman CAM, Lissenberg-Thunnissen SN, Visser R, Brouwer M, Mok JY, et al. Decoding the human immunoglobulin G-glycan repertoire reveals a spectrum of Fc-receptor- and complement-mediated-effector activities. Front Immunol. 2017;8(AUG): doi:10.3389/fimmu.2017.00877.
- Larsen MD, de Graaf EL, Sonneveld ME, Plomp HR, Nouta J, Hoepel W, Chen H-J, Linty F, Visser R, Brinkhaus M, et al. Afucosylated IgG characterizes enveloped viral responses and correlates with COVID-19 severity. Science. 2021;371(6532): doi: 10.1126/science.abc8378.
- van Osch TLJ, Nouta J, Derksen NIL, van Mierlo G, van der Schoot CE, Wuhrer M, Rispens T, Vidarsson G. Fc Galactosylation promotes hexamerization of human IgG1, leading to enhanced classical complement activation. J Immunol. 2021;207(6):1545–1554. doi: 10.4049/jimmunol.2100399.
- Peschke B, Keller CW, Weber P, Quast I, Lünemann JD. Fc-Galactosylation of human immunoglobulin gamma isotypes improves C1q binding and enhances complement-dependent cytotoxicity. Front Immunol. 2017;8(JUN):646.
- Sonneveld ME, Natunen S, Sainio S, Koeleman CAM, Holst S, Dekkers G, Koelewijn J, Partanen J, van der Schoot CE, Wuhrer M, et al. Glycosylation pattern of anti-platelet IgG is stable during pregnancy and predicts clinical outcome in alloimmune thrombocytopenia. Br J Haematol. 2016;174(2):310–320. doi: 10.1111/bjh.14053.
- Wang TT, Maamary J, Tan GS, Bournazos S, Davis C, Krammer F, Schlesinger S, Palese P, Ahmed R, Ravetch J. Anti-HA Glycoforms drive B cell affinity selection and determine influenza vaccine efficacy. Cell. 2015;162(1):160–169. doi: 10.1016/j.cell.2015.06.026.
- Selman MHJ, De Jong SE, Soonawala D, Kroon FP, Adegnika AA, Deelder AM, Hokke CH, Yazdanbakhsh M, Wuhrer M. Changes in antigen-specific IgG1 Fc N-glycosylation upon influenza and tetanus vaccination. Mol Cell Proteomics. 2012;11(4):1–10. doi: 10.1074/mcp.M111.014563
- Vidarsson G, Dekkers G, Rispens T. IgG subclasses and allotypes: from structure to effector functions. Front Immunol. 2014;5(OCT):1–17.
- Temming AR, de Taeye SW, de Graaf EL, de Neef LA, Dekkers G, Bruggeman CW, Koers J, Ligthart P, Nagelkerke SQ, Zimring JC, et al. Functional attributes of antibodies, effector cells, and target cells affecting NK cell–mediated antibody-dependent cellular cytotoxicity. J Immunol. 2019;203(12):3126–3135. doi: 10.4049/jimmunol.1900985.
- Wei B, Gao X, Cadang L, Izadi S, Liu P, Zhang HM, Hecht E, Shim J, Magill G, Pabon JR, et al. Fc galactosylation follows consecutive reaction kinetics and enhances immunoglobulin G hexamerization for complement activation. MAbs. 2021;13(1):1–10.
- Quast I, Keller CW, Maurer MA, Giddens JP, Tackenberg B, Wang L-X, Münz C, Nimmerjahn F, Dalakas MC, Lünemann JD. Sialylation of IgG Fc domain impairs complement-dependent cytotoxicity. J Clin Invest. 2015;125(11):4160–4170. doi: 10.1172/JCI82695.
- Wada R, Matsui M, Kawasaki N. Influence of N-glycosylation on effector functions and thermal stability of glycoengineered IgG1 monoclonal antibody with homogeneous glycoforms. MAbs. 2019;11(2):350–372.
- van Osch TLJ, Pongracz T, Geerdes DM, Mok JY, van Esch WJE, Voorberg J, Kapur R, Porcelijn L, Kerkhoffs JLH, van der Meer PF, et al. Altered Fc glycosylation of anti-HLA alloantibodies in haemato-oncological patients receiving platelet transfusions. J Thromb Haemost.2022. doi: 10.1111/jth.15898.
- Crow AR, Lazarus AH. Role of Fcgamma receptors in the pathogenesis and treatment of idiopathic thrombocytopenic purpura. J Pediatr Hematol Oncol. 2003;25 Suppl(1):S14–8.
- Meinke S, Sandgren P, Mörtberg A, Karlström C, Kadri N, Wikman A, Höglund P. Platelets made HLA deficient by acid treatment aggregate normally and escape destruction by complement and phagocytes in the presence of HLA antibodies. Transfusion. 2016;56(2):370–382. doi: 10.1111/trf.13350.
- Uribe-Querol E, Rosales C. Phagocytosis: our current understanding of a universal biological process. Front Immunol. 2020;11(June):1–13.
- Dustin ML. Complement receptors in myeloid cell adhesion and phagocytosis. Microbiol Spectr. 2016;4(6). doi: 10.1128/microbiolspec.MCHD-0034–2016.
- Norris PAA, Segel GB, Burack WR, Sachs UJ, Lissenberg-Thunnissen SN, Vidarsson G, Bayat B, Cserti-Gazdewich CM, Callum J, Lin Y, et al. FcγRI and FcγRIII on splenic macrophages mediate phagocytosis of anti-glycoprotein IIb/IIIa autoantibody-opsonized platelets in immune thrombocytopenia. Haematologica. 2021;106(1):250–254. doi: 10.3324/haematol.2020.248385.
- Ho MK, Springer TA. Mac-1 antigen: quantitative expression in macrophage populations and tissues, and immunofluorescent localization in spleen. J Immunol. 1982;128(5):2281 LP–2286.
- Schittenhelm L, Hilkens CM, Morrison VL. β(2) Integrins as regulators of dendritic cell, monocyte, and macrophage function. Front Immunol. 2017;8:1866.
- Prieto J, Eklund A, Patarroyo M. Regulated expression of integrins and other adhesion molecules during differentiation of monocytes into macrophages. Cell Immunol. 1994;156(1):191–211.
- Vo P, Purev E, West KA, McDuffee E, Worthy T, Cook L, Hawks G, Wells B, Shalabi R, Flegel WA, et al. A pilot trial of complement inhibition using eculizumab to overcome platelet transfusion refractoriness in human leukocyte antigen allo-immunized patients. Br J Haematol. 2020;189(3):551–558. doi: 10.1111/bjh.16385.
- Jackman RP, Lee JH, Pei R, Bolgiano D, Lebedeva M, Slichter SJ, Norris PJ. C1q-Binding anti-HLA antibodies do not predict platelet transfusion failure in trial to reduce alloimmunization to platelets study participants. Transfusion. 2016;56(6):1442–1450. doi: 10.1111/trf.13598.
- Saris A, Peyron I, van der Meer PF, Stuge TB, Zwaginga JJ, van Ham SM, ten Brinke A. Storage-Induced platelet apoptosis is a potential risk factor for alloimmunization upon platelet transfusion. Front Immunol. 2018;9(JUN):1–10. doi: 10.3389/fimmu.2018.01251.
- Peerschke EIB, Andemariam B, Yin W, Bussel JB. Complement activation on platelets correlates with a decrease in circulating immature platelets in patients with immune thrombocytopenic purpura. Br J Haematol. 2010;148(4):638–645.
- Dekkers G, Plomp R, Koeleman CAM, Visser R, von Horsten HH, Sandig V, Rispens T, Wuhrer M, Vidarsson G. Multi-Level glyco-engineering techniques to generate IgG with defined Fc-glycans. Sci Rep. 2016;6:1–12. doi: 10.1038/srep36964.
- van Osch TLJ, Oosterhoff JJ, Bentlage AEH, Nouta J, Koeleman CAM, Geerdes DM, Mok JY, Heidt S, Mulder A, Van Esch WJE, et al. Fc galactosylation of anti-platelet hIgg1 alloantibodies enhance complement activation on platelets. Haematologica. 2022. doi: 10.3324/haematol.2021.280493.
- Mulder A, Kardol MJ, Arn JS, Eijsink C, Franke MEI, Schreuder GMT, Haasnoot GW, Doxiadis IIN, Sachs DH, Smith DM, et al. Human monoclonal HLA antibodies reveal interspecies crossreactive swine MHC class I epitopes relevant for xenotransplantation. Mol Immunol. 2010;47(4):809–815. doi: 10.1016/j.molimm.2009.10.004
- Mulder A, Eijsink C, Kester MGD, Franke ME, Kardol MJ, Heemskerk MH, van Kooten C, Verreck FA, Drijfhout JW, Koning F, et al. Impact of peptides on the recognition of HLA class I molecules by human HLA antibodies. J Immunol. 2005;175(9):5950–5957.
- Daga S, Moyse H, Briggs D, Lowe D, Evans N, Jones J, Buchli R, McMurtrey C, Mulder A, Hildebrand W, et al. Human immunology direct quantitative measurement of the kinetics of HLA-specific antibody interactions with isolated HLA proteins. Hum Immunol. 2018;79(2):122–128.
- Duquesnoy RJ, Marrari M, Jelenik L, Zeevi A, Claas FHJ, Mulder A. Structural aspects of HLA class I epitopes reacting with human monoclonal antibodies in Ig-binding, C1q-binding and lymphocytotoxicity assays. Hum Immunol. 2013;74(10):1271–1279. doi: 10.1016/j.humimm.2013.05.016.
- Congy-Jolivet N, Drocourt D, Portet S, Tiraby G, Blancher A. Production and characterization of chimeric anti-HLA monoclonal antibodies targeting public epitopes as tools for standardizations of the anti-HLA antibody detection. J Immunol Methods. 2013;390(1–2):41–51.
- Lo M, Kim HS, Tong RK, Bainbridge TW, Vernes J-M, Zhang Y, Lin YL, Chung S, Dennis MS, Zuchero YJY, et al. Effector-Attenuating substitutions that maintain antibody stability and reduce toxicity in mice. J Biol Chem. 2017;292(9):3900–3908. doi: 10.1074/jbc.M116.767749.
- Falck D, Jansen BC, de Haan N, Wuhrer M. High-Throughput analysis of IgG Fc glycopeptides by LC-MS. Methods Mol Biol. 2017;1503:31–47.
- Temming AR, Bentlage AEH, de Taeye SW, Bosman GP, Lissenberg-Thunnissen SN, Derksen NIL, Brasser G, Mok JY, van Esch WJE, Howie HL, et al. Cross-Reactivity of mouse IgG subclasses to human Fc gamma receptors: antibody deglycosylation only eliminates IgG2b binding. Mol Immunol. 2020;127(March):79–86. doi: 10.1016/j.molimm.2020.08.015.
- Dekkers G, Bentlage AEH, Stegmann TC, Howie HL, Lissenberg-Thunnissen S, Zimring J, Rispens T, Vidarsson G. Affinity of human IgG subclasses to mouse Fc gamma receptors. MAbs. 2017;9(5):767–773. doi: 10.1080/19420862.2017.1323159.
- ten Brinke A, Karsten ML, Dieker MC, Zwaginga JJ, van Ham SM. The clinical grade maturation cocktail monophosphoryl lipid a plus IFNγ generates monocyte-derived dendritic cells with the capacity to migrate and induce Th1 polarization. Vaccine. 2007;25(41):7145–7152.
- Saris A, Tomson B, Brand A, Mulder A, Claas FH, Lorinser J, Scharenberg J, van Ham SM, ten Brinke A, Zwaginga JJ, et al. Platelets from donors with consistently low HLA-B8, -B12, or -B35 expression do not undergo antibody-mediated internalization. Blood. 2018;131(1):144–152. doi: 10.1182/blood-2017-07-799270.
- Vidarsson G, van de Winkel JG. Fc receptor and complement receptor-mediated phagocytosis in host defence. Curr Opin Infect Dis. 1998;11(3):271–278.
- Bruggeman CW, Dekkers G, Bentlage AEH, Treffers LW, Nagelkerke SQ, Lissenberg-Thunnissen S, Koeleman CAM, Wuhrer M, van den Berg TK, Rispens T, et al. Enhanced effector functions due to antibody defucosylation depend on the effector cell Fcγ receptor profile. J Immunol. 2017;199(1):204–211. doi: 10.4049/jimmunol.1700116.
- Nagelkerke SQ, Dekkers G, Kustiawan I, van de Bovenkamp FS, Geissler J, Plomp R, Wuhrer M, Vidarsson G, Rispens T, van den Berg TK, et al. Inhibition of FcγR-mediated phagocytosis by IVIg is independent of IgG-Fc sialylation and FcγRIIb in human macrophages. 2014;124(25):3709–3718. doi: 10.1182/blood-2014-05-576835.
- Walbaum S, Ambrosy B, Schütz P, Bachg AC, Horsthemke M, Leusen JHW, Mócsai A, Hanley PJ. Complement receptor 3 mediates both sinking phagocytosis and phagocytic cup formation via distinct mechanisms. J Biol Chem. 2021;296:100256. doi: 10.1016/j.jbc.2021.100256.