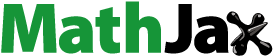
Abstract
Platelet extracellular vesicles (PEVs) are an emerging delivery vehi for anticancer drugs due to their ability to target and remain in the tumor microenvironment. However, there is still a lack of understanding regarding yields, safety, drug loading efficiencies, and efficacy of PEVs. In this study, various methods were compared to generate PEVs from clinical-grade platelets, and their properties were examined as vehicles for doxorubicin (DOX). Sonication and extrusion produced the most PEVs, with means of 496 and 493 PEVs per platelet (PLT), respectively, compared to 145 and 33 by freeze/thaw and incubation, respectively. The PEVs were loaded with DOX through incubation and purified by chromatography. The size and concentration of the PEVs and PEV-DOX were analyzed using dynamic light scattering and nanoparticle tracking analysis. The results showed that the population sizes and concentrations of PEVs and PEV-DOX were in the ranges of 120–150 nm and 1.2–6.2 × 1011 particles/mL for all preparations. The loading of DOX determined using fluorospectrometry was found to be 2.1 × 106, 1.7 × 106, and 0.9 × 106 molecules/EV using freeze/thaw, extrusion, and sonication, respectively. The internalization of PEVs was determined to occur through clathrin-mediated endocytosis. PEV-DOX were more efficiently taken up by MDA-MB-231 breast cancer cells compared to MCF7/ADR breast cancer cells and NIH/3T3 cells. DOX-PEVs showed higher anticancer activity against MDA-MB-231 cells than against MCF7/ADR or NIH/3T3 cells and better than acommercial liposomal DOX formulation. In conclusion, this study demonstrates that PEVs generated by PLTs using extrusion, freeze/thaw, or sonication can efficiently load DOX and kill breast cancer cells, providing a promising strategy for further evaluation in preclinical animal models. The study findings suggest that sonication and extrusion are the most efficient methods to generate PEVs and that PEVs loaded with DOX exhibit significant anticancer activity against MDA-MB-231 breast cancer cells.
Plain Language Summary
What is the context?
● Current synthetic drug delivery systems can have limitations and side effects.
● Platelet extracellular vesicles (PEVs) are a natural and potentially safer alternative for delivering cancer drugs to tumors.
● However, there is still a lack of understanding about how to produce PEVs and how effective they are in delivering drugs.
What is new?
● We compared different methods for producing PEVs from clinical-grade platelets and found that sonication and extrusion were the most effective methods.
● The PEVs were loaded with a cancer drug called doxorubicin (DOX) and tested their ability to kill breast cancer cells.
What is the impact?
● PEVs loaded with DOX were effective at killing cancer cells, especially MDA-MB-231 breast cancer cells.
● This study demonstrates that PEVs are a promising strategy for delivering cancer drugs to tumors and that sonication and extrusion are the most efficient methods for producing PEVs.
● The results suggest that further evaluation of PEVs in preclinical animal models is warranted to determine their potential as a cancer drug delivery system.
Abbreviations: ADP: adenosine diphosphate; bFGF: basic fibroblast growth factor; BSA: bovine serum albumin; CD41: platelet glycoprotein IIb; CD62P: P-selectin; CFDASE: 5-(and-6)-carboxyfluorescein diacetate: succinimidyl ester; CPLT: cryopreserved platelet; CPZ: chlorpromazine hydrochloride; CTC: circulating tumor cell; DMSO: dimethyl sulfoxide; DDS: drug delivery system; DOX: doxorubicin; EPR: enhanced permeability and retention; EV: extracellular vesicle; FBS: fetal bovine serum; GMP: good manufacturing practice; GF: growth factor; HER2: human epidermal growth factor receptor 2; HGF: hepatocyte growth factor; Lipo-DOX: liposomal doxorubicin; MDR: multi-drug resistance; MMP-2: matrix metalloproteinase-2; MP: microparticle; MSC: mesenchymal stromal cell; NP: nanoparticle; NTA: nanoparticle tracking analysis; PAR-1: protease activated receptor-1; PAS: platelet additive solution; PBS: phosphate-buffered saline; PC: platelet concentrate; PEG: polyethylene glycol; PEV: platelet-derived extracellular vesicle; DOX-PEV: doxorubicin-loaded platelet-derived extracellular vesicle-encapsulated; PFA: paraformaldehyde; PF4: platelet factor 4; P-gp: P-glycoprotein; PLT: platelet; PS: phosphatidylserine; SDS-PAGE: sodium dodecylsulfate polyacrylamide gel electrophoresis; SEM: scanning electron microscopy; TCIPA: tumor cell-induced PLT aggregation; TDDS: targeted drug delivery system; TEG: thromboelastography; TF: tissue factor; TF-EV: extracellular vesicle expressing tissue factor; TME: tumor microenvironment; TNBC: triple-negative breast cancer; TXA2: thromboxane-A2; VEGF: vascular endothelial growth factor; WHO: World Health Organization.
Introduction
There is growing interest in engineering cells, such as stem cells, macrophages, and red blood cells, to serve as translational alternatives to synthetic carriers for drug delivery.Citation1 Cells offer significant advantages as drug delivery systems (DDSs) for clinical translation, including biocompatibility, long residence times, and low immunogenicity, although their handling under conditions meeting good manufacturing practices (GMPs) can be complex.Citation1–3 Although platelets (PLTs) have interesting features, only a relatively limited number of studies have considered them as DDSs. The collection infrastructure of allogeneic human PLT concentrates (PCs) is well established in most countries, providing a readily available cellular source meeting quality and safety criteria for transfusion. Additionally, plateletpheresis collection procedures can readily be transposed to obtain autologous PLTs from patients. Using licensed PCs as source materials and approved collection procedures can thus facilitate the repositioning of PLTs as source materials for preparing clinical-grade DDSs.
In addition to their role in hemostasis, PLTs can, through expressions of multiple membrane markers, play, in particular, a unique role as functional and targeting vectors of anticancer agents.Citation4–7 These membrane markers include GPIIb (cluster of differentiation 41 (CD41)), GPIIIa (CD61), GPIaIIa (CD49b/CD29), GPIba (CD42b), P-selectin (CD62), and activated platelets also expose phosphatidylserine (PS) phospholipid.Citation8 The rationale for such clinical applications is reinforced by the fact that PLTs are anucleated, making them ideal as “Trojan horse” cargoes of nucleotoxic compounds as they will not undergo drug-induced apoptosis.
A few previous studies have demonstrated that PLTs can be loaded with doxorubicin (DOX) and, using various cellular and animal models, that DOX-loaded PLTs indeed provide targeting and enhanced killing of cancer cells.Citation9–12 Furthermore, when exposed to cancer cells and, in particular, to released tissue factor (TF), PLTs excrete extracellular vesicles (EVs; PEVs) of approximately 100–200 nm in size.Citation9 Studies have demonstrated that PEVs, once released, can specifically contribute to the regulation of cancer progression in vivo.Citation13 This is due to their ability to interact with, and be internalized by, target cells within the tumor microenvironment, including cancer cells.Citation13 This recent observation led us to hypothesize not only that DOX-loaded PLTs could release DOX-loaded PEVs in contact which cancer cells, which we have already demonstrated recently,Citation9 but also that DOX-loaded PEVs could be used as a potent stand-alone DDS of anticancer drugs.
Provided that a biomedical engineering approach can be developed to efficiently generate and load PEVs, these biological subcellular PLT structures should offer several practical advantages as DDSs. For instance, their nanosize should confer a higher capacity to penetrate and be retained within the tumor microenvironment (TME). Additionally, PEVs should express the various PLT membrane proteins and phosphatidylserine (PS) that can support interactions and internalization by cancer cells. Moreover, in contrast to PLTs, which are 2 ~ 4 um in size, PEVs have the potential to withstand sterile filtration on a 0.2-µm filter for bacterial sterility, facilitating the manufacturing processCitation14 and its compliance with GMP.
However, optimal conditions for generating PEVs and loading them with anticancer drugs using scalable procedures have not been proposed. We report here extensive studies comparing various approaches to generate and characterize PEVs and load them with DOX. We investigated the potential of various breast cancer cell lines to internalize such DOX-loaded PEVs and compared their cytotoxic effects to those of free DOX and Doxil®, a liposomal formulation that has been approved by the US Food and Drug Administration (FDA).Citation15 Breast cancer was used as a model system, as it is one of the leading causes of mortality among women worldwide.Citation16 Triple-negative breast cancer (TNBC) and multidrug resistance (MDR) present significant challenges for breast cancer treatment due to their high incidence, poor prognosis, and inadequate therapeutic options.Citation17 TNBC is characterized by a lack of estrogen, progesterone, and human epidermal growth factor receptor 2 (HER2) protein receptors, leading to inadequate responses to hormone and anti-HER2 monoclonal antibody therapy.Citation16 MDR creates obstacles to chemotherapy involving the anticancer drugs, taxol, and DOX, due to drug efflux pumping, such as by P-glycoprotein (P-gp).Citation18 Therefore, developing a safe and intelligent DDS is critical for treating refractory breast cancer. Our hypothesis was that DOX-loaded PEVs would demonstrate superior targeting capabilities for MDR breast cancer and TNBC cells (MCF7/ADR and MDA-MB-231) when compared to the free drug or liposomal formulation.Citation19,Citation20
Materials and methods
Study design
The overall study design is depicted in .
Figure 1. Experimental design: platelets (PLTs) isolated from clinical-grade PLT concentrates were subjected to sonication, freezing/thawing, extrusion, and incubation to generate extracellular vesicles (PEVs) that were incubated with and loaded with doxorubicin (DOX). Free DOX was eliminated by size-exclusion chromatography, and the DOX-loaded PEVs (DOX-PEVs) were characterized.

Preparation of cryopreserved platelets (CPLTs).
This study was approved by the Institutional Review Board of Taipei Medical University (TMU-JIRB no.: 201905060). PCs (N = 50) were collected from volunteer donors at the Taipei Blood Center (Taiwan Blood Services Foundation, Guandu, Taiwan) using an apheresis procedure (MCS+ cell separators, Haemonetics, Braintree, MA, USA).Citation9 PCs were delivered to our laboratory within 5 days of collection and processed the same day. PCs were centrifuged at 1500 ×g for 10 min to pelletize the PLTs. PLTs were suspended in platelet additive solution (PAS) containing 6% dimethyl sulfoxide (DMSO) and kept frozen at −80°C, as in our previous research.Citation9 Blood cell counts (white blood cells, red blood cells, and PLTs) were quantified by ABC Vet (ABX Diagnostics, Montpellier, France).
Preparation of PLT EVs (PEVs).
Four types of PEVs were prepared. PLTs were thawed at 37°C for 5 min, washed with 0.1 µm (Pall, New York, NY, USA) filtered Dulbecco’s phosphate-buffered saline (DPBS, HyClone, Logan, UT, USA), and resuspended at a concentration of approximately 1.42 × 109 PLTs/mL in DPBS. Suspended PLTs (20 mL) were aliquoted into 50-mL centrifuge tubes and subjected to four types of treatments to generate PEVs. Extrusion: PLT suspensions were extruded 11 times using an Avanti mini-extruder (Avanti Polar Lipids, Alabaster, AL, USA) and 1000-, 800-, and 400-nm polycarbonate membrane filters. Freeze-thaw: PLT suspensions were subjected to three cycles of freezing at −80°C for 1 h and thawing at 37°C for 10 min. Sonication: PLT suspensions were sonicated for 10 cycles of 30 s on/off at 20 kHz using a UP-80 ultrasonic processor (ChromTech, Taipei, Taiwan) on ice for a total of 5 min. Incubation: Suspensions were incubated at 25°C for 1 h. The respective suspensions were then centrifuged sequentially at 3000 and 104 ×g to remove any cells and cell debris, and the supernatants were sterile-filtered through 0.22-μm filters (Pall). The suspensions were aliquoted and frozen at −80°C until further analysis.
Loading of DOX in isolated PEVs (DOX-PEVs).
PEVs (1 mL approximately 2 × 1011 EVs) generated by incubation, extrusion, freeze/thawing, and sonication were incubated with 1 mL of 200 µM DOX in DPBS at 37°C for 24 h on a suspension mixer (SM-3000; Yihder, Taipei, Taiwan). One and a half milliliters of each mixture was passed through 5 mL of prepacked Sephadex G-25 resin (GE Healthcare, Uppsala, Sweden) equilibrated in DPBS to separate DOX-PEVs from unloaded DOX. Flow-through fractions of 500 µL each were collected. Fractions 4 to 6 (1.5 mL) that contained DOX-PEVs were collected.
Characterization of DOX-PEVs
DOX loading efficiency
One hundred microliter aliquots of DOX-PEVs isolated by Sephadex G-25 chromatography were lysed with 100 µL of 5% sodium dodecylsulfate (SDS; Sigma-Aldrich, St. Louis, MO, USA) and vortexed for 1 min. The calibration curve for DOX was prepared in 5% SDS at concentrations of 0.78, 1.56, 3.13, 6.25, 12.5, 25, 50, and 100 µM. The concentration of loaded DOX in the PEVs was measured by a fluorospectrometer (Thermo Electron, Vantaa, Finland) at excitation and emission wavelengths of 470 and 585 nm, respectively. The loading efficiency (EE%) of DOX in PEVs was calculated by the following equation:
Scanning electron microscopy
Degreased 16-mm coverslips (Marienfeld, Lauda-Königshofen, Germany) were coated with a CD41 antibody (1:1000, Clone: EPR4330, Abcam, Cambridge, MA, USA) for 1 h at 23 ± 2°C. Two hundred microliters of PEVs and DOX-PEVs were loaded on the coverslips at 23 ± 2°C and incubated for 1 h. Adherent PEVs were prefixed with 4% paraformaldehyde (PFA; Fluka, Buchs, Switzerland), followed by post-fixation with 2% PFA (Fluka) and 2.5% glutaraldehyde (Sigma-Aldrich) in 0.2 M cacodylate (Sigma-Aldrich) for 30 min. Fixed PEVs were dehydrated using ethanol gradients (70%~100%) at 23 ± 2°C, and gold-coated. The morphology of isolated PEVs was observed by scanning electron microscopy (SEM, SU3500, Hitachi, Tokyo, Japan).
Dynamic light scattering
Aliquots (0.8 mL) of PEVs and DOX-PEVs were loaded into folded capillary cells (Malvern Instrument, Worcestershire, UK). The size distribution and zeta potential were determined by a Zetasizer Nano ZSP instrument equipped with a 10-mW He-Ne laser at a wavelength of 633 nm and 25°C (Malvern Instrument).
Nanoparticle tracking analysis (NTA)
The number and size distribution profile of PEVs and DOX-PEVs were measured by an NTA equipped with a 488-nm laser (Nanosight NS300, Malvern Instruments). PEVs and DOX-PEVs were diluted with 0.1 µm-filtered DPBS, which was also used as a negative control. The camera level was set to 16 and an analysis detection threshold of 5. Three repeated 60-s measurements were recorded and analyzed with NTA 3.4 software.
Western blotting
Protein concentrations of PEVs and DOX-PEVs were determined using a Pierce BCA protein assay kit (ThermoFisher Scientific). Ten micrograms of proteins was heated at 70°C for 10 min and loaded onto a Precast SurePAGE 4–12% Bis-Tris protein gel (GenScript, Nanjing, China). Proteins were transferred to polyvinylidene difluoride membranes (Pall, Port Washington, NY, USA), and blocked using 5% bovine serum albumin (BSA; Sigma-Aldrich). Membranes were incubated with antibodies against platelet markers CD41 (1:1000, Clone: EPR4330, Abcam, Cambridge, MA, USA) and CD62P (1:108, Clone: AK-6, Abcam), and EV markers CD9 (1:200, Clone: C-4, Santa Cruz Biotechnology, Santa Cruz, CA, USA) and CD63 (1:200, Clone: MX-49.129.5, Santa Cruz). Horseradish peroxidase-conjugated antibodies (1:2000, GeneTex, Irvine, CA, USA) were used as a secondary antibody. Detection was done by enhanced chemiluminescence (ECL) (GE Healthcare). Bands were analyzed on a BioSpectrum 810 Imaging System (Analytik Jena, Jena, Germany).
Phosphatidylserine (PS) expression
PS expression on PEV surfaces is associated with procoagulant activity that was measured by a Zymuphen microparticle (MP)-activity capture assay (Hyphen BioMed, Paris, France) following the supplier’s instructions. Briefly, 100 µL of isolated PEVs (2 × 1010), plasma control high (“RC high”) and plasma control low (“RC low”) was diluted with sample diluent and added to an Annexin V-coated 96-well plate and incubated at 37 ± 1°C for 1 h. FVa-FXa complex (at 100 µL) with calcium and 50 μL of prothrombin were added to the plate and incubated at 37 ± 1°C for 10 min. A thrombin substrate (50 μL) was added to generate thrombin based on the concentration of the phospholipids present. The intensity of the absorbance was measured at 405 nm after adding 50 μL of 2% citric acid.
Cellular assays
Cell lines
NIH/3T3, an embryonic fibroblast cell line (a gift from Dr. Lu Long-Sheng, Taipei Medical University (TMU)) was maintained in modified minimum essential medium (MEM) alpha (HyClone, Logan, UT, USA) supplemented with 10% fetal bovine serum (FBS, Gibco, ThermoFisher Scientific, Waltham, MA, USA). MDA-MB-231, a TNBC cell line (a gift from Dr. Deng Win-Ping, TMU) and MCF7/ADR, a DOX-resistant breast cancer cell line (a gift from Dr. Mi Fwu-Long, TMU) were grown in Dulbecco’s modified Eagle medium (DMEM, HyClone) supplemented with 10% FBS (Gibco). All cells were incubated at 37 ± 1°C in a CO2 incubator (Model 370, ThermoFisher Scientific).
PEV uptake analysis by flow cytometry
Isolated PEVs were labeled with the fluorescent dye 5-(and-6)-carboxyfluorescein diacetate, succinimidyl ester (CFDA-SE; Biotium, Fremont, CA, USA) following the manufacturer’s instructions. Briefly, 10 µL of 4000 µM CFDA-SE was mixed with 2 mL of PEVs (ca. 2 × 1011 PEVs/mL) and incubated at 37 ± 1°C for 2 h on a suspension mixer (Yihder). Excess dye was removed by passing 1.5 mL of the suspension through 5-mL Sephadex G-25 chromatography (Sigma-Aldrich). Flow-through fractions 4 to 6 (0.5 mL each) were pooled for further analysis. CFDA-SE-labeled PEVs (2 × 1011, based on NTA quantification) were added to 12-well plates seeded with 4 × 105 MDA-MB-231, MCF7/ADR, or NIH/3T3 cells and incubated for 1, 3, 6, and 12 h at 37 ± 1°C in an incubator. Cells were detached with 0.05% trypsin-EDTA (ThermoFisher Scientific) and washed with DPBS (HyClone) twice. Cell pellets were resuspended with 100 µL of a 500-fold diluted Zombie violet fixable viability kit (Biolegend, San Diego, CA, USA) and incubated for 10 min at 23 ± 2°C. Then, 900 µL of DPBS was added to wash the cells, followed by centrifugation at 300 ×g for 3 min at 23 ± 1°C. Cell pellets were resuspended with 500 µL of DPBS. Unstained cells were used as a negative control, and 5 µM of CFDA-SE was used as a positive control. Viable cells were gated at excitation and emission wavelengths of 405 and 440 ± 25 nm, respectively. The cellular uptake of CFDA-SE-labeled PEVs was determined by the mean fluorescence intensity (MFI) at excitation and emission wavelengths of 488 and 530 ± 15 nm, respectively, using an Attune™ NxT Acoustic Focusing Cytometer (ThermoFisher Scientific, Carlsbad, CA, USA).
PEV uptake mechanism
Chlorpromazine hydrochloride (CPZ, Sigma-Aldrich) an endocytosis inhibitor, was dissolved in DMSO at 30 mg/mL (84.4 mM). Then, 84.4 mM CPZ was diluted in the cellular medium to get final concentration of CPZ at 25 µM. CPZ was added to pretreat cells for 30 min. CFDA-SE-PEVs (at 2 × 1011) were incubated with 4 × 105 MDA-MB-231, MCF7/ADR, or NIH/3T3 cells for 3 h at 37 ± 1°C in an incubator. Medium was used as a negative control, and 5 µM of CFDA-SE was used as a positive control. The residual dose of DMSO (0.03%) to solubilize CPZ is nontoxic to human cancer cell lines such as MCF-7 and MDA-MB-231,Citation21 as was confirmed in our preliminary tests. The cellular uptake was analyzed by flow cytometry as described above.
In vitro cytotoxicity of DOX-PEVs on breast cancer cells.
Four thousand MDA-MB-231, MCF7/ADR, and NIH/3T3 cells (100 µl) were seeded in 96-well plates overnight and subsequently treated with three types of PEVs and DOX-PEVs, DOX, and Lipo-DOX (TTY Biopharm, Taipei, Taiwan). Triton X-100 (0.1%)-treated cells were used as a positive control, and untreated cells were used as a negative control. After 72 h of incubation, the in vitro cytotoxicity was assessed by a Cell Counting Kit-8 (CCK-8; Sigma-Aldrich) assay. The CCK-8 solution was added to each well and incubated for 3 h at 37 ± 1°C, and the absorbance of each well was read at 450 and 690 nm as reference wavelengths in a spectrometer. Determination of the number of viable cells in cytotoxicity assays was done using the formula:
Cell viability (%) = [(absorbance of the experimental well - absorbance of blank wells)/(absorbance of control wells - absorbance of blank wells)] × 100
Statistical analysis
All tests were done in at least three independent experiments using different batches of PEV-DOX. The experimental data are expressed as the mean ± SD. Statistical analyses were performed using GraphPad Prism 6.0 software with a one-way ANOVA for DLS, NTA, DOX loading capacity analysis, and MP activity assay; a two-way ANOVA was used for cellular uptake of PEVs and cytotoxicity of DOX-PEV. A p value of ≤ 0.05 was considered to indicate a significant difference (* p ≤ .05, ** p ≤ .01, *** p ≤ .001, **** p ≤ .0001, non-significant=ns, p > .05).
Results
Isolation and characterization of PEVs.
PEVs were generated from CPLTs using four methods: extrusion, three freeze/thaw cycles, sonication, and incubation, followed by isolation from the starting PLTs using centrifugation and 0.22-µm filtration, and were characterized as depicted in .
The morphologies of PEVs in the different preparations were similar, with a spheroid shape as observed by SEM (). Population sizes ranged from ca. 120 to 150 nm by DLS (incubation: 149.8 ± 3.1 nm > extrusion: 136.7 ± 2 nm > freeze/thaw: 126.8 ± 2.4 nm > sonication: 124.3 ± 4.8 nm; ). All PEV preparations displayed a non-significantly different negative zeta potential of between −11 and −12 mV under a physiologically neutral pH condition (incubation: −11.4 ± 1.1 mV, extrusion: −12.2 ± 0.7 mV, freeze/thaw: −12.1 ± 0.8 mV, sonication: −12.3 ± 1; ). The number of filter-sterilized PEVs generated per milliliter as quantified by the NTA was higher when using extrusion ((7.01 ± 2.13) × 1011) or sonication ((7.04 ± 0.96) × 1011) than freeze/thaw ((2.06 ± 0.11) × 1011) or incubation ((0.46 ± 0.18) × 1011) (), corresponding to yields of approximately 493, 496, 145, and 33 PEVs/PLT, respectively.
Figure 2. Isolation and characterization of platelet extracellular vesicles (PEVs). (a) Scanning electron microscopy (SEM) images of each isolated PEV. The white arrows point to the isolated PEVs. (b) the z-average size and (c) zeta potential of the PEVs were measured by dynamic light scattering (DLS). (d) Concentrations of PEVs were measured by a nanoparticle tracking analysis (NTA). Measurements in Fig 2b, 2c, and 2d are from a population of PEVs. Data are presented as the mean ± SD (n = 3). DLS, zeta potential, and NTA data were analyzed by a one-way ANOVA with Tukey’s multiple-comparison test using GraphPad prism 6.0 software. Data are presented as the mean ± SD (n = 3). *p ≤ .05, **p ≤ .01, ***p ≤ .001, ****p ≤ .0001, ns. p > .05.

Thus, all four methods could generate PEVs, but production yields were substantially higher (close to 500 PEVs/PLT) by extrusion or sonication. The 0.22-μm sterilizing filtration of isolated PEVs for storage was performed before further drug-loading steps. The three modes of PEV generation that provided the highest yields (extrusion, sonication, and freeze/thaw) were used to evaluate further drug-loading procedures.
Isolation and characterization of PEVs and DOX-loaded PEVs (DOX-PEVs).
We then evaluated the capacity of these three selected populations of PEVs to be loaded with DOX using incubation at 37°C for 24 h under mild mixing. Any residual unloaded DOX was removed by Sephadex G-25 chromatography. Population sizes of purified DOX-PEVs ranged from ca. 130 to 140 nm by DLS (extrusion: 138.5 ± 1.8 nm; freeze/thaw 135.2 ± 1.4 nm; sonication 131.3 ± 0.7 nm; ). The size distribution of DOX-PEVs obtained by extrusion, and that of the PEV controls (120 to 140 nm) that were processed in the same way using DLS indicated that DOX loading and Sephadex G-25 chromatography did not significantly affect population sizes. Sizes of DOX-PEVs obtained by sonication and freeze/thaw were slightly larger than that of unloaded PEVs (p ≤.05). The zeta potential of DOX-PEVs was between −12 and −13 mV (extrusion: −13 ± 0.2 mV, freeze/thaw: −12.4 ± 1.4 mV, sonication: −13.7 ± 2.5 mV; ), similar to that of unloaded PEVs.
Figure 3. Isolation and characterization of doxorubicin (DOX)-platelet (PLT) extracellular vesicles (EVs; PEVs). (a) Size distribution and (b) zeta potential of DOX-PEVs measured by dynamic light scattering (DLS). (c) Quantity of DOX molecules loaded per PEV measured by fluorospectrometry. (d) Protein expression of platelet markers (Cd41a and CD62P) and EV markers (CD9 and CD63) of each unloaded and loaded PEV analyzed by Western blotting. Data are presented as the mean ± SD (n = 3). The size distribution, zeta potential, DOX quantification, and protein concentration data were analyzed by a one-way ANOVA with Tukey’s test using GraphPad Prism 6 software. *p ≤ .05, **p ≤ .01, ****p ≤ .0001, ns. p > .05. Abbreviations: NTA: nanoparticle tracking analysis.

We then determined the concentration of DOX in DOX-PEVs by fluorospectrometry. These values corresponded to (2.1 ± 0.2) × 106 (freeze-thaw), (1.7 ± 0.3) × 106 (extrusion), and (0.9 ± 0.2) × 106 (sonication) DOX molecules per EV (). The Western blot analysis () revealed that expressions of PEV surface markers of CD41, CD62P, CD9, and CD63 were similar for all types of DOX-PEVs and PEVs supporting the lack of a detrimental impact by the drug-loading procedure.
Procoagulant activity of PEVs.
We measured the procoagulant activity of PEV preparations using the MP activity kit. This kit determines the functional procoagulant activity of PS exposed on the surface of PEVs isolated by this capture assay (). PEVs generated by freeze-thaw demonstrated approximately 3 times less PS-associated procoagulant activity (390.6 ± 49 nM) than those obtained via extrusion (960.7 ± 32.8 nM) or sonication (1131.25 ± 131.6 nM). There was no significant difference (p > .05) between the procoagulant activities of PEVs produced by extrusion and sonication. Therefore, these data suggest that PEVs generated by freeze-thaw would be less prothrombogenic in vivo than those obtained by extrusion or sonication.
Figure 4. Procoagulant activity of platelet EVs (PEVs) treated with extrusion, freeze/thaw, and sonication. Data are presented as the mean ± SD (n = 3). Differences between PEVs were analyzed by a one-way ANOVA with Tukey’s multiple comparisons using GraphPad prism 6.0 software, *** p < .001, **** p < .0001, ns. p > .05.

PEV cellular uptake by flow cytometry.
To determine the efficiency of EV internalization by the two types of breast cancer cell lines (MDA-MB-231 and MCF7/ADR) and one normal cell line (NIH/3T3), three populations of PEVs were labeled with CFDA-SE (CFDA-SE-PEVs) and incubated with the cell lines for 1, 3, 6, and 12 h. The MFIs of CFDA-SE-PEVs at 1, 3, 6, and 12 h were analyzed by flow cytometry to determine the extent of PEV uptake by each cell type. Histograms (Fig. S1) and MFIs () of the three PEV populations were significantly higher in MDA-MB-231 and MCF7/ADR cells than the NIH/3T3 cell line. Flow cytometric data showed that PEVs obtained by extrusion and sonication had stronger internalization by breast cancer cell lines than the embryonic fibroblast cell line.
Figure 5. Internalization of isolated platelet extracellular vesicles (PEVs) in MDA-MB-231, MCF7/ADR, and NIH/3T3 cells as analyzed by flow cytometry. The mean fluorescence intensities (MFIs) of CFDASE-PEVs internalized by (b) MDA-MB-231, (c) MCF7/ADR, and (d) NIH/3T3 cells for 1, 3, 6, and 12 h. Data are presented as the mean ± SD (n = 3). MFI data were analyzed by a two-way ANOVA with Tukey’s multiple-comparisons test using GraphPad prism 6.0 software. *p ≤ .05, **p ≤ .01, ***p ≤ .001, ****p ≤ .0001, ns. p > .05.

Furthermore, the FCM histogram () and MFIs () of CFDASE-PEVs after treatment with CPZ, a clathrin-mediated endocytosis inhibitor, were significantly lower than those of untreated cells, indicating cell uptake of PEVs based on the clathrin-mediated endocytosis pathway.
Figure 6. The mechanism of 5-(and-6)-carboxyfluorescein diacetate, succinimidyl ester-labeled (CFDASE)-platelet extracellular vesicles (PEVs) in MDA-MB-231, MCF7/ADR, and NIH/3T3 cells as analyzed by flow cytometry. (a) Flow cytometric histograms of cellular uptake inhibition of CFDASE-PEVs pretreated without chlorpromazine hydrochloride (CPZ) or with CPZ for 30 min following by incubation with MDA-MB-231, MCF7/ADR, or NIH/3T3 cells for 3 h. The mean fluorescence intensities (MFIs) of CFDASE-PEVs internalized by (b) MDA-MB-231 cells, (c) MCF7/ADR and (d) NIH/3T3 cells. The flow cytometric results indicated that PEV cellular uptake was inhibited by CPZ. Data are presented as the mean ± SD (n = 3). MFI data were analyzed by a two-way ANOVA with Sidak’s multiple-comparisons test using GraphPad prism 6.0 software. **p ≤ .01, ***p ≤ .001, ****p ≤ .0001.

Cytotoxicity effects of DOX-PEVs toward breast cancer cells.
We further examined whether the intensity of cellular uptake impacted the cytotoxicity. The effects of the three types of DOX-PEVs on breast cancer cell lines were assessed by a CCK-8 assay. Viabilities of the MDA-MB-231 and MCF7/ADR breast cancer cell lines and NIH/3T3 embryonic fibroblast cell line exposed to the three types of DOX-PEVs, free DOX, and liposomal (Lipo)-DOX were compared to that of untreated cells (negative control; data not shown) and 0.1% Triton-X 100-treated cells (positive control; data not shown) for 72 h. The cytotoxic effects of the three types of DOX-PEVs on MDA-MB-231 cells, compared to that of Lipo-DOX, increased at DOX concentrations between 0.05 and 1 µM ().
Figure 7. In vitro cytotoxicity of doxorubicin (DOX)-loaded-platelet extracellular vesicles (PEVs) on cancer cell lines. (a) MDA-MB-231, (b) MCF7/ADR, and (c) NIH/3T3 cell lines were grown for 72 h in the presence of extrusion DOX-PEVs, freeze/thawed DOX-PEVs, sonicated DOX-PEVs, liposomal (Lipo)-DOX, and DOX. Cell viability was assessed with a CCK-8 assay. Data are presented as the mean ± SD (n = 3). The cytotoxicity of DOX-PEVs was analyzed by a two-way ANOVA with Tukey’s multiple-comparison test using GraphPad prism 6.0 software. *p < .05, **p < .01, ***p < .001, ****p < .0001; * compared to the Lipo-DOX group; # compared to the DOX group; @ compared among the three PEV-DOX groups.

Thus, DOX-PEVs were more cytotoxic to the MDA-MB-231 breast cancer cell line than was Lipo-DOX. As expected, the DOX-resistant MCF7/ADR cell line was more resistant to free DOX and Lipo-DOX compared to the other cell lines. Interestingly, the cytotoxic effect on MCF7/ADR cells by DOX-PEVs was significantly higher than that of free DOX at DOX concentrations of 0.05 ~ 0.5 µM () and Lipo-DOX. In addition, DOX-PEVs were less cytotoxic to the NIH/3T3 embryonic fibroblast cell line than was free DOX at DOX concentrations of 0.1 ~ 1 µM (). The results indicated that DOX-PEVs provided more-selective delivery of DOX to breast cancer cell lines compared to a normal cell line.
Discussion
Research has shown that PEVs can play a unique role in cancer progression due to their ability to interact with, and be internalized by, cells in the tumor microenvironment, including cancer cells.Citation13 This led us to hypothesize that PEVs might serve as a dedicated vehicle for the delivery of anti-cancer drugs, provided that a suitable manufacturing process can be developed using clinical-grade allogeneic PC or autologous PC as starting source material. Consequently, in this study, we investigated the feasibility of generating PEVs from PLTs isolated from clinical-grade human PCs, for use as a DDS for DOX, an anticancer drug commonly used to treat breast cancer. To facilitate scaling-up and translational applications, we developed a freeze-thaw protocol using 6% DMSO for long-term storage and potential pooling of isolated PLTs. While previous studies have shown that thawed CPLTs can release PEVs with hemostatic activity for transfusions,Citation14,Citation22 their potential as a DDS has not yet been explored to our knowledge. Moreover, we demonstrate that both naive and loaded PEVs generated from thawed PLTs can be microfiltered for bacterial safety.
The lack of standardized processed and qualification assessments for EV-based DDSs has made GMP-grade production challenging, as noted in previous analyses.Citation23 To standardize our manufacturing approaches for PEVs, we used pooled CPLTs as starting material to limit batch-to-batch variability. We evaluated various procedures for PEV generation and performed quality and quantity control analyses of PEV production and the DOX-loading procedure. To increase yields, some physical methods such as extrusionCitation24 and sonication,Citation25 have been used to generate EVs. Recent studies have shown that EV production yields from monocytes and macrophages by extrusion, which maintain surface markers for targeting efficiency, can be 100 ~ 250-fold higher than those naturally released from cells.Citation26–28
We evaluated several physical methods to generate PEVs for DDSs. While previous studies have shown that macrophages and mesenchymal stromal cells (MSCs) can generate EVs when exposed to physical methods such as extrusionCitation26–29 and sonication,Citation25 little is known about the optimal procedures to stimulate PLTs to form EV for use as DDSs.Citation30 To stimulate PEV production, we treated CPLTs with extrusion, sonication, and freeze/thawing and compared PEV yields to a control group in which PLTs were incubated at 37°C without physical stress. Our data showed that PEVs could readily be generated from PLTs isolated from clinical-grade PCs, with yields of up to 500 EVs or EV-like events per PLT using extrusion and sonication, compared to close to 150 using freeze/thawing and only about 30 during incubation. These numbers are higher than those generated by calcium ionophore activation,Citation31 which yielded 57 PEVs. In comparison, EV numbers generated from human umbilical cord MSCs treated by sonication were 20-fold higher than those generated by spontaneous secretion.Citation25 Few studies have compared PEV generation methods from PLTs so far. However, it is believed that PEVs can be generated by physical means such as sonication, electroporation, extrusion, and freeze/thaw cycles, or by chemical stimulation using thrombin, adenosine diphosphate (ADP), lipopolysaccharide, Ca2+ ionophores, and collagens.Citation31
Our study also suggests that loading DOX into isolated PEVs is more efficient than loading it into PLTs prior to PEV generation. Indeed, our previous data have shown that 15 ~ 36 × 106 molecules of DOX can be loaded in one PLT (approximately. 3 µm) obtained 3 to 9 days after collection.Citation9 However, our current data show that 2 × 106 molecules of DOX are loaded into a single PEV, which measures about 100–150 nm, a size 20–30 times smaller than that of a PLT. Therefore, post-loading DOX in PEVs proves to be a more efficient process than loading PLT with DOX first and then generating PEV-DOX.
In our study, we made a significant finding that the sizes of PEVs generated from various methods were consistently in the range of 120 ~ 150 nm. Moreover, starting from 1.42 × 109 PLTs/mL, we were able to generate (2 ~ 6) × 1011 PEVs, which is at least equivalent if not better than the yield obtained using PLT agonist activation.Citation32 Our analysis also revealed that PEVs have a negative charge under physiological conditions (pH 7.35 ~ 7.45) likely due at least in part to the abundance of negatively charged phospholipids on their surface.Citation33 This negative charge was further confirmed by measuring the zeta potential of isolated PEVs in DPBS at pH 7.2, which ranged from −11 to −12 mV. This negative surface charge contributes to the stability of PEVs in the blood circulation.Citation34 We also demonstrated that multiple methods can be used to generate PEVs and among them, extrusion and sonication were the most effective, producing up to 500 “mini-PLT structures” from one PLT. According to our calculations, this number is close to the maximum number of PEVs (0.13 µm in diameter) that can mathematically be obtained from one PLT (3 µm in diameter) based on the surface area of a spherical surface area (4Πr2) formula. However, in spite of the consistency in the size detected by DLS and NTA data, one cannot ascertain that all detected events are “intact” PEVs, and not also cell or membrane debris of a similar size.
The excess DOX can be easily removed by size-exclusion Sephadex-G25 chromatography after loading, with a good resolution between the elution of PEV-DOX and free DOX (Fig. S2). This process is not only straightforward but also scalable for this application.Citation35 Furthermore, any residual cell debris can be eliminated by centrifugation and 0.2-µm filtration without any substantial loss of PEVs. Achieving aseptic bacterial filtration with a 0.2-µm filter is crucial for GMP-grade processing and clinical translation, as emphasized before.Citation36
EVs are comprised of an outer lipid bilayer and an inner aqueous core, which can be loaded with both hydrophobic and hydrophilic drugs. Active and passive methods are used for loading drugs into EVs. For instance, DOX was actively loaded into cell-derived EVs using methods such as sonicationCitation37 and electroporation.Citation38 In our study, we observed that after co-incubating 1 mL of 1011 PEVs and 1 mL of 200 µM DOX at 37°C for 24 hours, the loading efficiency of DOX within PEVs was approximately 10%, as determined by spectrofluorometry (data not shown). We also found that PEVs generated through freeze/thawing had a higher loading yield (approximately 2 × 106 DOX molecules) than other types of PEVs (as shown in ).
Studies have shown that PEVs can transfer CD41 integrins or microRNAs to breast cancer cells, demonstrating their targeting capacity toward breast cancer cells.Citation39,Citation40 Importantly, our results indicate that the surface markers of PEVs remained unaltered after drug loading, suggesting that the known cancer cell-targeting capacity of PEVs should be retained. Intriguingly, we also observed that freeze/thaw-treated PEVs had lower procoagulant activity than other types of PEVs (as depicted in ). This is an essential criterion for potential clinical translation since it suggests a lower risk of thrombogenesis associated with this type of PEV.
PLT membrane-coated nanoparticles (NPs) have been employed to target metastatic cancer cells and circulating tumor cells.Citation41–44 Macrophage-derived EVs loaded with anticancer agents exhibited higher cytotoxicity to MDR and TNBC cancer cells than the free drug.Citation19,Citation20 Our findings (illustrated in ), show that MDA-MB-231 cells internalized more PEVs compared to MCF7/ADR breast cancer cells, indicating that MDA-MB-231 cells may be more sensitive to DOX-PEVs prepared by sonication, extrusion, and freeze/thawing than those treated with DOX. The efficiency of drug delivery is dependent on the EV uptake pathway. EVs can transfer drugs while entering cells through either fusion with cytoplasmic membranes or endocytosis.Citation45 Therefore, to fully comprehend the impacts of PEV internalization on potential therapeutic strategies for breast cancer cell lines and understand the impacts of PEV internalization on targeted drug delivery, it is essential to further assess potential therapeutic strategies for breast cancer cell lines. The mechanism of EV uptake by cells primarily involved endocytosis, which may occur via a clathrin-dependent pathway (as shown in ), a caveolin-dependent pathway, a lipid raft-mediated pathway, micropinocytosis, phagocytosis, and membrane fusion, depending on their surface proteins.Citation46
One limitation of our study is the use of the murine NIH/3T3 cell line as a control in a human in vitro system to test human PEV internalization. Indeed, it can be argued that the species difference might explain the reduced internalization of human PEV-DOX and subsequent reduced cytotoxicity observed in murine NIH/3T3 compared to human cancer cell lines. However, the NIH/3T3 cell line is often used as a control for human breast cancer cell lines when studying the uptake of nanoparticles,Citation47 and these cells were also found to internalize nanoparticles more efficiently than e.g. A549 human lung carcinoma cells.Citation48 Another limitation if that our current experimental data cannot discern whether the PEV-DOX specifically target cancer cells in the tumor microenvironment, or whether a more general effect that also affect non-cancer cells may co-exist. Based on our data, we anticipate that there is a preferential and faster internalization of PEV-DOX by cancer cells than by normal cells due to interactions between membrane markers. However, we do not exclude - and even anticipate-, some level of competing internalization by normal cells through passive endocytosis. The comparative rate and extent of internalization of PEV-DOX by cancer cells and normal cells could potentially be best studied using a co-culture system in the presence of PEV-DOX. Yet, such a co-culture system has its own drawbacks: (a) it is still an oversimplification of the tumor environment, (b) systemic factors are lacking, and (c) the selected proportions and distribution of the two cell types could be arbitrary. Despite its limitations, 3D co-culture systems might represent a valuable method to show a preferential uptake of PEV-DOX by cancer cells over normal cells. Due to their interactions with cancer cell membranes, PEVs should demonstrate greater retention within the tumor microenvironment than synthetic nanocarriers, leading to more pronounced cytotoxic effects. Another point to consider is the fate of the platelet granules content during PEV generation. Our study, as designed, cannot exclude the presence of granule-derived trophic factors, including angiogenins such as vascular endothelium growth factor, that could promote- tumor development and progression. Our in vitro data, however, indicate that above a certain dose, the cytotoxic effect of DOX is stronger than any possible tumor progression effect, suggesting that this is not a substantial concern when using such PEVs as delivery vehicle of an anti-cancer agent.
Conclusions
In this study, we demonstrate the technical feasibility of using PEV-DOX as DDSs for cancer treatment. Our results show that DOX, an anticancer drug, can be successfully loaded into PEVs at doses reaching approximately 2 × 106 DOX molecules using an overnight procedure. The sizes of the isolated PEVs (120 ~ 150 nm) are suitable for targeting and retention within the TME due to the enhanced permeability and retention (EPR) effect. We also illustrate the feasibility of various PEV-generating procedures, and we identifying at least two methods capable of generating nearly 500 “mini-platelet” EV structures from a single PLT, a number close to the maximum number of PEVs that can mathematically be obtained from one PLT. Additionally, our data demonstrate that PEVs and DOX-PEVs can undergo sterile filtration and be stored by freezing without inducing a risk of procoagulant activity or altering surface markers, rendering them potentially suitable for clinical translation. Furthermore, we show that DOX-loaded PEVs exhibit higher uptake efficiency and anticancer efficacy in drug-resistant MCF7/ADR breast cancer cells. While it has been evidenced that PEVs may exert their own intrinsic effects on tumor progression, which can be either beneficial or detrimental, due to their interaction with cells in the tumor environment,Citation13 any pathophysiological effects associated with PEVs will be counter-balanced by the local dominant cytotoxic effect of DOX.
Therefore, our work provides compelling evidence that PEVs, prepared from healthy allogeneic donors, can serve as effective DDS for cancer treatment. Given their human cell origins, PEVs carry a low risk of immunogenicity and offer superior biocompatibility and targeting capacity. Additionally, the preparation process is also suitable for the preparation of autologous PEVs. While further studies are necessary to elucidate the mechanism of PEVs uptake by breast cancer cells, our findings suggest that PEVs could represent a promising approach for cancer treatment. Despite the absence of animal data in this work, our results contribute valuable and novel insights with potential implications for clinical developments, and they open new avenues for exploring innovative applications of platelets in human medicine.
Authors contributions
T.B. designed and directed the research project. T.B. and Y.W. developed the experimental methodology and delineated the experimental procedure. Y.W. and D.L. contributed to the data analysis of the experimental results. L.D., O.N., L.B. help for sample preparation. L.L., C.C., and L.L. help with analytical procedure development and technique support. T.B., H.G., and L. L. interpretation of the results; and all authors approved the final manuscript.
Supplemental Material
Download TIFF Image (25.3 MB)Supplemental Material
Download TIFF Image (43.4 MB)Acknowledgments
We express our thanks for the technical support provided by the Core Facility at Taipei Medical University. We acknowledge the blood products supplied by the Taipei Blood Center of Taiwan Blood Services Foundation.
Disclosure statement
No potential conflict of interest was reported by the author(s).
Supplementary material
Supplemental data for this article can be accessed online at https://doi.org/10.1080/09537104.2023.2237134
Additional information
Funding
References
- Yang L, Yang Y, Chen Y, Xu Y, Peng J. Cell-based drug delivery systems and their in vivo fate. Adv Drug Deliv Rev. 2022;187:114394. doi:10.1016/j.addr.2022.114394. Epub 2022/06/20.
- Tan S, Wu T, Zhang D, Zhang Z. Cell or cell membrane-based drug delivery systems. Theranostics. 2015;5(8):863. doi:10.7150/thno.11852.
- Sun Y, Su J, Liu G, Chen J, Zhang X, Zhang R, Jiang M, Qiu M. Advances of blood cell-based drug delivery systems. Eur J Pharm Sci. 2017;96:115–12. doi:10.1016/j.ejps.2016.07.021. Epub 2016/10/28.
- Gay LJ, Felding-Habermann B. Contribution of platelets to tumour metastasis. Nat Rev Cancer. 2011;11(2):123–34. doi:10.1038/nrc3004.
- Franco AT, Ware J. Pathophysiology 2: the role of platelets in cancer biology. Cancer Treat Res. 2019;179:37–54. Epub 2019/07/19.
- Bambace NM, Holmes CE. The platelet contribution to cancer progression. J Thromb Haemost. 2011;9(2):237–49. doi:10.1111/j.1538-7836.2010.04131.x. Epub 2010/11/03.
- Goubran HA, Burnouf T, Radosevic M, El-Ekiaby M. The platelet–cancer loop. Eur J Intern Med. 2013;24(5):393–400. doi:10.1016/j.ejim.2013.01.017. Epub 2013/02/26.
- Perez-Pujol S, Marker PH, Key NS. Platelet microparticles are heterogeneous and highly dependent on the activation mechanism: studies using a new digital flow cytometer. Cytom Part A. 2007;71(1):38–45. doi:10.1002/cyto.a.20354.
- Wu YW, Huang CC, Changou CA, Lu LS, Goubran H, Burnouf T. Clinical-grade cryopreserved doxorubicin-loaded platelets: role of cancer cells and platelet extracellular vesicles activation loop. J Biomed Sci. 2020;27(1):45. doi:10.1186/s12929-020-00633-2. Epub 2020/03/24.
- Xu P, Zuo H, Zhou R, Wang F, Liu X, Ouyang J, Chen B. Doxorubicin-loaded platelets conjugated with anti-CD22 mAbs: a novel targeted delivery system for lymphoma treatment with cardiopulmonary avoidance. Oncotarget. 2017;8(35):58322–37. doi:10.18632/oncotarget.16871. Epub 2017/09/25.
- Xu P, Zuo H, Chen B, Wang R, Ahmed A, Hu Y, Ouyang J. Doxorubicin-loaded platelets as a smart drug delivery system: an improved therapy for lymphoma. Sci Rep. 2017;7(1):42632. doi:10.1038/srep42632. Epub 2017/02/16.
- Li T, Chen T, Chen H, Wang Q, Liu Z, Fang L, Wan M, Mao C, Shen J. Engineered platelet-based micro/nanomotors for cancer therapy. Small. 2021;17(52):e2104912. doi:10.1002/smll.202104912. Epub 2021/11/07.
- Lazar S, Goldfinger LE. Platelets and extracellular vesicles and their cross talk with cancer. Blood. 2021;137(23):3192–200. doi:10.1182/blood.2019004119. Epub 2021/05/04.
- Johnson L, Raynel S, Seghatchian J, Marks DC. Platelet microparticles in cryopreserved platelets: potential mediators of haemostasis. Transfus Apher Sci. 2015;53(2):146–52. doi:10.1016/j.transci.2015.10.011. Epub 2015/11/10.
- Barenholz Y. Doxil® — the first FDA-approved nano-drug: lessons learned. J Control Release. 2012;160(2):117–34. doi:10.1016/j.jconrel.2012.03.020. Epub 2012/04/10.
- Azamjah N, Soltan-Zadeh Y, Zayeri F. Global trend of breast cancer mortality rate: a 25-year study. Asian Pac J Cancer Prev. 2019;20(7):2015–20. doi:10.31557/APJCP.2019.20.7.2015. Epub 2019/07/28.
- Foulkes WD, Smith IE, Reis-Filho JS. Triple-negative breast cancer. N Engl J Med. 2010;363(20):1938–48. doi:10.1056/NEJMra1001389. Epub 2010/11/12.
- Mechetner E, Kyshtoobayeva A, Zonis S, Kim H, Stroup R, Garcia R, Parker RJ, Fruehauf JP. Levels of multidrug resistance (MDR1) P-glycoprotein expression by human breast cancer correlate with in vitro resistance to taxol and doxorubicin. Clin Cancer Res. 1998;4(2):389–98. Epub 1998/05/14.
- Haney MJ, Zhao Y, Jin YS, Li SM, Bago JR, Klyachko NL, Kabanov AV, Batrakova EV. Macrophage-derived extracellular vesicles as drug delivery systems for Triple Negative Breast Cancer (TNBC) therapy. J Neuroimmune Pharmacol. 2020;15(3):487–500. doi:10.1007/s11481-019-09884-9. Epub 2019/11/14.
- Kim MS, Haney MJ, Zhao Y, Mahajan V, Deygen I, Klyachko NL, Inskoe E, Piroyan A, Sokolsky M, Okolie O, et al. Development of exosome-encapsulated paclitaxel to overcome MDR in cancer cells. Nanomedicine. 2016;12(3):655–64. doi:10.1016/j.nano.2015.10.012. Epub 2015/11/21.
- Nguyen ST, Nguyen H-L, Truong KD. Comparative cytotoxic effects of methanol, ethanol and DMSO on human cancer cell lines. Biomed Res Ther. 2020;7(7):3855–9. doi:10.15419/bmrat.v7i7.614.
- Eker I, Yilmaz S, Cetinkaya RA, Pekel A, Unlu A, Gursel O, Yilmaz S, Avcu F, Musabak U, Pekoglu A, et al. Generation of platelet microparticles after cryopreservation of apheresis platelet concentrates contributes to hemostatic activity. Turk J Haematol. 2017;34(1):64–71. doi:10.4274/tjh.2016.0049. Epub 2016/04/21.
- Meng W, He C, Hao Y, Wang L, Li L, Zhu G. Prospects and challenges of extracellular vesicle-based drug delivery system: considering cell source. Drug Deliv. 2020;27(1):585–98. doi:10.1080/10717544.2020.1748758. Epub 2020/04/09.
- Rayamajhi S, Nguyen TDT, Marasini R, Aryal S. Macrophage-derived exosome-mimetic hybrid vesicles for tumor targeted drug delivery. Acta Biomater. 2019;94:482–94. doi:10.1016/j.actbio.2019.05.054. Epub 2019/05/28.
- Wang L, Abhange KK, Wen Y, Chen Y, Xue F, Wang G, Tong J, Zhu C, He X, Wan Y. Preparation of engineered extracellular vesicles derived from human umbilical cord mesenchymal stem cells with ultrasonication for skin rejuvenation. ACS Omega. 2019;4(27):22638–45. doi:10.1021/acsomega.9b03561. Epub 2020/01/08.
- Jang SC, Kim OY, Yoon CM, Choi DS, Roh TY, Park J, Nilsson J, Lotvall J, Kim YK, Gho YS. Bioinspired exosome-mimetic nanovesicles for targeted delivery of chemotherapeutics to malignant tumors. Acs Nano. 2013;7(9):7698–710. doi:10.1021/nn402232g. Epub 2013/09/06.
- Gangadaran P, Hong CM, Oh JM, Rajendran RL, Kalimuthu S, Son SH, Gopal A, Zhu L, Baek SH, Jeong SY, et al. In vivo non-invasive imaging of radio-labeled exosome-mimetics derived from red blood cells in mice. Front Pharmacol. 2018;9:817. doi:10.3389/fphar.2018.00817. Epub 2018/08/15.
- Jo W, Kim J, Yoon J, Jeong D, Cho S, Jeong H, Yoon YJ, Kim SC, Gho YS, Park J. Large-scale generation of cell-derived nanovesicles. Nanoscale. 2014;6(20):12056–64. doi:10.1039/C4NR02391A. Epub 2014/09/06.
- Kwon MH, Song KM, Limanjaya A, Choi MJ, Ghatak K, Nguyen NM, Ock J, Yin GN, Kang JH, Lee MR, et al. Embryonic stem cell-derived extracellular vesicle-mimetic nanovesicles rescue erectile function by enhancing penile neurovascular regeneration in the streptozotocin-induced diabetic mouse. Sci Rep. 2019;9(1):20072. doi:10.1038/s41598-019-54431-4. Epub 2019/12/29.
- Kailashiya J, Gupta V, Dash D. Engineered human platelet-derived microparticles as natural vectors for targeted drug delivery. Oncotarget. 2019;10(56):5835–46. doi:10.18632/oncotarget.27223. Epub 2019/10/28.
- Aatonen MT, Ohman T, Nyman TA, Laitinen S, Gronholm M, Siljander PR. Isolation and characterization of platelet-derived extracellular vesicles. J Extracell Vesicles. 2014;3(1):24692. doi:10.3402/jev.v3.24692. Epub 2014/08/26.
- Lopez E, Srivastava AK, Burchfield J, Wang YW, Cardenas JC, Togarrati PP, Miyazawa B, Gonzalez E, Holcomb JB, Pati S, et al. Platelet-derived- extracellular vesicles promote hemostasis and prevent the development of hemorrhagic shock. Sci Rep. 2019;9(1):17676. doi:10.1038/s41598-019-53724-y. Epub 2019/11/30.
- Midekessa G, Godakumara K, Ord J, Viil J, Lattekivi F, Dissanayake K, Kopanchuk S, Rinken A, Andronowska A, Bhattacharjee S, et al. Zeta potential of extracellular vesicles: toward understanding the attributes that determine colloidal stability. ACS Omega. 2020;5(27):16701–10. doi:10.1021/acsomega.0c01582. Epub 2020/07/21.
- Elsharkasy OM, Nordin JZ, Hagey DW, de Jong OG, Schiffelers RM, Andaloussi SE, Vader P. Extracellular vesicles as drug delivery systems: why and how? Adv Drug Deliv Rev. 2020;159:332–43. doi:10.1016/j.addr.2020.04.004. Epub 2020/04/20.
- Agrahari V, Agrahari V, Burnouf PA, Chew CH, Burnouf T. Extracellular microvesicles as new industrial therapeutic frontiers. Trends Biotechnol. 2019;37(7):707–29. doi:10.1016/j.tibtech.2018.11.012. Epub 2019/01/15.
- Andriolo G, Provasi E, Lo Cicero V, Brambilla A, Soncin S, Torre T, Milano G, Biemmi V, Vassalli G, Turchetto L, et al. Exosomes from human cardiac progenitor cells for therapeutic applications: development of a gmp-grade manufacturing method. Front Physiol. 2018;9:1169. doi:10.3389/fphys.2018.01169. Epub 2018/09/11.
- Lee H, Park H, Noh GJ, Lee ES. Ph-responsive hyaluronate-anchored extracellular vesicles to promote tumor-targeted drug delivery. Carbohydr Polym. 2018;202:323–33. doi:10.1016/j.carbpol.2018.08.141. Epub 2018/10/06.
- Tian Y, Li S, Song J, Ji T, Zhu M, Anderson GJ, Wei J, Nie G. A doxorubicin delivery platform using engineered natural membrane vesicle exosomes for targeted tumor therapy. Biomaterials. 2014;35(7):2383–90. doi:10.1016/j.biomaterials.2013.11.083. Epub 2013/12/19.
- Janowska-Wieczorek A, Marquez-Curtis LA, Wysoczynski M, Ratajczak MZ. Enhancing effect of platelet-derived microvesicles on the invasive potential of breast cancer cells. Transfusion. 2006;46(7):1199–209. doi:10.1111/j.1537-2995.2006.00871.x. Epub 2006/07/14.
- Gasperi V, Vangapandu C, Savini I, Ventimiglia G, Adorno G, Catani MV. Polyunsaturated fatty acids modulate the delivery of platelet microvesicle-derived microRnas into human breast cancer cell lines. J Nutr Biochem. 2019;74:108242. doi:10.1016/j.jnutbio.2019.108242. Epub 2019/10/31.
- Wang H, Wu J, Williams GR, Fan Q, Niu S, Wu J, Xie X, Zhu LM. Platelet-membrane-biomimetic nanoparticles for targeted antitumor drug delivery. J Nanobiotechnology. 2019;17(1):60. doi:10.1186/s12951-019-0494-y. Epub 2019/05/16.
- Li J, Ai Y, Wang L, Bu P, Sharkey CC, Wu Q, Wun B, Roy S, Shen X, King MR. Targeted drug delivery to circulating tumor cells via platelet membrane-functionalized particles. Biomaterials. 2016;76:52–65. doi:10.1016/j.biomaterials.2015.10.046.
- Pan V, Siva PN, Modery-Pawlowski CL, Sekhon UDS, Gupta AS. Targeted killing of metastatic cells using a platelet-inspired drug delivery system. RSC Adv. 2015;5(57):46218–28. doi:10.1039/C5RA05339K.
- Modery-Pawlowski CL, Master AM, Pan V, Howard GP, Sen Gupta A. A platelet-mimetic paradigm for metastasis-targeted nanomedicine platforms. Biomacromolecules. 2013;14(3):910–9. doi:10.1021/bm301996p. Epub 2013/01/31.
- Saari H, Lisitsyna E, Rautaniemi K, Rojalin T, Niemi L, Nivaro O, Laaksonen T, Yliperttula M, Vuorimaa-Laukkanen E. FLIM reveals alternative EV-mediated cellular up-take pathways of paclitaxel. J Control Release. 2018;284:133–43. doi:10.1016/j.jconrel.2018.06.015. Epub 2018/06/16.
- Mulcahy LA, Pink RC, Carter DR. Routes and mechanisms of extracellular vesicle uptake. J Extracell Vesicles. 2014;3(1):24641. doi:10.3402/jev.v3.24641. Epub 2014/08/22.
- Sisin NNT, Mat NFC, Rashid RA, Dollah N, Razak KA, Geso M, Algethami M, Rahman WN. Natural baicalein-rich fraction as radiosensitizer in combination with bismuth oxide nanoparticles and cisplatin for clinical radiotherapy. Int J Nanomed. 2022;17:3853–74. doi:10.2147/IJN.S370478. Epub 20220902.
- Jochums A, Friehs E, Sambale F, Lavrentieva A, Bahnemann D, Scheper T. Revelation of different nanoparticle-uptake behavior in two standard cell lines NIH/3T3 and A549 by flow cytometry and time-lapse imaging. Toxics. 2017;5(3):15. doi:10.3390/toxics5030015. Epub 20170719.