Abstract
Platelet-rich plasma (PRP) holds promise as a therapeutic modality for wound healing; however, immediate utilization encounters challenges related to volume, concentration, and consistency. Cryopreservation emerges as a viable solution, preserving PRP’s bioactive components and extending its shelf life. This study explores the practicality and efficacy of cryopreserved platelet-rich plasma (cPRP) in wound healing, scrutinizing both cellular mechanisms and clinical implications. Fresh PRP and cPRP post freeze-thaw underwent assessment in macrophage, fibroblast, and endothelial cell cultures. The impact of cPRP on active component release and cell behavior pertinent to wound healing was evaluated. Varied concentrations of cPRP (1%, 5%, 10%) were examined for their influence on cell polarization, migration, and proliferation. The results showed minimal changes in cPRP’s IL-1β levels, a slight decrease in PDGF-BB, and superior effects on macrophage M2 polarization and fibroblast migration, while no statistical significance was observed in endothelial cell angiogenesis and proliferation. Remarkably, 5% PRP exhibited the most significant stimulation among all cPRP concentrations, notably impacting cell proliferation, angiogenesis, and migration. The discussion underscores that cPRP maintains platelet phenotype and function over extended periods, with 5% cPRP offering the most favorable outcomes, providing a pragmatic approach for cold storage to extend post-thaw viability and amplify therapeutic effects.
Plain Language Summary
What is the context?
Platelet-rich plasma (PRP) is a potential bioactive material for wound healing, but using it immediately faces issues like volume, concentration, and consistency.
Low-temperature freezing is a method employed to preserve PRP. However, the current understanding of the effects of the freezing-thawing process on the components of PRP and its impact on cells relevant to wound healing remains unclear.
What is new?
This study explores the feasibility and effectiveness of using cryopreserved PRP at −80°C for promoting wound healing. This research stands out for its focus on cellular responses and practical implications in therapeutic contexts.
To understand their distinct impact on different cell types relevant to wound healing, the study meticulously examined various final concentrations of cPRP (1%, 5%, 10%).
The study identified the superior effects of 5% cPRP on crucial cellular activities, notably in cell polarization, proliferation, angiogenesis, and migration.
What is the impact?
Low-temperature freezing can be considered an effective method for PRP preservation.
Some bioactive components in cPRP exhibit subtle changes; however, these changes result in better effects on certain cell types related to healing.
The study illustrates that all concentrations of cPRP effectively enhance cell proliferation, migration, and differentiation, emphasizing the comparable efficacy of cryopreserved PRP to non-cryopreserved PRP.
Introduction
Platelet-rich plasma (PRP) has emerged as a promising therapeutic approach in the field of wound healing due to its regenerative properties and ability to accelerate tissue repair.Citation1–3 Traditional methods of PRP preparation have certain limitations in terms of collection volume, platelet concentration, and consistency. With the emergence of automated systems such as the Com Tec blood component separation machine, substantial progress has been made in the processing of PRP.Citation2,Citation4 The utilization of the Com Tec machine provides several notable benefits, including the capacity to handle larger volumes of blood samples, leading to elevated platelet concentrations. Moreover, this advanced system enables precise control over the separation procedure, ensuring enhanced standardization in PRP preparation.Citation5 Compared to immediate usage, cryopreserved platelet-rich plasma (cPRP) offers practical advantages to overcome limitations by providing longer shelf life, efficient inventory management, and standardized delivery.Citation6–8 cPRP proves to be a promising alternative in various clinical settings, enhancing patient care and treatment outcomes.Citation9–11
In recent years, cPRP has been the focus of extensive research due to its composition and clinical applications.Citation12–14 Thorough analyses of the components in cPRP have been conducted utilizing diverse experimental techniques, such as cell counting, growth factor analysis, and proteomics.Citation15–17 These investigations aim to evaluate the content and characteristics of platelets and other bioactive components present in cPRP.Citation18–21 Furthermore, clinical studies have explored the utilization of cPRP in various fields, including orthopedics, dermatology, and dentistry.Citation13,Citation22,Citation23 Combination therapies incorporating cPRP with stem cells, hyaluronic acid, or matrix-supported autologous cell transplantation have been investigated to enhance tissue repair and regeneration.Citation24–26 To comprehend the potential of cPRP in wound healing, a thorough examination of its in vitro cellular mechanisms is imperative. Several cellular processes, including inflammation regulation, cell proliferation, and migration, play pivotal roles in wound healing.Citation27,Citation28 Research has demonstrated that cPRP retains its ability to release growth factors and cytokines, which are crucial for promoting wound healing.Citation29,Citation30 A comprehensive understanding of these cellular mechanisms will provide valuable insights into the therapeutic potential of cPRP and its application in the context of wound healing. This article seeks to investigate the potential application of cPRP prepared using the Com Tec apheresis system, along with its underlying cellular mechanisms, in the context of wound healing.
Study design and methods
Study design
The study utilized a paired design to assess the impact of cryopreservation on platelet hemostatic activity. Platelet (PLT) units were evaluated before freezing, after thawing, and during post-thaw storage. In vitro experiments involved THP-1 macrophages, L929 fibroblasts, and human umbilical vein endothelial cells (HUVECs). Different cPRP concentrations were examined for effects on cell behavior, gene expression, and cytokine release using ELISA assays.
PRP preparation
Whole blood samples were obtained from 21 male healthy volunteers at the Department of Transfusion, The First Affiliated Hospital of Anhui Medical University, China. All healthy volunteers provided informed consent in accordance with the protocols approved by the Ethics Committee of Anhui Medical University (license number: 2023570). The blood collection was performed using venipuncture, resulting in a platelet concentration of 206.27 ± 41.76 × 103/µL, a red blood cell concentration of 4.56 ± 0.56 × 106/µL, and a white blood cell concentration of 5.59 ± 1.44 × 103/µL. Fresh PRP was prepared and purified using the Com Tec automated blood component separation machine (Fresenius Kabi, Germany), following the guidelines approved by the Ethics Committee of Anhui Medical University (License Number: 2023570). Platelet count was measured on a hematology analyzer (Beckman Coulter LH 785, America), and the platelet concentration was adjusted to 1381.57 × 103/µL. Compared to whole blood, there was a 6.70-fold increase in platelet concentration. Contaminating red and white blood cells were respectively below 0.10 × 106/µL and 0.11 × 103/µL.
Subsequently, the PRP was aliquoted aseptically, followed by cryopreservation at −80°C in an ultra-low temperature freezer. Prior to each use, the PRP was thawed in a 37°C water bath, and all PRP used in this research has been stored less than 3 months. In addition, the PRP samples was diluted with cell culture medium to obtain different concentrations (1%, 5%, 10%) in experimental groups.
Cell culture
THP-1 cells, derived from human monocytes, L929 fibroblast cells from mice, and HUVEC endothelial cells from human umbilical veins were obtained from the American Type Culture Collection (ATCC) (Manassas, VA, United States). THP-1 cells were cultured in RPMI 1640 medium, L929 cells in high-glucose DMEM, and HUVECs in DMEM/F12 medium (Gibco, America). The culture media were supplemented with 10% fetal bovine serum and 1% penicillin-streptomycin, and cells were maintained at 37°C in a 5% CO2 humidified incubator. The media were refreshed every three days, and cells were passaged 2–3 times per week.
Differentiation of THP-1 macrophages
THP-1 cells, derived from human monocytes, were used for macrophage differentiation. Cells were cultured in RPMI 1640 basal medium containing 10% fetal bovine serum and 1% penicillin-streptomycin at 37°C in a 5% CO2 incubator. THP-1 cells were seeded in a 6-well cell culture plate at a density of 8 × 10Citation5 cells/mL, and macrophage differentiation toward the M0 phenotype was induced by adding 100 ng/mL of phorbol 12-myristate 13-acetate (PMA) (Sigma, America) to the complete culture medium. After reaching the M0 phenotype, cells were further cultured and differentiated toward the M1 phenotype by adding 100 ng/mL lipopolysaccharide (LPS) (Sigma, America) to the complete culture medium. Cells were observed for morphological changes after 48 hours of incubation. For differentiation toward the M2 phenotype, 20 ng/mL of interleukin-4 (IL-4) and 20 ng/mL of interleukin-13 (IL-13) (Proteintech, America) were added to the complete culture medium, and cells were observed for morphological changes after 48 hours of incubation at 37°C.
Platelet activity assay
Fresh PRP (5 mL) and cPRP (5 mL), with a concentration of 1381.57 × 103/µL, were activated with a 250 U/mL thrombin activator by combining thrombin and calcium gluconate, and then placed in 10 mL of PBS buffer. Following the addition of thrombin and calcium gluconate, a fibrin clot was formed, signifying the activation of PRP and the initiation of growth factor release. The samples were shaken on a shaking platform at 37°C and 100 rpm. At predefined time intervals, 1 mL of PBS was collected, and an equal volume of fresh PBS was added. The concentrations of the growth factor PDGF-BB and the cytokine IL-1β released from platelets in fresh and cPRP were evaluated using an ELISA assay (R&D Systems, America) to assess the activity levels before and after cryopreservation.
Quantifying cytokine levels in fresh PRP and cPRP
The assessment of fresh PRP and cPRP utilizing the ELISA assay (R&D Systems, America) was performed strictly in accordance with the manufacturer’s instructions.
Detection of the effects of cPRP on macrophage polarization
Immunofluorescence staining of CD206/DAPI to assess the impact of PRP on macrophage M1/M2 polarization: THP-1 cells were seeded in confocal culture dishes and induced into M0 phenotype, then co-cultured with the different samples for 3 days. After washing twice with PBS, the cells were blocked for 1 hour and then stained overnight at 4°C with the primary antibody CD206 (Cell Signaling, America). Following three washes with PBS, the cells were incubated with Alexa Fluor 488-conjugated goat anti-rabbit IgG (1:500) for 2 hours. After three washes with PBS, the cells were stained with DAPI-containing mounting medium. Finally, confocal images were obtained, and the fluorescence intensity of positive expression was measured using ImageJ software.
qPCR analysis of the expression of macrophage differentiation-related genes
M0 macrophages were co-cultured with the different samples mentioned above. After 3 and 5 days of culture, cells were collected, and RNA was extracted following the manufacturer’s instructions. The RNA concentration was determined using a Nanodrop, and RT-PCR was performed to reverse transcribe RNA into cDNA. The relative expression levels of macrophage polarization-related genes (IL-6 and IL-10) were then measured using qPCR with the respective templates ().
Table I. Related gene primer sequences for RT-PCR and qPCR analysis.
Flow cytometry analysis of macrophage surface marker expression
M0 macrophages were co-cultured with cPRP at different final concentrations in macrophage culture medium for 3 days. Cells were collected, resuspended in PBS buffer, centrifuged at 1500 rpm for 3 minutes, and washed. After adjusting the cell concentration, cells were incubated with V450 PB-CD80 and PC7-CD163 fluorescent antibodies in the dark at 4°C for 30 minutes. After washing the cells with PBS, they were fixed with 200 μL of IC fixation buffer and incubated in the dark at 4°C for 1 hour. Following two washes with permeabilization buffer, 100 μL of permeabilization buffer was added to resuspend the cells, and APC-CD206 fluorescent antibody was added. After further incubation, the cells were washed with PBS, resuspended in 400 μL of PBS, filtered through a 300-mesh filter, and analyzed using flow cytometry.
Impact of cPRP on L929 cell migration
Scratch assay
L929 cells were seeded in a 6-well plate at a density of 1 × 106 cells per well. After pre-culturing overnight, a scratch was made when the cells reached 80% confluence. After washing the cells with PBS three times to remove floating cells, the medium containing 5% cPRP or different final concentrations of cPRP was added for further incubation. The cells were imaged at 0 and 12 hours using a microscope, and the migration area was analyzed using ImageJ software.
Impact of cPRP on HUVECs angiogenic ability
In vitro angiogenesis assay
Pre-chilled matrigel matrix gel (Corning, America) was added to a 24-well plate at 250 μL per well and allowed to solidify at 37°C and 5% CO2 for 1 hour. Starved HUVECs were digested and seeded at a density of 5 × 105 cells/mL. Then, 5% cPRP or different final concentrations of cPRP were added. The control group received HUVECs complete culture medium. Each well was supplemented with 500 μL of cell suspension and incubated at 37°C and 5% CO2 for 4 hours. The formation of endothelial cell tubules, number of tubules, and tubule length were recorded using a microscope and analyzed using ImageJ software.
VEGF gene expression analysis
HUVECs were seeded in a 6-well plate at a density of 1 × 104 cells per well. After pre-culturing overnight, 5% cPRP or different final concentrations of cPRP were added. The control group received L929 complete culture medium. After co-culturing for 3, 5, and 7 days, RNA was extracted for RT-PCR and qPCR analysis to determine the expression levels of the VEGF gene.
Impact of PRP on L929 and HUVECs proliferation
L929 and HUVECs were respectively seeded in a 96-well plate at a density of 5 × 103 cells per well. After pre-culturing overnight, 5% cPRP or different final concentrations of cPRP were added. At 1, 3, and 5 days, 10 μL of CCK-8 solution was added to each well, followed by incubation at 37°C. The optical density (OD) at 450 nm was measured. Each time point had three replicates and included blank controls and negative controls.
Statistics analysis
All data are presented as mean ± standard deviation (SD). GraphPad Prism 6.0 was used for data visualization and analysis. The significance of differences between the fresh and post-cPRP treatment groups was assessed using a two-tailed t-test. For comparisons among three or more groups, one-way analysis of variance (ANOVA) was performed. A p-value of less than 0.05 was considered statistically significant, indicating meaningful results.
Results
Differences in appearance and release of bioactive components between fresh PRP and cPRP
PRP collected through the Com Tec apheresis system () was cryopreserved at −80°C to obtain cPRP (). Upon thawing, Visual analysis of PRP assessed for signs of precipitation (), stratification (visible layer separation), and deterioration (e.g., color changes, clot formation, particulate presence), with no significant differences observed. Subsequently, we assessed the differential release of cytokines (IL-1β) and growth factors (PDGF-BB) from platelets in the two sample groups by ELISA analysis. As shown in , there was no significant difference in the release of IL-1β between the two sample groups within 72 hours. Regarding PDGF-BB (), the cumulative release was comparable within the first 6 hours but differed after 12 hours. At 72 hours, the cumulative release of PDGF-BB in fresh PRP was 12 212.25 ± 1041.21 pg/mL, while in cPRP, it was 8040.01 ± 333.87 pg/mL. This difference may be attributed to the slight structural or functional impact of cryopreservation on PDGF-BB; nevertheless, cPRP still exhibited a relatively high release capacity.
Figure 1. Differences in appearance and release of bioactive components between fresh PRP and cPRP. (a) A photograph of fresh PRP. (b) A photograph of PRP passing through − 80°C freezing. (c) A photograph of cPRP. (d) The cumulative release profiles of the cytokine IL-1β in fresh and cryopreserved PRP. (e) The cumulative release profiles of the cytokine PDGF-BB in fresh and cryopreserved PRP.
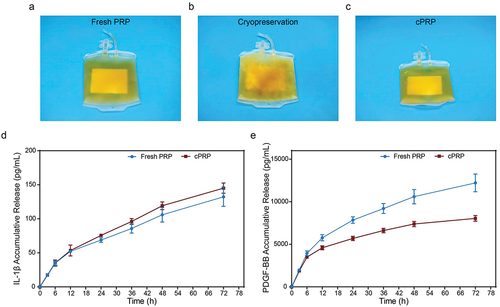
Quantifying cytokine levels in fresh PRP and cPRP
ELISA quantification assessed factor concentrations in pre-collection plasma, Fresh PRP, and cPRP. The results demonstrated that compared to pre-collection plasma, fresh PRP exhibited a more than twofold increase in enriched concentrations of various cytokines. Additionally, the concentrations of PDGF-BB and VEGF in cPRP significantly increased, reaching 3226.87 ± 194.90 pg/mL and 357.02 ± 30.85 pg/mL, respectively, markedly higher than the levels in fresh PRP. This might be due to cryopreservation process subjected PRP platelets to physical stimulation, resulting in structural breakdown and subsequent release of intracellular bioactive substances, notably causing significant release of cytokines PDGF-BB (), VEGF (), and growth factor IL-10 () in cPRP. These experimental outcomes further validate the concentration of growth factors and cytokines in PRP compared to pre-collection plasma, emphasizing the enhanced release of these biological components in PRP following the freezing-thawing process.
Figure 2. Quantifying cytokine levels in fresh PRP and cPRP. (a–c) the concentration of the cytokine PDGF-BB (a), VEGF (b) and IL-10 (c) in pre-collection plasma, fresh PRP, and cPRP. The above data are expressed as mean ± standard deviation, ns, not significant; *p < .05, **p < .01, ***p < .001, ****p < .0001.
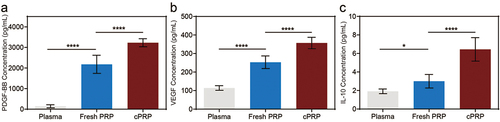
Effects of fresh PRP and cPRP on cellular responses
Despite minor differences observed in factor release between fresh PRP and cPRP, evaluating the influence of cryopreservation on various wound-healing-relevant cells necessitates in vitro assays. Among these cells, macrophages play a pivotal role during inflammation and repair stages, with appropriate polarization aiding in balancing inflammatory responses, tissue restoration, and accelerated wound recovery.Citation31,Citation32 Co-culturing macrophages with pre and post-cryopreservation PRP facilitated evaluation of macrophage polarization using CD206/DAPI immunofluorescence staining (). Statistical analysis of fluorescence intensity indicated that CD206, a representative marker of M2 macrophages, exhibited comparable average expression levels in fresh and cPRP co-cultures, with no statistically significant differences (). In q-PCR analysis, the varying expression levels of distinct genes hold relevance to macrophage polarization. Specifically, the anti-inflammatory cytokine IL-10 demonstrates a substantial upregulation in M2 macrophages, while the pro-inflammatory cytokine IL-6 witnesses heightened expression in M1 macrophages. In comparison to the control group, a three-day co-culture yields no statistically significant divergence in cellular polarization between the two groups, rendering notable M2 polarization inconspicuous. However, subsequent to a five-day co-culture, the cPRP group showcases a heightened level of IL-10 gene expression, standing in marked contrast to both the control and fresh PRP groups (p < .05) (). Conversely, in terms of IL-6 gene expression levels, both PRP groups exhibit a notably diminished expression after three and five days of co-culture relative to the control group (). These findings suggest that M1 macrophage polarization is inhibited, and there is minimal variation between pre and post-cryopreservation states.
Figure 3. The comparison of in vitro cell evaluation between the fresh PRP and cPRP. (a) Fluorescence images of co-cultured macrophages with the fresh PRP and cPRP, respectively. The macrophage polarization was evaluated through CD206/DAPI immunofluorescence staining (green: CD206; blue: DAPI). The scale bars are 20 µm. (b) Statistical analysis results of average fluorescence intensity of CD206 of fresh PRP and cPRP co-culturing macrophages. (c, d) compare the expression levels of macrophage polarization related genes IL-10 (c) and IL-6 (d) after 3 days and 5 days coculturing. GAPDH as the internal reference to calculate the ct value. (e, f) CCK-8 absorbance values were obtained by co-culturing L929 cells (e), and HUVEC cells (f), with the fresh PRP and cPRP for 1 day, 3 days, and 5 days, respectively. (g, i) scratch test and migration area percentage statistical results of L929 in 5% fresh PRP and 5% cPRP for 12 hours. (h, j, k) after culturing HUVECs in a culture medium containing 5% fresh PRP and 5% cPRP for 4 hours, the number of meshes (j) and total length (k) were statistically analyzed. (l) The mRNA expression of angiogenesis-related gene VEGF is assessed using qPCR after 3 days, 5 days, and 7 days of HUVEC training with the fresh PRP and cPRP. The above data are expressed as mean ± standard deviation, ns, not significant; *p < .05, **p < .01, ***p < .001, ****p < .0001.
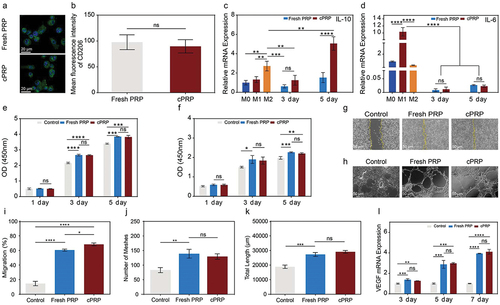
During the regeneration phase of wound healing, the proliferation of fibroblasts and endothelial cells serves as the cornerstone for the reconstruction of tissue structure.Citation33,Citation34 As depicted in , it is evident that, in comparison to the control group, both fresh PRP and cPRP significantly promoted fibroblast proliferation after three and five days of co-culture. Additionally, there were no statistically significant differences between the two PRP groups, implying that cryopreservation does not impede the ability of PRP to stimulate fibroblast proliferation. In a separate experiment, PRP demonstrated a significant enhancement in the proliferation of human endothelial cells (). Following a three-day co-culture, the co-culture of fresh PRP exhibited a marked variance in cell count in contrast to the control group, whereas this variance was less distinct with cPRP. By the fifth day, the cell count of both PRP co-culture groups exceeded that of the control group.
The proliferation and migration of fibroblasts facilitate their recruitment to damaged tissue sites. In the in vitro scratch assay, over a 12-hour period, the closure degree of cell scratch areas co-cultured with both PRP groups was notably higher compared to the control group (). Notably, cPRP demonstrated a higher migration rate than fresh PRP. Evaluating the branching pattern and length of tubular structures formed by endothelial cells in culture dishes permits the evaluation of the ability of distinct culture conditions to stimulate endothelial cell angiogenesis. Morphological statistics of HUVECs cultured under different conditions () demonstrated that, relative to the group with normal culture medium, co-culture with both fresh PRP and cPRP led to greater numbers of vascular branching points and longer capillary lengths (). However, no statistically significant differences were detected between the two PRP groups. Elevated VEGF gene expression in endothelial cells serves as a marker of enhanced angiogenesis. qPCR analysis indicated that both PRP groups significantly enhanced VEGF gene expression across consecutive time points relative to the control group, with no significant intergroup differences (). Hence, a sequence of in vitro experiments indicates that cryopreservation preserves the bioactive properties of PRP. Concerning cells associated with wound healing, cryopreservation exerts a minimal influence on cellular responses, and in certain instances, cPRP exhibits even improved efficacy. Consequently, coupled with the clinical applicability facilitated by cPRP, it showcases considerable potential for therapeutic applications.
Effect of cPRP concentration on polarization of macrophages
Platelets within cPRP are rich in growth factors and cytokines that can influence cell proliferation, differentiation, and functionality.Citation29 Variations in cPRP concentrations might lead to diverse cellular responses, as excessively high concentrations could induce cellular hyperstimulation, while lower concentrations might not provide adequate bioactive components. Thus, the exploration of optimal concentrations to harness the stimulatory effects of cPRP is crucial for enhancing its therapeutic efficacy in wound treatment.
Firstly, to investigate the impact of different cPRP concentrations on polarization, macrophages were co-cultured with cPRP at 1%, 5%, and 10% concentrations, respectively. Microscopic examination of CD206/DAPI immunofluorescence-stained cells showed that all three cPRP concentration groups induced macrophage polarization toward the M2 phenotype, with the 5% concentration group displaying a notably elongated morphology compared to the others (). Quantitative analysis of fluorescence intensity supported these observations, revealing significant CD206 expression across all co-cultured macrophage groups. Moreover, the 5% cPRP concentration led to the highest CD206 expression, while the 10% concentration group exhibited the lowest expression levels among the tested concentrations ().
Figure 4. Effect of cPRP concentration on polarization of macrophages. (a) Fluorescence images of co-cultured macrophages with three groups of cPRP with varying concentration. The macrophage polarization was evaluated through CD206/DAPI immunofluorescence staining (green: CD206; blue: DAPI). (b) Statistical analysis results of average fluorescence intensity of CD206 of fresh PRP and cPRP co-culturing macrophages. (c, d) the expression levels of macrophage polarization related genes IL-6 (c), and IL-10 (d), after co-culturing with three cPRP concentration groups for 3 days and 5 days. (e–g) statistical charts of the expression levels of CD80, CD163, and CD206, markers of M0, M1, and M2 macrophages. (h–j) statistical charts comparison of the differences in the expression of macrophage surface markers CD80, CD163, and CD206 after co-culturing with three cPRP concentration groups. The above data are expressed as mean ± standard deviation, ns, not significant; *p < .05, **p < .01, ***p < .001, ****p < .0001.
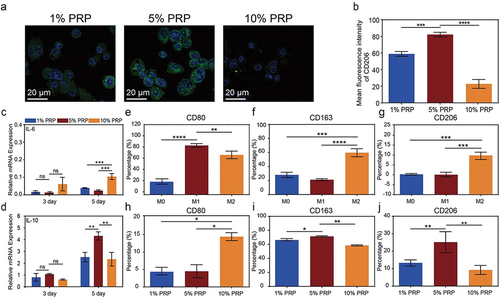
By conducting q-PCR analysis on cytokine gene expression, compared to the control group, macrophages co-cultured with cPRP at three different concentrations exhibited lower expression levels of the M1 polarization-associated factor IL-6, while showing higher expression of the M2 polarization-associated factor IL-10 (). Notably, after five days of co-culture, IL-10 expression levels significantly increased relative to the three-day co-culture, with the 5% concentration group displaying the highest expression. These findings underscore the M2 polarization-inducing capacity of all cPRP concentrations, with the 5% concentration proving to be the most effective.
Continuing the investigation, we proceeded to select three specific factor expressions as markers, and subsequently utilized flow cytometry to sort the co-cultured macrophages (). The outcomes revealed that, concerning the M1 cell-associated marker CD80, the proportion of CD80-labeled cells was markedly reduced in the cPRP co-culture groups compared to the control (). Conversely, upon sorting based on M2 cell-associated markers CD163 and CD206, all three co-culture groups exhibited distinct trends toward M2 macrophage polarization in contrast to the control group. Specifically, over 60% of cells expressed CD163 and more than 10% of cells displayed CD206 expression. Consistently across the results of the flow cytometry experiments for all three groups, the 10% cPRP group exhibited the least suppression of M1 macrophage polarization and the least promotion of M2 macrophage polarization, while the 5% cPRP group demonstrated the most favorable outcomes in terms of macrophage polarization effects ().
Effect of cPRP concentration on the fibroblast and endothelial cell
Autologous PRP from patients offers potential advantages, avoiding potential foreign body rejection and immune responses. Different cPRP concentrations were co-cultured with L929 fibroblasts to assess cellular compatibility. All cPRP concentration groups showed consistently high compatibility over time, with cell viability exceeding 100% compared to controls (). The growth factors from PRP can enhance fibroblast proliferation, expediting new tissue formation and wound healing. This was affirmed by CCK-8, demonstrating significant proliferation enhancement for L929 cells in all cPRP concentration groups. Particularly, the 5% cPRP concentration exhibited the most substantial promotion compared to other groups ().
Figure 5. Effect of cPRP concentration on the proliferation and migration fibroblast. (a) The results of CCK-8 absorbance values after co-culturing L929 cells with three cPRP concentration groups for 1 day, 3 days, and 5 days, respectively. (b) Cell viability analysis after co-culturing L929 cells with three cPRP concentration groups for 1 day, 3 days, and 5 days. (c, d,) scratch test and migration area percentage statistical results of L929 in culture media containing different final concentrations of cPRP for 12 hours. The above data are expressed as mean ± standard deviation, ns, not significant; *p < .05, **p < .01, ***p < .001, ****p < .0001.
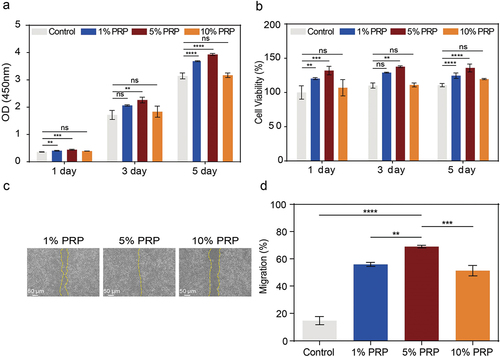
In the scratch assay, the process of wound filling at the scratched area reflects fibroblast migration capability. On a densely covered monolayer of cells, consistent initial scratch widths exhibited variations in the filling rate after 12 hours of co-culture with various cPRP concentrations (). The statistical results in demonstrated that all three cPRP co-culture groups exhibited higher migration rates compared to the blank control, with the 5% concentration group showing the highest migration rate, indicating the most effective promotion of fibroblast migration capability.
Endothelial cells play a crucial role in constituting the endothelial layer of blood vessels. During the wound healing process, the bioactive agents present abundantly in PRP play a vital role in promoting the proliferation of endothelial cells. This proliferation is crucial for the formation of new vascular structures and effective tissue repair.Citation33 Similar to the outcomes observed in promoting fibroblast proliferation, different concentrations of cPRP stimulate HUVECs proliferation, with the most significant effect demonstrated by the 5% concentration (). Cell viability assays demonstrate that various concentrations of cPRP maintain a high level of compatibility with HUVECs, whether assessed at 1, 3, or 5 days ().
Figure 6. Effect of cPRP concentration on the vascularization and proliferation of HUVECs. (a) The results of CCK-8 absorbance values after co-culturing HUVECs with three cPRP concentration groups for 1 day, 3 days, and 5 days, respectively. (b) Cell viability analysis after co-culturing HUVECs with three cPRP concentration groups for 1 day, 3 days, and 5 days. (c–e) after culturing HUVECs for 4 hours in culture medium containing different final concentrations of cPRP(c), the total length of in vitro angiogenesis (d) and the number of meshes (e) were statistically analyzed. (f) After co-culturing with HUVECs at different final concentrations of cPRP for 3 days, 5 days, and 7 days, q-PCR showed mRNA expression of angiogenesis-related gene VEGF. The above data are expressed as mean ± standard deviation, ns, not significant; *p < .05, **p < .01, ***p < .001, ****p < .0001.
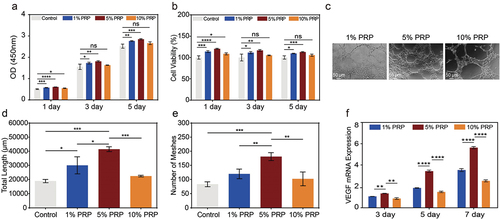
Growth factors present in PRP, particularly platelet-derived vascular endothelial growth factor (VEGF), are known to stimulate the formation of blood vessel networks in wound areas, facilitating the delivery of oxygen and nutrients essential for wound healing.Citation20 After co-culturing HUVECs with different concentrations of cPRP for four hours, a consistent angiogenic tendency was observed in the matrix (). Analysis of tube length revealed that all three concentrations of cPRP promoted tube length growth compared to the control group cultured in regular growth medium, with the 5% concentration exhibiting the most significant effect (). The number of branching points in tubular structures is also a crucial indicator of angiogenesis. Statistical results further indicated the efficacy of cPRP in augmenting the number of branching points, with the 5% concentration yielding the most favorable outcomes (). Finally, qPCR analysis of the VEGF gene expression of co-cultured HUVECs confirms the pro-angiogenic potential of cPRP. Nonetheless, in comparison to the 1% and 10% concentrations, the 5% concentration consistently manifested heightened VEGF expression levels, spanning both the 3-day and subsequent 5-day and 7-day time points ().
Discussion
PRP is widely used in the fields of sports medicine, traumatology, and orthopedics, and has become a highly regarded therapeutic modality.Citation22,Citation35–37 Its rich content of growth factors and cytokines demonstrates potential efficacy in promoting tissue healing, facilitating soft tissue and bone regeneration, and reducing pain while shortening recovery time.Citation38,Citation39 In sports medicine, it is commonly employed for treating muscle strains, tendon injuries, and joint damage, as well as for enhancing athletes’ recovery and performance.Citation40–42 In traumatology, it is extensively utilized to accelerate wound healing, facilitate soft tissue repair, and promote fracture healing.Citation43–45 In orthopedic surgery, the use of PRP can stimulate bone regeneration, reduce postoperative complications, and expedite the recovery process.Citation46,Citation47 The successful clinical applications of PRP serve as reliable support for its further utilization in medical practice.
The generation process of cPRP mirrors that of human platelet lysate (HPL), the latter being a widely acknowledged substitute for fetal bovine serum in cell culture.Citation48–51 HPL has garnered global recognition for its efficacy, with numerous studies emphasizing its concentration of growth factors and cytokines compared to non-frozen PRP, along with their impact on cell proliferation.Citation52–55 Given its established efficacy as both a cell culture medium and a clinically tested blood product for tissue regeneration across various disorders, we compare cPRP with non-frozen PRP in this manuscript.Citation56–58 From a clinical perspective, cryopreservation technology offers a practical solution in addressing the challenges associated with the long-distance transportation and long-term storage of PRP.Citation59 The utilization of cPRP, achieved through freezing, offers the flexibility of on-demand utilization, alleviating constraints imposed by time and location and thereby adapting better to diverse clinical settings.Citation60,Citation61 In contrast, the application of fresh PRP is constrained by a limited timeframe for collection, preparation, and utilization. This study demonstrates that cPRP maintains its bioactivity; however, distinctions persist between cPRP and fresh PRP, potentially attributed to cryopreservation-induced impact on specific bioactive molecules.Citation9,Citation21 Further investigation could focus on the specialized steps involved in cPRP preparation and management to ensure that the freezing and thawing processes do not compromise its effectiveness.
Given the intricacies of clinical application, precise control over the concentration of cPRP is crucial for maximizing its therapeutic efficacy.Citation62 The experimental findings of this study have unveiled the pronounced influence of cPRP concentration on pivotal cellular processes implicated in wound healing, encompassing macrophage polarization, fibroblast proliferation and migration, as well as endothelial cell proliferation and angiogenesis. Through systematic assessment, this work has identified optimal cPRP concentration ranges for specific cellular responses, thereby furnishing substantial foundations for personalized treatment approaches. Such meticulous control not only facilitates tailored therapeutic strategies to address distinct wound healing stages, but also curtails unnecessary cellular responses arising from excessive or inadequate concentrations, thereby ensuring the attainment of optimal treatment efficacy in clinical applications.
The experimental data and conclusions derived from this study bear significant implications for guiding and advancing the future utilization of PRP in clinical applications. Macrophages, fibroblasts, and endothelial cells play pivotal roles in different phases of wound healing, and the precise modulation of cPRP concentration can optimize their responses during the inflammatory and regenerative stages. This facet holds promising potential for promoting wound healing, mitigating scar formation, and enhancing tissue regeneration. Informed by the guidance offered by this study, forthcoming clinical research and applications can adeptly select and adjust cPRP concentrations, thereby attaining more efficient and personalized therapeutic effects. Moreover, the implications of this study extend to diverse domains of PRP application, encompassing fields like trauma surgery, plastic surgery, and sports medicine. In summation, the experimental results presented herein not only offer pivotal experimental support for the clinical application of cPRP but also lay a robust foundation for the extensive use of PRP in various medical contexts in the future.
However, this study still has certain limitations. Different freezing conditions may exert varying effects on PRP after freezing, such as storage temperature and duration, potentially influencing the activity and biological properties of platelets, requiring further in-depth investigation. Additionally, outcomes obtained from in vitro experiments may not entirely reflect in vivo human conditions. Hence, for the extrapolation of experimental findings into clinical medical applications, more studies are needed to validate their efficacy and safety.
Conclusion
Through a series of in vitro experiments, this study extensively investigated the impact of cryopreservation on PRP bioactivity and its cellular effects in wound healing processes. The findings demonstrated the robust maintenance of bioactivity in cPRP, which exhibited significant regulatory capabilities in key cellular processes such as macrophage polarization, fibroblast proliferation, and endothelial cell functionality. Notably, distinct cPRP concentrations displayed varying degrees of regulatory influence on cellular functions. These discoveries offer substantive groundwork for personalized therapies, enabling future clinical applications and research to selectively adjust cPRP concentrations for enhanced treatment outcomes. Moreover, these insights establish a solid foundation for the broad applications of PRP in the medical domain.
Acknowledgments
We thank the blood donors as well as the staff at the blood centers making this study possible.
Disclosure statement
No potential conflict of interest was reported by the author(s).
Additional information
Funding
References
- Xu P, Wu Y, Zhou L, Yang Z, Zhang X, Hu X, Yang J, Wang M, Wang B, Luo G. et al. Platelet-rich plasma accelerates skin wound healing by promoting re-epithelialization. Burns Trauma. 2020;8:tkaa028. doi: 10.1093/burnst/tkaa028.
- Oneto P, Etulain J. PRP in wound healing applications. Platelets. 2020;32(2):189–11. doi: 10.1080/09537104.2020.1849605.
- dos Santos RG, Santos GS, Alkass N, Chiesa TL, Azzini GO, da Fonseca LF, dos Santos AF, Rodrigues BL, Mosaner T, Lana JF. The regenerative mechanisms of platelet-rich plasma: a review. Cytokine. 2021;144:155560–8. doi: 10.1016/j.cyto.2021.155560.
- Keklik M, Eser B, Kaynar L, Sivgin S, Keklik E, Solmaz M, Ozturk A, Buyukoglan R, Yay M, Cetin M. et al. Comparison of plateletpheresis on the fenwal amicus, Fresenius COM.TEC, and trima accel cell separators. J Clin Apher. 2015;30(3):171–5. doi: 10.1002/jca.21358.
- Philip J, Biswas AK, Chatterjee T, Mallhi RS. Comparative analysis of various aspects of plateletpheresis on the fenwal amicus and Fresenius COM.TEC cell separator instruments. Lab Med. 2014;45(4):315–23. doi: 10.1309/LM491RNLMWLFHIMS.
- Slichter SJ, Dumont LJ, Cancelas JA, Jones M, Gernsheimer TB, Szczepiorkowski ZM, Dunbar NM, Prakash G, Medlin S, Rugg N. et al. Safety and efficacy of cryopreserved platelets in bleeding patients with thrombocytopenia. Transfusion. 2018;58(9):2129–38. doi: 10.1111/trf.14780.
- Jimenez-Marco T, Castrillo A, Hierro-Riu F, Vicente V, Rivera J. Frozen and cold-stored platelets: reconsidered platelet products. Platelets. 2021;33(1):27–34. doi: 10.1080/09537104.2021.1967917.
- Kleinveld DJB, Sloos PH, Noorman F, Maas MAW, Kers J, Rijnhout TWH, Zoodsma M, Hoencamp R, Hollmann MW, Juffermans NP. et al. The use of cryopreserved platelets in a trauma‐induced hemorrhage model. Transfusion. 2020;60(9):2079–89. doi: 10.1111/trf.15937.
- McLean C, McMillan L, Petrik J, Fraser AR, Morrison A. Cryopreserved platelets and their suitability in being re‐suspended in additive solution. Vox Sang. 2020;115(8):676–85. doi: 10.1111/vox.12993.
- Chadebech P, de Ménorval M-A, Bodivit G, Mekontso‐Dessap A, Pakdaman S, Jouard A, Galactéros F, Bierling P, Habibi A, Pirenne F. et al. Evidence of benefits from using fresh and cryopreserved blood to transfuse patients with acute sickle cell disease. Transfusion. 2016;56(7):1730–8. doi: 10.1111/trf.13636.
- Kaux JF, Libertiaux V-O, Dupont L, Colige A, Denoël V, Lecut C, Hego A, Gustin M, Duwez L, Oury C. et al. Platelet-rich plasma (PRP) and tendon healing: comparison between fresh and frozen-thawed PRP. Platelets. 2019;31(2):221–5. doi: 10.1080/09537104.2019.1595563.
- Huber SC, Montalvão SADL, da Silv LQ, Junior JLRC, De Paula EV, Annichino-Bizzacchi JM. Freeze-dried platelet rich plasma (PRP) has biological activity in acute wound healing of animals, inducing significantly angiogenesis. Blood. 2016;128(22):5030–3. doi: 10.1182/blood.V128.22.5030.5030.
- Kinoshita H, Orita S, Inage K, Fujimoto K, Shiga Y, Abe K, Inoue M, Norimoto M, Umimura T, Ishii T. et al. Freeze-dried platelet-rich plasma induces osteoblast proliferation via platelet-derived growth factor receptor-mediated signal transduction. Asian Spine J. 2020;14(1):1–8. doi: 10.31616/asj.2019.0048.
- Stolla M, Li VJ, Mack JP. Preparation, preservation, and storage of platelet concentrates. In: Simon TL, Gehrie EA, McCullough J, Roback JD, Snyder EL, editors. Rossi’s Principles of Transfusion Medicine; 2022. p. 179–87 doi: 10.1002/9781119719809.ch17.
- Qiao J, An N, Ouyang X. Quantification of growth factors in different platelet concentrates. Platelets. 2017;28(8):774–8. doi: 10.1080/09537104.2016.1267338.
- Baba K, Yamazaki Y, Sone Y, Sugimoto Y, Moriyama K, Sugimoto T, Kumazawa K, Shimakura Y, Takeda A. An in vitro long-term study of cryopreserved umbilical cord blood-derived platelet-rich plasma containing growth factors—PDGF-BB, TGF-β, and VEGF. J Craniomaxillofac Surg. 2019;47(4):668–75. doi: 10.1016/j.jcms.2019.01.020.
- Kim JI, Bae HC, Park HJ, Lee MC, Han HS. Effect of storage conditions and activation on growth factor concentration in platelet‐rich plasma. J Orthop Res. 2019;38(4):777–84. doi: 10.1002/jor.24520.
- Whitney KE, Dornan GJ, King J, Chahla J, Evans TA, Philippon MJ, LaPrade RF, Huard J. The effect of a single freeze–thaw cycle on matrix metalloproteinases in different human platelet-rich plasma formulations. Biomedicines. 2021;9(10):1043–59. doi: 10.3390/biomedicines9101403.
- Melnikov DV, Kirillova KA, Zakharenko AS, Sinelnikov MY, Ragimov AA, Istranov AL, Startseva OI. Effect of cryo-processing on platelet-rich autoplasma preparations. Sovremennye Tekhnologii Meditsine. 2021;12(6):54–60. doi: 10.17691/stm2020.12.6.07.
- Roffi A, Filardo G, Assirelli E, Cavallo C, Cenacchi A, Facchini A, Grigolo B, Kon E, Mariani E, Pratelli L. et al. Does platelet-rich plasma freeze-thawing influence growth factor release and their effects on chondrocytes and synoviocytes? Biomed Res Int. 2014;2014:692913–44. doi: 10.1155/2014/692913.
- Hosny N, Goubran F, BadrEldin Hasan B, Kamel N. Assessment of vascular endothelial growth factor in fresh versus frozen platelet rich plasma. J Blood Transfus. 2015;2015:706903–9. doi: 10.1155/2015/706903.
- Feigin K, Shope B. Use of platelet-rich plasma and platelet-rich fibrin in dentistry and oral surgery: introduction and review of the literature. J Vet Dent. 2019;36(2):109–23. doi: 10.1177/0898756419876057.
- Sharun K, Pawde AM. Variables affecting the potential efficacy of platelet-rich plasma in dermatology. J Am Acad Dermatol. 2021;84(1):47–8. doi: 10.1016/j.jaad.2020.08.080.
- Vadala G, Russo F, Musumeci M, D’Este M, Cattani C, Catanzaro G, Tirindelli MC, Lazzari L, Alini M, Giordano R. et al. Clinically relevant hydrogel-based on hyaluronic acid and platelet rich plasma as a carrier for mesenchymal stem cells: rheological and biological characterization. J Orthop Res. 2017;35(10):2109–16. doi: 10.1002/jor.23509.
- Meamar R, Ghasemi-Mobarakeh L, Norouzi MR, Siavash M, Hamblin MR, Fesharaki M. Improved wound healing of diabetic foot ulcers using human placenta-derived mesenchymal stem cells in gelatin electrospun nanofibrous scaffolds plus a platelet-rich plasma gel: a randomized clinical trial. Int Immunopharmacol. 2021;101(Pt B):108282–90. doi: 10.1016/j.intimp.2021.108282.
- Zhang L, Miao H, Wang D, Qiu H, Zhu Y, Yao X, Guo Y, Wang Z. Pancreatic extracellular matrix and platelet-rich plasma constructing injectable hydrogel for pancreas tissue engineering. Artif Organs. 2020;44(12):532–51. doi: 10.1111/aor.13775.
- Monsuur H, van den Broek L, Jhingoerie R, Vloemans A, Gibbs S. Burn eschar stimulates fibroblast and adipose mesenchymal stromal cell proliferation and migration but inhibits endothelial cell sprouting. Int J Mol Sci. 2017;18(8):1790–801. doi: 10.3390/ijms18081790.
- Park G, Qian W, Zhang M-J, Chen Y-H, Ma L-W, Zeng N, Lu Q, Li Y-Y, Ma W-W, Yin X-F. et al. Platelet-rich plasma regulating the repair of ultraviolet B–induced acute tissue inflammation: adjusting macrophage polarization through the activin receptor–follistatin system. Bioengineered. 2021;12(1):3125–36. doi: 10.1080/21655979.2021.1944026.
- Novakovic VA, Chatterjee M, Gilbert GE. Cryopreserved platelets retain agonist responsiveness and support for factor VIII function. Blood. 2020;136(Supplement 1):3–4. doi: 10.1182/blood-2020-143366.
- Johnson L, Reade MC, Hyland RA, Tan S, Marks DC. In vitro comparison of cryopreserved and liquid platelets: potential clinical implications. Transfusion. 2015;55(4):838–47. doi: 10.1111/trf.12915.
- Wu J, Zhang L, Shi J, He R, Yang W, Habtezion A, Niu N, Lu P, Xue J. Macrophage phenotypic switch orchestrates the inflammation and repair/regeneration following acute pancreatitis injury. EBioMedicine. 2020;58:102920–32. doi: 10.1016/j.ebiom.2020.102920.
- Louiselle AE, Niemiec SM, Zgheib C, Liechty KW. Macrophage polarization and diabetic wound healing. Transl Res. 2021;236:109–16. doi: 10.1016/j.trsl.2021.05.006.
- Gurevich DB, David DT, Sundararaman A, Patel J. Endothelial heterogeneity in development and wound healing. Cells. 2021;10(9):2338–43. doi: 10.3390/cells10092338.
- Calabrese EJ, Dhawan G, Kapoor R, Agathokleous E, Calabrese V. Hormesis: wound healing and fibroblasts. Pharmacol Res. 2022;184:106449–81. doi: 10.1016/j.phrs.2022.106449.
- Aljefri AM, Brien CO, Tan TJ, Sheikh AM, Ouellette H, Bauones S. Clinical applications of PRP: musculoskeletal applications, Current practices and update. Cardiovasc Intervent Radiol. 2023;46(11):1504–16. doi: 10.1007/s00270-023-03567-y.
- Graiet H, Lokchine A, Francois P, Velier M, Grimaud F, Loyens M, Berda-Haddad Y, Veran J, Dignat-George F, Sabatier F. et al. Use of platelet-rich plasma in regenerative medicine: technical tools for correct quality control. BMJ Open Sport Exerc Med. 2018;4(1):e000442. doi: 10.1136/bmjsem-2018-000442.
- Gilat R, Haunschild ED, Knapik DM, Evuarherhe A Jr., Parvaresh KC, Cole BJ. Hyaluronic acid and platelet-rich plasma for the management of knee osteoarthritis. Int Orthop (SICOT). 2021;45(2):345–54. doi: 10.1007/s00264-020-04801-9.
- van der Bijl I, Vlig M, Middelkoop E, de Korte D. Allogeneic platelet-rich plasma (PRP) is superior to platelets or plasma alone in stimulating fibroblast proliferation and migration, angiogenesis, and chemotaxis as relevant processes for wound healing. Transfusion. 2019;59(11):3492–500. doi: 10.1111/trf.15535.
- Miroshnychenko O, Chang WT, Dragoo JL. The use of platelet-rich and platelet-poor plasma to enhance differentiation of skeletal myoblasts: implications for the use of autologous blood products for muscle regeneration. Am J Sports Med. 2017;45(4):945–53. doi: 10.1177/0363546516677547.
- Paget LDA, Reurink G, de Vos RJ, Weir A, Moen MH, Bierma-Zeinstra SMA, Stufkens SAS, Goedegebuure S, Krips R, Maas M. et al. Platelet-rich plasma injections for the treatment of ankle osteoarthritis. Am J Sports Med. 2023;51(10):2625–34. doi: 10.1177/03635465231182438.
- Boivin J, Tolsma R, Awad P, Kenter K, Li Y. The biological use of platelet-rich plasma in skeletal muscle injury and repair. Am J Sports Med. 2023;51(5):1347–55. doi: 10.1177/03635465211061606.
- Andia I, Abate M. Platelet-rich plasma: combinational treatment modalities for musculoskeletal conditions. Front Med. 2018;12(2):139–52. doi: 10.1007/s11684-017-0551-6.
- Mastrogiacomo M, Nardini M, Collina MC, Di Campli C, Filaci G, Cancedda R, Odorisio T. Innovative cell and platelet rich plasma therapies for diabetic foot ulcer treatment: the allogeneic approach. Front Bioeng Biotechnol. 2022;10:869408. doi: 10.3389/fbioe.2022.869408.
- He M, Chen T, Lv Y, Song P, Deng B, Guo X, Rui S, Boey J, Armstrong DG, Ma Y. et al. The role of allogeneic platelet-rich plasma in patients with diabetic foot ulcer: Current perspectives and future challenges. Front Bioeng Biotechnol. 2022;10:993436. doi: 10.3389/fbioe.2022.993436.
- Vladulescu D, Scurtu LG, Simionescu AA, Scurtu F, Popescu MI, Simionescu O. Platelet-rich plasma (PRP) in dermatology: cellular and molecular mechanisms of action. Biomedicines. 2024;12(1):7. doi: 10.3390/biomedicines12010007.
- Zhu L, Li P, Qin Y, Xiao B, Li J, Xu W, Yu B. Platelet-rich plasma in orthopedics: bridging innovation and clinical applications for bone repair. J Orthop Surg. 2024;32(1):10225536231224952. doi: 10.1177/10225536231224952.
- Tang R, Wang S, Yang J, Wu T, Fei J. Application of platelet-rich plasma in traumatic bone infections. Expert Rev Anti Infect Ther. 2021;19(7):867–75. doi: 10.1080/14787210.2021.1858801.
- Rodrigues RM, Valim VS, Berger M, da Silva APM, Fachel FNS, Wilke II, da Silva WOB, Santi L, da Silva MAL, Amorin B. et al. The proteomic and particle composition of human platelet lysate for cell therapy products. J Cell Biochem. 2022;123(9):1495–505. doi: 10.1002/jcb.30310.
- Fortunato TM, Beltrami C, Emanueli C, De Bank PA, Pula G. Platelet lysate gel and endothelial progenitors stimulate microvascular network formation in vitro: tissue engineering implications. Sci Rep. 2016;6(1):25326. doi: 10.1038/srep25326.
- Astori G, Amati E, Bambi F, Bernardi M, Chieregato K, Schäfer R, Sella S, Rodeghiero F. Platelet lysate as a substitute for animal serum for the ex-vivo expansion of mesenchymal stem/stromal cells: present and future. Stem Cell Res Ther. 2016;7(1):93. doi: 10.1186/s13287-016-0352-x.
- Guiotto M, Riehle MO, Raffoul W, Hart A, di Summa PG. Is human platelet lysate (hPL) the ideal candidate to substitute the foetal bovine serum for cell-therapy translational research? J Transl Med. 2021;19(1):426. doi: 10.1186/s12967-021-03104-w.
- Hosseini S, Soltani-Zangbar MS, Zamani M, Yaghoubi Y, Rikhtegar Ghiasi R, Motavalli R, Ghassabi A, Iranzad R, Mehdizadeh A, Shakouri SK. et al. Comparative evaluation of autologous platelet-rich plasma and platelet lysate in patients with knee osteoarthritis. Growth Factors. 2023;41(3):165–77. doi: 10.1080/08977194.2023.2227273.
- Potter N, Westbrock F, Grad S, Alini M, Stoddart MJ, Schmal H, Kubosch D, Salzmann G, Kubosch EJ. Evaluation of the influence of platelet-rich plasma (PRP), platelet lysate (PL) and mechanical loading on chondrogenesis in vitro. Sci Rep. 2021;11(1):20188. doi: 10.1038/s41598-021-99614-0.
- Lykov AP, Bondarenko NA, Surovtseva MA, Kim II, Poveshchenko OV, Pokushalov EA, Konenkov VI. Comparative effects of platelet-rich plasma, platelet lysate, and fetal calf serum on mesenchymal stem cells. Bull Exp Biol Med. 2017;163(6):757–60. doi: 10.1007/s10517-017-3897-5.
- Klatte-Schulz F, Schmidt T, Uckert M, Scheffler S, Kalus U, Rojewski M, Schrezenmeier H, Pruss A, Wildemann B. Comparative analysis of different platelet lysates and platelet rich preparations to stimulate tendon cell biology: an in vitro study. Int J Mol Sci. 2018;19(1):212. doi: 10.3390/ijms19010212.
- Merolle L, Iotti B, Berni P, Bedeschi E, Boito K, Maurizi E, Gavioli G, Razzoli A, Baricchi R, Marraccini C. et al. Platelet-rich plasma lysate for treatment of eye surface diseases. J Vis Exp. 2022;2022(186). doi: 10.3791/63772.
- Chen CF, Wang PF, Liao HT. Platelet-rich plasma lysate enhances the osteogenic differentiation of adipose-derived stem cells. Ann Plast Surg. 2024;92(1S Suppl 1):12–20. doi: 10.1097/SAP.0000000000003765.
- Bindal P, Gnanasegaran N, Bindal U, Haque N, Ramasamy TS, Chai WL, Kasim NH. Angiogenic effect of platelet-rich concentrates on dental pulp stem cells in inflamed microenvironment. Clin Oral Investig. 2019;23(10):3821–31. doi: 10.1007/s00784-019-02811-5.
- Kelly K, Cancelas JA, Szczepiorkowski ZM, Dumont DF, Rugg N, Dumont LJ. Frozen platelets—development and future directions. Transfus Med Rev. 2020;34(4):286–93. doi: 10.1016/j.tmrv.2020.09.008.
- Beitia M, Delgado D, Mercader J, Gimeno I, Espregueira-Mendes J, Aizpurua B, Sánchez M. The effect of short-term cryopreservation on the properties and functionality of platelet-rich plasma. Platelets. 2023;34(1):2210243–52. doi: 10.1080/09537104.2023.2210243.
- Harrison P, Alsousou J. The state of the art and future of PRP therapy. Platelets. 2021;32(2):150–1. doi: 10.1080/09537104.2020.1869718.
- cHuish S, Green L, Kempster C, Smethurst P, Wiltshire M, Prajapati C, Allen E, Cardigan R. A comparison of platelet function in cold‐stored whole blood and platelet concentrates. Transfusion. 2021;61(11):3224–35. doi: 10.1111/trf.16657.