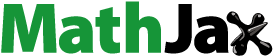
ABSTRACT
Paper-based lateral flow immunoassays (LFIAs) are an interesting on site technique for detecting heavy metals. Pb(II) is one of the toxic heavy metals in polluted environmental water. This paper reports the synthesis and characterization of three Fe3O4-based nanoadsorbents that show different performance to Pb(II), Cu(II), Cd(II), Hg(II), As(V), Zn(II), Fe(III), Ca(II), Mg(II), Mn(II), and Al(III) when a GO or an amino group is introduced. Compared with Fe3O4, Fe3O4-GO showed higher adsorption percentage to many heavy metal ions under slightly acid condition. The negative surface on Fe3O4@NH2 had favourable interactions for four main divalent metal ion adsorption, which followed the order: Pb(II) > Cu(II) > Hg(II) > Cd(II). Combined with antibody-functionalized AuNPs showed high affinity and specificity toward Pb(II), an Fe3O4@NH2-based LFIA with five times greater sensitivity for detecting Pb(II) in lake water was developed. The cut-off value of this Fe3O4@NH2-combined LFIA method for detection Pb(II) in lake water was 20 ng/mL and the detection result for 10 ng/mL Pb(II) was obviously observable by the naked eye or a strip reader machine. This detection strategy could be readily adapted for other small molecules or biomolecules as an ultrasensitive screening tool.
Introduction
Nowadays, uncontrolled and deliberate discharge of heavy metal ion-containing pollutants has become a major problem for the environment. Heavy metals such as lead, mercury, cadmium, and arsenic have steadily been accumulating in the environment, in water, and in soil. Removing these heavy metals from natural waters has become a great challenge because of their adverse effects on environment ecosystems and human health (Peng, Li, Liu, & Song, Citation2017).
Various technologies have been explored to eliminate the negative effects of heavy metals on natural systems, such as ion exchange, membrane separation, flocculation, ultrafiltration, chemical precipitation, neutralization, and bioremediation strategies by specialists (Zou et al., Citation2016). Most of these methods need expensive equipment and are time-consuming and difficult to apply in the field. Development of novel, economical, robust, and high efficiency adsorbents remains a continuing area of research to satisfy large-scale water treatment requirements.
One interesting type of adsorbent for this purpose is magnetic nanoparticles and magnetic nanoparticle-based composites, for example, magnetic nanoparticle graphene oxide composites or magnetic nanoparticle core–shell composites. Graphene is a honeycomb-like one atom thick carbon lattice that has attracted attention for its large adsorption capacity for heavy metals (Cui et al., Citation2015; Ren et al., Citation2016; Vilela, Parmar, Zeng, Zhao, & Sanchez, Citation2016). By oxidation of natural graphite, polar GO can be synthesized with lamellar surfaces and attached oxygenated functional groups (C = O, C–O, –OH, and epoxy). These functional groups, when distributed on the GO surface and edges, make GO sheets strongly hydrophilic and dispersible in water. Because of its large specific surface area, GO, as a superb host, has been functionalized with numerous materials (Perreault, Fonseca de Faria, & Elimelech, Citation2015; Stankovich et al., Citation2006). However, recycling of GO suffers from its high dispersion characteristics in water.
Because of their characteristics of paramagnetism, biocompatibility, and safety, Fe3O4 nanocrystals and their GO composites have been seen as an easy, low cost, and environmentally friendly strategy for adsorbing, separating, and recovering heavy metals from water (Peng et al., Citation2017; Xin et al., Citation2012; Zhang et al., Citation2013a; Zhang, Wu, Dong, Li, & Pan, Citation2017b). The reported Fe3O4-based GO composites include nano Fe3O4@GO/PS (Kassaee, Motamedi, & Majdi, Citation2011), GO-Fe3O4-DXR (Yang et al., Citation2009), GO-Fe3O4 (Zubir, Yacou, Motuzas, Zhang, & Diniz da Costa, Citation2015), EDTA-mGO (Cui et al., Citation2015), Ppy-Fe3O4/rGO (Wang et al., Citation2015), RGO-Fe3O4 (Chandra et al., Citation2010), GO/ Fe3O4 composites (Li et al., Citation2012; Zhao et al., Citation2016), RGO/PEI/ Fe3O4 (Wang et al., Citation2017a) and so on (Pan et al., Citation2017). This new class of graphene-based composites has significant properties in the fields of MR imaging (Cong, He, Lu, & Yu, Citation2010), protein separation, electron conduction (Su, Cao, Ren, & Hu, Citation2011; Zhou, Song, Ma, & Chen, Citation2011), catalytic oxidation (Dong et al., Citation2012), and organic pollutant removal (Geng et al., Citation2012; Han et al., Citation2012; Zubir et al., Citation2015). For example, Chen et al. prepared a GO-Fe3O4 hybrid by chemical deposition and then loaded it with doxorubicin hydrochloride (DXR) to obtain controlled targeted drug carriers (Yang et al., Citation2009). Ye et al. developed a controlled method by adding Fe(acac)3 in 1-methyl-2-pyrrolidone dropwise to an EGO/1-methyl-2-pyrrolidone solution and found through Fourier transform infrared spectroscopy that metal-carbonyl coordination offered a tight and robust linkage between magnetite and GO through Fourier transform infrared spectroscopy (Shen et al., Citation2010). Yu et al. synthesized PSS-coated reduced GO sheets that were then decorated in situ with Fe3O4 in triethylene glycol solution (Cong et al., Citation2010). One of their most important applications was heavy metal removal. Kim et al. found that magnetite-reduced graphene oxide composites (Fe3O4-RGO) showed over 99.9% removal for 1 ppb As(III) and As(V); As(V) anions were largely adsorbed even at low pH (Chandra et al., Citation2010). Chen et al. prepared GO/Fe3O4 composites and a ternary magnetic composite to evaluate sorption performance toward Cu(II) and Cr(VI) (Li et al., Citation2012; Zhao et al., Citation2016). Du et al. synthesized EDTA-functionalized magnetic graphene oxide (EDTA-mGO) and found that metal chelation by EDTA and the electrostatic attractions of the abundant oxygenated functional groups on the GO surface played an important role in adsorbing Pb(II), Hg(II), and Cu(II) (Cui et al., Citation2015). Yuan et al. fabricated polypyrrole-decorated Fe3O4/rGO composites through in situ polymerization and indicated that concentration and pH had a significant influence on removing Cr(VI), which was reduced to low toxicity Cr(III) with the aid of Ppy nitrogen groups (Wang et al., Citation2015). Ding et al. synthesized Fe3O4/GO, a novel magnetic nanocomposite, based on a one step chemical coprecipitation method. The composite showed higher adsorption capacity for Cu(II) and Pb(II) at high pH due to its strong complexation ability with carboxylic groups (Sun, Liang, Han, Zhang, & Ding, Citation2015). Most studies in this field have focused on material characterization, dosage, pH effect, the adsorption isotherm test, and the kinetic adsorption and regeneration test. The adsorption capacity and adsorption performance of heavy metal ions were improved, and separation of GO from water was also facilitated.
Although Fe3O4 and Fe3O4 composites have aroused considerable interest because of the ease of separation they offer, they suffer from a tendency to aggregate and decompose in acid-regenerated solution. To protect magnetic nanoparticles from aggregation or dissolution under low pH, it is recommended to stabilize Fe3O4 with a silica shell by surface modification. Some applicable functional groups such as NH2 or SH can be introduced solidly and tightly through a simple silanization method (Zhang et al., Citation2013a; Zhang et al., Citation2013b).
However, only a few studies have investigated the adsorption behaviour of Fe3O4-based adsorbents (i.e. Fe3O4, Fe3O4-GO, Fe3O4@NH2) in solutions of multiple metal ions, including Pb(II), Hg(II), Cu(II), Zn(II), As(V), and Cd(II). Importantly, until now, there has been no application of these nanomaterials for developing a low cost, environmentally friendly, and portable antibody-functionalized AuNPs based heavy-metal LFIA test, a rapid and widespread on-site detection tool (Cheng, Yang, Ni, Peng, & Lai, Citation2017; Deng, Yang, Wu, Zhang, & Jiang, Citation2018; Peng et al., Citation2018; Wang et al., Citation2017b; Xing, Jing, Zhang, & Yuan, Citation2017); most applications have been for instrument analysis.
In this study, Fe3O4, Fe3O4-GO, and Fe3O4@NH2 were prepared. The morphologies, structures, and properties of composites were characterized by TEM, FTIR, XRD, and other methods. Adsorption and desorption experiments were performed, and the results fit the pseudo second-order kinetic model. Their performance to Pb(II), Cu(II), Cd(II), Hg(II), As(V), Zn(II), Fe(III), Ca(II), Mg(II), Mn(II), and Al(III) were evaluated when a GO or an amino group is introduced. Finally, Fe3O4@NH2 was selected to enrich Pb(II) and has been successfully used to develop LFIA, a fast onsite method for detecting Pb(II) in lake water.
Materials and methods
Chemicals and materials
Graphite powder was purchased from Sinopharm Chemical Reagent Co. Ltd. Graphene oxide was synthesized based on a modified Hummer’s method (Wang et al., Citation2013). 3-aminopropyl trimethoxysilane (APTMS) and tetraethyl orthosilicate (TEOS) were purchased from Aladdin Chemistry Co., Ltd., China. Milli-Q deionized water was used in all experiments. Other reagents including potassium dichromate (K2Cr2O7), potassium permanganate (KMnO4), hydrogen peroxide (H2O2, 30%), sodium acetate (NaAc), and iron (III) chloride hexahydrate (FeCl3·6H2O) were of analytical reagent grade.
Synthesis of Fe3O4
Fe3O4 nanoparticles were prepared using the co-precipitation method with some modifications (Fried, Shemer, & Markovich, Citation2001). 1.736 g NH4Fe(SO4)2·12H2O and 0.784 g (NH4)2Fe(SO4)2·6H2O were added to 100 mL deaerated ultrapure water. The aqueous solution was heated to 80°C under vigorous and constant agitation for 30 min. To precipitate Fe2+ and Fe3+ ions for synthesis of magnetite particles, an ammonia solution was used. 20 mL of 1 M NH4OH was added dropwise, and the reaction was maintained for an additional 30 min. The colour changed from light red to reddish-brown and finally black. N2 was used as the protective gas throughout the experiment. The solution was cooled to room temperature. The reaction product, a black precipitate, was separated by an external magnetic field and washed with ethanol and deionized water for several cycles. Finally, the Fe3O4 nanoparticles were dried by vacuum freezing.
Synthesis of Fe3O4-GO
First, graphene oxide was prepared from graphite according to a modified Hummer’s method. Then Fe3O4-GO was synthesized by a chemical co-precipitation method with some modifications (Sun et al., Citation2015; Zubir et al., Citation2015). 1.736 g NH4Fe(SO4)2·12H2O and 0.784 g (NH4)2Fe(SO4)2·6H2O were added to 100 mL deaerated ultrapure water. The aqueous solution was heated to 80°C under vigorous and constant agitation for 30 min. To prevent GO sheets from stacking together at low pH, 1 M NH4OH was added dropwise to the reaction to maintain pH 3.0. Then 23 mg synthesized GO dispersed in 30 mL high purity water was ultrasonicated for 2 h to obtain a clear dispersion of GO and added to a solution of iron ions for another 30 min of agitation. The solution pH was adjusted to 10.0 by adding 1M NH4OH dropwise. The mixture was reacted for 1 h at 80°C. N2 was used as the protective gas throughout the experiment. The solution was cooled to room temperature. The reaction product was separated by an external magnetic field and washed with ethanol and deionized water for several cycles. Finally, the Fe3O4-GO composites were dried by vacuum freezing.
Synthesis of Fe3O4@NH2
Magnetic Fe3O4@NH2 was prepared by a solvothermal method and modified sequentially with TEOS and APTMS to graft amine groups, as previously reported (Liu et al., Citation2009; Zhang et al., Citation2013a). Briefly, FeCl3·6H2O (1.62 g), Na3Cit (1.5 g), and NaOAc (4.0 g) were dissolved in ethylene glycol (60 mL). After vigorous stirring for 2 h, a yellow solution was obtained and was transferred to a Teflon-lined stainless-steel autoclave (100 mL). The solution was sealed and heated at 200°C for reaction for 12 h. The autoclave was cooled to room temperature, and the obtained Fe3O4 was washed several times with water and ethanol. Finally, the products were collected with a magnet and then redispersed in 80 mL water for further introduction of amine groups.
Typically, 40 mL obtained Fe3O4 was diluted by 300 mL ethanol, and the mixture was homogenized by ultrasonication for 15 min. 2 mL NH3·H2O was added, and the solution was stirred vigorously for 30 min at room temperature. 1.0 mL TEOS was dropped into the solution, and stirring was performed for 45 min. 0.01–1.0 mL APTMS was introduced and reacted for 4 h. The synthesized materials were washed several times with water and ethanol and dried by vacuum freezing before use.
Characterization
The morphologies of as-prepared Fe3O4 (prepared by the co-precipitation method) and of Fe3O4-GO and Fe3O4@NH2 (prepared by the solvothermal method) were determined with transmission electron microscopy (TEM, JEM-200CX, JEOL, Japan). Fourier transform infrared (FT-IR) spectra were recorded with resolution 1 cm−1 in the 400–4000 cm−1 region using a Bruker Tensor 27 FTIR spectrometer. The Pb(II) concentration was analyzed using an ICP-MS spectrophotometer (Hitachi Limited, Japan).
Adsorption and desorption experiments
Stock standard solutions (1000 mg/L) including Pb(II), Cu(II), Cd(II), Hg(II), As(V), Zn(II), Fe(III), Ca(II), Mg(II), Mn(II), and Al(III) were purchased from the National Research Center for Certified Reference Materials (Beijing, China). Freshly prepared solutions containing 40 ng/mL Pb(II), Cu(II), Cd(II), Hg(II), As(V), Zn(II), Mg(II), Mn(II), and Al(III), 200 ng/mL Fe(III), and 2,000 ng/mL Ca(II) were used in each experiment.
The adsorbents (10 mg) were homogenized and added to 40 mL of fresh prepared aqueous sample solution containing multiple metal ions with adjusted pH ranging from 2.0 to 8.0. The tube was mixed by an analog rotisserie tube rotator (SCILOGEX MX-RL-E) for 2–110 min. After the adsorption process had finished, the Fe3O4, Fe3O4-GO, and Fe3O4@NH2 were gathered by an external magnetic field. The settled solution was collected and tested for unadsorbed concentrations of heavy metals in the supernatant. The heavy metal concentrations were determined by ICP-MS with various dilution ratios. The experiments were all done three times, and the average values were used. The amounts of Pb(II), Cu(II), Cd(II), Hg(II), and As(V) adsorbed onto Fe3O4, Fe3O4-GO, and Fe3O4@NH2 and the removal efficiency were calculated by the following equations:
(1)
(1)
(2)
(2) where C0 and Ce (ng/mL) are the initial and equilibrium heavy metal concentrations respectively, M (mg) is the mass of adsorbent, V (mL) is the volume of adsorption solution, and qt (mg/g) is the adsorbed amount of heavy metals per unit mass of adsorbent at time t.
In the desorption and regeneration experiments, HCl was used as the desorbing agent. 1 mL of the above three desorbing agents at different concentrations were mixed with Fe3O4, Fe3O4-GO, and Fe3O4@NH2 and shaken for 5–30 min at room temperature. Through magnetic separation, the eluent was separated from the gathered magnetic particles, which were washed three times with ultrapure water. The concentrations of desorbed heavy metal ions were determined by ICP-MS.
Analysis of Pb(II) in lake water combining magnetic materials with LFIA
Pb(II) was used as a model ion to illustrate the detection system of magnetic material-based heavy metal LFIA as showed Scheme 1. 20 ng/mL Pb(II) was spiked into lake water and adsorbed by Fe3O4@NH2 (or Fe3O4, Fe3O4-GO). The pH was adjusted to 7. A certain amount of adsorbent was added to 5 mL lake water solution and mixed fully to make sure that Pb(II) were completely adsorbed. Then an external magnetic field was used to isolate the adsorbent. The separated magnetic precipitates were collected and eluted by 0.02 M HCl. Then the strip was used to draw the eluted solution, which was prepared according to the previous method (Xing et al., Citation2015). Typically, 180 µL sample solution was mixed with 20 µL 0.1 M Tris pH 7.5 containing 1 mM ITCBE, which formed complexes with Pb(II). The mixture was added to freeze-dried AuNP-labeled antibody in the micro-wells. Then a prepared strip, composed of a nitrocellulose membrane, a sample pad, and an adsorbent pad, was used to draw the solution up the strip to the test line and the control line. The reaction was stopped in 5 min and could be easily read by eye or using a mobile strip reader. The relative intensities of the test line and the control line will changed with Pb(II) concentration. The effects of interfering ions, solid-to-liquid ratio, contact time, and eluent were assessed for best performance.
Results and discussion
Characterization
The co-precipitation method was used to obtain Fe3O4 particles in alkaline medium. A shows some typical electron micrographs. The particles deposited from the aqueous suspension in the 25–30 nm range were formed with broad size distributions. Fe3O4-GO composite was formed according to previous reports (Cong et al., Citation2010; Zubir et al., Citation2015) and successfully prepared based on a one step synthesis method by an optimized process. The detailed distribution of the Fe3O4 particles on GO sheets was further revealed. In the primary phase of the reaction, carboxylate anions on the GO surface captured Fe3+ and Fe2+ ions before the ammonia solution was added. B and C clearly show that, unlike the pristine GO sheets, the Fe3O4 particles were coated on the GO. The Fe3O4-GO composite was washed six times, and no aggregated or free particles were detected; the distribution of magnetite particles on the GO sheet was also uniform. Magnetite particles modified with NH2 and protected by SiO2 were synthesized by a modified solvothermal reaction at 200°C. As shown in D, TEM revealed that the prepared nanoparticles had a nearly uniform size of about 100 nm and a spherical shape. SiO2 was simultaneously and successfully coated onto the surface of the Fe3O4 loading of amino functionality. A higher magnification TEM image confirmed that the SiO2 on the surface of the Fe3O4@NH2 was about 10 nm thick (E and F).
Figure 1. Characterization of as-prepared adsorbent: TEM images of Fe3O4 prepared by co-precipitation method (A), GO (B), Fe3O4-GO (C), Fe3O4 prepared by solvothermal method (D), and Fe3O4@NH2 (E, F).
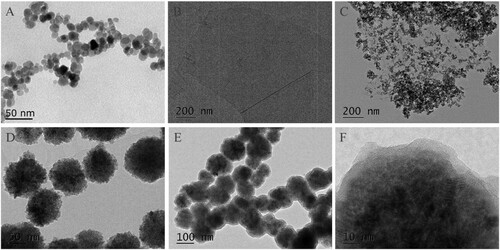
Fe3O4 and its composites obtained by various methods showed the typical X-ray diffraction (XRD) patterns of magnetite. Figure S1A shows the XRD patterns of Fe3O4, GO, Fe3O4-GO, and Fe3O4@NH2, which confirmed the phase and structure of the synthesized samples. Compared with previous reports on crystallin magnetic nanoparticles, the Fe3O4 XRD pattern exhibited six distinct and identical peaks. The diffraction peaks of Fe3O4 at 2θ values of 30.1°, 35.7°, 43.0°, 53.8°, 57.2°, 62.8°, and 74.3° indexed to the (220), (311), (400), (422), (511), (440), and (533) lattice planes compared to the Jade PDF card (89-4319). This indicated that Fe3O4 had a cubic crystal system. Corresponding to the (002) reflection, a very sharp diffraction peak at 10.02° identified the GO. No obvious diffraction peak attributed to graphite was observed in the spectrum of the Fe3O4-GO composites because of the disordered stacking of graphene sheets (Cong et al., Citation2010). As for the presence of amorphous silica components, the XRD pattern of Fe3O4@NH2 showed an extra band around 2θ = 23°, which was consistent with the TEM results.
Figures S1(B) and S1(C) show the FTIR spectra of GO, Fe3O4, Fe3O4-GO, and Fe3O4@NH2. In the GO spectrum, as shown in Figure S1(B), the intense band at ∼3423 cm−1 was attributed to the O-H stretching vibration of the carboxylic acid groups of GO. The peak at 1631 cm−1 could be assigned to the skeletal vibrations of the C = O bond originating from carboxyl functional groups. Deformation of the C–O band was observed in the band at 1091 cm−1. The peak at 584 cm−1 was assigned to the Fe-O bond vibration of Fe3O4. Comparing the FTIR spectra of GO with those of the Fe3O4-GO composite, the FTIR spectrum of the Fe3O4-GO composite owned the new band at 587 cm−1, which can be attributed to the Fe-O bond vibration of Fe3O4 in the Fe3O4-GO composite. This band obviously differed from that of GO. In addition, the bands at 897 cm−1 and 1381 cm−1 supported the hypothesis that the Fe3O4 NPs were covalently bonded to GO.
Figure S1(C) shows Fe3O4 prepared by the solvothermal method, where typical bands at around 592 cm−1 were assigned to Fe-O stretching and bands at around 3400 cm−1 were ascribed to O-H vibrations. The bands at 1626 cm−1 and 1381 cm−1 were associated with carboxylate groups. As for Fe3O4@NH2, on the surface of the Fe3O4 cores, SiO2 and aminopropyl were also inspected by FT-IR experiments to verify their successful coating, as seen in Figure S1(C). Except for the broad bands at around 3400 cm−1 and the typical bands at 592 cm−1 in both samples, the Si-O-Si asymmetric stretching vibration owned the characteristic peak of 1066 cm−1, which indicated the formation of silica shells on the surface of Fe3O4. The bands corresponding to the N-H stretching vibration were not easily distinguishable because of a possible hindrance by OH stretching from water (Zhu et al., Citation2018). The FTIR spectrum confirmed that the prepared Fe3O4, Fe3O4-GO, and Fe3O4@NH2 contained plentiful oxygen and nitrogen functional groups, which could act as available adsorption sites in the heavy metal ion adsorption process.
Batch adsorption experiments
The optimal solution pH for various heavy metal ion adsorbent systems may be different due to variations in the standard reduction potential of the heavy metal ions, the metal electronegativity, the first stability constant of the associated metal hydroxide, and the atomic structure of the adsorbents. Different adsorbents have slightly different optimal pH values. For Fe3O4, the optimal pH for adsorbing Pb(II) was around 5.5 (Nassar, Citation2010), whereas it was 4.2 for EDTA-functionalized magnetic graphene oxide (EDTA-mGO) (Cui et al., Citation2015) and about 6 for Fe3O4@SiO2-NH2 MNPs (Zhang et al., Citation2013a). In the adsorption process, the matrix solution pH was a significant aspect because it determined the solubility of metal ions, the concentration of counter ions on the functional groups of the adsorbent, and the degree of ionization of the adsorbent (Amuda, Giwa, & Bello, Citation2007).
To investigate the effect of pH on multi-element adsorption behaviour, the solution pH was adjusted with HCl and NaOH solution in the 2–8 range. Multi-element [including Pb(II), Cu(II), Cd(II), Hg(II), and As(V)] adsorption experiments were performed in 50 mL polypropylene bottles containing 40 mL aqueous solution for 30 min. The sorbent concentration was kept at 0.25 g/L with 50 mg/L NaCl for ionic strength adjustment purposes.
Effect of pH on multi-element adsorption by Fe3O4
Experiments in this study showed, as illustrated in A and B, that the adsorption effect of As(V) still existed even at pH 2 and that the removal efficiencies of bare Fe3O4 and Fe3O4-GO both showed near complete (over 98%) arsenic removal within 40 ppb. Similarly, it has been observed that acid conditions are favourable for As(V) adsorption onto iron oxide (Dixit & Hering, Citation2003). As-Fe shells formed because of strongly adsorbed and coprecipitated As(V) on iron oxide products (Kanel, Manning, Charlet, & Choi, Citation2005; Manning, Hunt, Amrhein, & Yarmoff, Citation2002). For Pb(II), Cd(II), Cu(II), and Hg(II), the surface of Fe3O4 adsorbents may also undergo protonation/deprotonation with pH change, as described in the following equation:
where OH− and H+ refer to the potential determining ions.
In the bare Fe3O4 adsorption process, as shown in A, the adsorption percentage of Pb(II) was approximately 94.8% around pH 6. At lower pH values, the adsorption percentage was approximately 20% around pH 3. As for the pH effect on adsorption of Pb(II), Cd(II), Cu(II), and Hg(II), the adsorption amounts were all increased at higher pH (Cui et al., Citation2015; Liu, Zhao, & Jiang, Citation2008; Madadrang et al., Citation2012; Zhang et al., Citation2013a; Zhao et al., Citation2011). Electrostatic attraction may play an important role for Fe3O4 in the adsorption process. For example, in the pH range from 2.0 ± 0.1 to 6.0 ± 0.1, the dominant form of Pb(II) was positively charged Pb(II) species. When the pH values were higher (>7), other Pb(II) species including Pb(OH)+ and Pb(OH)2 were usually present. Adsorption of Pb(OH)+ and precipitation of Pb(OH)2 may occur at the same time in the process of Pb(II) removal (Zhao et al., Citation2011). In a basic solution, the surface of the adsorbent existed mainly in the form of M-O−, and the negatively charged surface had a significantly high electrostatic attraction to Pb(II). As the solution became more acidified, except for competitive adsorption existing between Pb2+ and H3O+ with adsorption site groups, the increasing number of positively charged sites (M-OH2+) led to a reduction in the number of negatively charged sites, which counteracted the adsorption of Pb(II) ions due to electrostatic repulsion.
Effects of pH on multi-element adsorption by Fe3O4-GO
In the case of Fe3O4 decorated with GO as shown in B, when the pH was increased from 2 to 5, the removal efficiency increased due to the deprotonation effect. Fe3O4-GO composite showed excellent performance compared to pure Fe3O4. Fe3O4@GO had higher adsorption percentages for Pb(II), Cd(II), Cu(II), and Hg(II) than did Fe3O4 because of the additional activity sites provided by GO. Compared with Fe3O4, Fe3O4-GO showed higher adsorption percentage to many heavy metal ions under slightly acid condition, as shown in B. At a pH of about 4, 92.63% of Pb(II), 59.45% of Cu(II), 55% of Hg(II), and 28.54% of Cd(II) were adsorbed by Fe3O4-GO; the corresponding efficiencies for Fe3O4 were 73.19% for Pb(II), 3.85% for Cu(II), 18.16% for Hg(II), and 7.77% for Cd(II). On the surfaces of graphene oxide, the abundant oxygen-containing functional group nanosheets offered additional strong surface complexation to divalent cations (Peng et al., Citation2017). GO has high affinity for multiple metal ions through surface complexation; this affinity follows the order: Pb(II) > Cu(II) Cd(II), which was consistent with the results obtained here (Gu & Fein, Citation2015). The inner sphere surface complexes of an aqueous metal cation (Mm+) on deprotonated surface sites on the GO were expressed by the following reaction:
where R represents the surface of GO to which each functional group type Si is attached.
Effects of pH on multi-element adsorption by Fe3O4@NH2
On the surface of Fe3O4@NH2, SiO2 was perfectly coated and formed a protective layer, as can be observed from the TEM image. In C, negative ions [As(V)] showed no adsorption under acid conditions and very low removal efficiency under neutral and basic conditions because of this coating that prevented these ions from contacting the Fe3O4. C shows the variations in the amount of Pb(II) adsorbed to Fe3O4@NH2 with pH, which can be explained as follows. Competitive adsorption between Pb2+ and H3O+ with -NH2 groups and adsorption processes was dominated by acid–base interactions. At acid pH, numerous H3O+ ions, commonly several times the number of the Pb(II) ions, covered the surface, leading to less Pb(II) adsorption (Anbia & Lashgari, Citation2009). However, the Fe3O4@NH2 surface became negatively charged at alkaline pH, and the -NH2 groups were deprotonated. The negative surface had favourable interactions for four main divalent metal ion adsorption, which followed the order: Pb(II) > Cu(II) > Hg(II) > Cd(II). Amino functionalization of hollow silica nanospheres (NH2-SNHS) has been studied and showed improved adsorption capacity following a similar order: Pb(II) > Cd(II) > Ni(II) (Najafi, Yousefi, & Rafati, Citation2012). Acid–base conditions, electronegativity, and ionic radius may be responsible for the observed selectivity (Shahbazi, Younesi, & Badiei, Citation2011). The acid–base behaviour of the surface -NH2 groups can be illustrated as follows:
Therefore, the optimized adsorption pH for purposes of simultaneous adsorption of Pb(II), Cd(II), Cu(II), and As(V) was found to be 6.0–8.0 using Fe3O4-GO. As for selective adsorption of Pb(II), Fe3O4@NH2 showed the best performance among these three nanomaterials at pH 6.0–7.0. Insoluble divalent positive ion species will not be generated at pH < 7.2, especially when their concentration is less than their solubility limit (Li et al., Citation2017; Shahbazi et al., Citation2011).
Performance of Fe3O4, Fe3O4-GO, and Fe3O4@NH2 sorbents
Six divalent or trivalent cations, including Fe(III), Al(III), Zn(II), Mn(II), Mg(II), and Ca(II), that are commonly abundant in lake water were investigated to examine the performance of Fe3O4, Fe3O4-GO, and Fe3O4@NH2 sorbents for adsorbing interfering cations. Each of their nitrates was added to the solution at a concentration of 40 ng/mL [except 200 ng/mL for Fe(III) and 2000 ng/mL for Ca(II)] before sorbent addition.
As shown in D, all three materials exhibited very good adsorption for Fe(III) and Al(III) and no adsorption for Ca(II). For other metal ions, Fe3O4 showed Zn(II) removal efficiency of about 71% and almost no adsorption for Mn(II) and Mg(II). As for Fe3O4-GO, it showed increased removal efficiency, to 100%, 59%, and 24% for Zn(II), Mn(II), and Mg(II) respectively, indicating the advantage of GO for adsorption of these metal ions. Considering its high removal efficiency for Pb(II), Cu(II), Cd(II), Hg(II), and As(V), it can be stated that Fe3O4-GO showed a general adsorption ability for metal ions. On the contrary, Fe3O4@NH2 had a selectively for adsorption of Pb(II) from complex matrices, as shown in D. Its low removal efficiency for Cd(II) (∼51%) may be attributed to competitive binding from the other metal ions in the multi-element system used in this research (Xiong et al., Citation2015). This result was different from that for Cd(II) adsorption by the amino-functionalized Fe3O4@SiO2 core–shell magnetic nanomaterial (Wang et al., Citation2010). In neutral and basic solutions, formation of a metal complex M2+ bonded to two -NH2 is the favourite binding mode, as stated by Debbaudt et al. (Zhang et al., Citation2017a).
Adsorption kinetics
Adsorption kinetics is an important aspect of describing the adsorption rate of a molecule onto a surface. The kinetics of adsorption on Fe3O4, Fe3O4-GO, and Fe3O4@NH2 were determined by contact with a 100 mL solution containing Pb(II), Cu(II), Cd(II), Hg(II), and As(V) at pH 7 (the concentration of each metal ion was 40 ng/mL). Depending on initial metal concentration, most of the cations began to form a precipitate at pH 8-11. In this experiment, due to low concentration of the metal ions used, precipitation at pH ∼7 was insignificant. shows the uptake rate of these six metal ions at room temperature. The adsorption capacity was enhanced rapidly in the initial 5 min, slowed down between 5 and 20 min, and reached equilibrium at about 2 h.
Figure 3. Effect of contact time on adsorption on Fe3O4(A), Fe3O4-GO(B), and Fe3O4@NH2 (C); Pseudo-second-order model for adsorption of Pb(II) on Fe3O4, Fe3O4-GO, and Fe3O4@NH2(D).
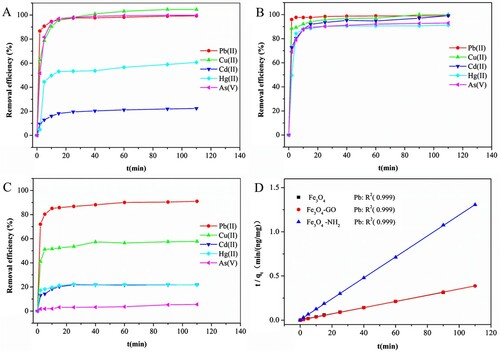
For all metal ions, 30 min was enough to attain adsorption equilibrium. For example, adsorption of Pb(II), Cu(II), and As(V) by Fe3O4 and Fe3O4-GO at pH 7 showed similar removal efficiencies (both greater than 98.6%), as shown in A–C, which was consistent with reported results (Liu et al., Citation2008). Compared with Fe3O4, Fe3O4-GO showed stronger adsorption for Cd(II). Different kinetic models, including the Elovich model, the Bangham model, the intraparticle diffusion model, pseudo first-order and pseudo second-order kinetic models, and Weber-Morris kinetic models were used to fit the experimental data to describe the multi-element adsorption system. In the three situations involving Fe3O4, Fe3O4-GO, and Fe3O4@NH2, the coefficients of determination (R2) from the pseudo-second-order model fitted to the data were higher than those from the other models.
Therefore, the pseudo second-order model was deemed most appropriate for describing the simultaneous behaviour of multiple heavy metal elements involved in chemical adsorption (Ngah & Fatinathan, Citation2010). The pseudo second-order equation can be written as follows:
(3)
(3) Rearrangement into the linear form based on integrating the equation for the boundary conditions (t = 0 to t = t and qt = 0 to qt = qt) gives:
(4)
(4) where qe (mg/g) and qt (mg/g) are the amounts of solute adsorbed at equilibrium and at time t (min) and k (mg/(g min) is the equilibrium rate constant. A slope of 1/qe and an intercept of
can be obtained by plotting t/qt against t, giving a linear relationship. Taking Pb(II) as an example, the measured kinetic data for Pb(II) adsorbed by these three Fe3O4-based nanoparticles all fitted well with the pseudo-second-order model, as shown in D. Figure S2 lists the fitting results for the other metal ions adsorbed by Fe3O4 and Fe3O4-GO.
Adsorption isotherms were constructed by varying the initial concentrations of Pb(II), Cu(II), Cd(II), Hg(II), and As(V) from 0.5 to 100 mg/L at pH 7 for 6 h. Table S1 shows the results (equilibrium adsorption capacity, qe, mg/g). They indicated that these materials were powerful heavy metal adsorbents. Given the abundant oxygen-containing functional groups on GO, the maximum adsorption capacities (Csmax) of Pb(II) ions reported at 293 K were 842 mg/g as obtained from the simulated Langmuir isotherm models on GO, which is 23 times higher than that of Pb(II) ions on iron oxide and twice that on graphene at the same experimental conditions (Zhao et al., Citation2011).
Stability and regeneration of adsorbent
Acid treatment is a common and feasible approach to regenerate heavy metal-loaded iron oxide. The first step was to determine the stability of Fe3O4, Fe3O4-GO, and Fe3O4@NH2 adsorbents under different acidic concentrations. The leached Fe contents were detected and are summarized in . For Fe3O4 and Fe3O4-GO under 1 M HCl conditions for 24 h, more than 86.6% of the Fe was leached, but only 5.23% of the amino-functionalized adsorbent. Enhanced stability of Fe3O4@NH2 under acidic conditions was observed.
Table 1. Fe content of different nanoparticles treated with acid solution.
High acid concentrations (i.e. more than 1 M HCl) could elute Pb(II) and lead to irreversible breakdown of Fe3O4. At the oxide-water interfaces for bare Fe3O4 and Fe3O4-GO, surface complexes of Pb(II) were found to be hydrolyzed and adsorbed as mononuclear bidentate complexes to the edges of Fe3O4 (Bargar, Brown, & Parks, Citation1997). In these bidentate edge-sharing geometries, Pb(II) was bonded to the iron oxide surfaces through Pb-O. Most surface species, for example Fe-(OH)-Pb, are stable and difficult to restore to their full adsorption capability. Fe-O will inevitably be destroyed if the regenerating solution is strong enough to break the Pb-O. On the contrary, weak acid achieves a weak elution rate. Regeneration of bare Fe3O4 and Fe3O4-GO suffers from unstable surfaces under acid conditions. In the stability experiment, 10 mg Fe3O4 were almost dissolved in the 1 M HCl solution after 24 h. For Fe3O4@NH2, 1 M HCl solution was used for regeneration and reused in three consecutive cycles. Using Pb(II) as an example, the adsorption capacity was 101.2 mg/g in the first use of sorbents, but decreased to around 96.2 and 92.5 mg/g in the second and third uses, respectively, indicating that adsorption was stable.
Use of Fe3O4@NH2 in strip sensor for sensitive detection of Pb(II) in lake water
In a previous study by the authors, thiol- and amine bifunctionalized mesoporous silica was synthesized by a one pot method and used for uptake and speciation of arsenic in combination with ICP-MS. 10 mL 100 ng/mL As(V) or As(III) sample solution was concentrated within 10 min and detected by ICP-MS after a sequential elution strategy. Easy operation and appropriate detection levels were achieved in a short time; however, there still involved expensive instruments (Li et al., Citation2014). Based on previous sensitive Pb(II) antibody screening, a rapid and highly sensitive LFIA has been developed for detection of Pb(II) in drinking water under 10 ng/mL (Kuang et al., Citation2013). In this research, Pb(II) was previously concentrated from lake water within the past 10 min and detected onsite by LFIA within 5 min based on a Pb(II) strip sensor.
Strip performance for detecting Pb(II) in drinking water and lake water
The heavy metal strip detection process has been described before (Xing et al., Citation2015; Xing, Liu, Zhang, Kuang, & Xu, Citation2014; Xu et al., Citation2016). As shown in Figure S3, when the concentration of Pb(II) in drinking water was greater than 10 ng/mL, the test line on strip 7 disappeared, which could be determined by the naked eye. Pb(II)-ITCBE-antibody–AuNP was formatted, which could not be captured by Pb(II)-ITCBE-BSA on the test line.
However, the sensitivity of the Pb(II) strip for detecting lake water was less. The cut-off value was 100 ng/mL for inspection by the naked eye, as shown in . This method was disabled for detection of trace levels of Pb(II) (<10 ng/mL) in lake water. Many interfering ions and organic matter are also present in lake water. Microelements, including 88.9 µg/mL Ca(II), 6.12 µg/mL Mg(II), 0.057 µg/mL Zn(II), 0.042 µg/mL Fe(II), 0.02 µg/mL Al(II), and 0.019 µg/mL Mn(II), were found in the water. The Pb(II) concentration, ∼0.2 ng/mL in lake water, was confirmed by ICP-MS. Other toxic heavy metals, including As(V), Hg(II), Cd(II), and Cu(II), were all below 1 ng/mL.
Use of Fe3O4@NH2 for highly sensitive Pb(II) detection in lake water
Based on the above experimental data, Fe3O4@NH2 had several advantages for developing a fast field detection method. First, the adsorption equilibrium time was short when the initial metal ion concentrations was low. In this condition, the available metal ions were more rapidly adsorbed to the adsorption site (Madadrang et al., Citation2012). Secondly, Fe3O4@NH2 decreased interference from other cations, including Cd (II), Hg(II), Zn(II), Mg(II), Mn(II), Fe(III), and Ca(II). The adsorbed Pb(II) could be easily eluted by weak acid solution (0.02M) without destroying the nanoparticles. Third, the high enrichment effect could enhance the sensitivity of LFIA. Scheme 1 illustrates the Fe3O4@NH2 enrichment and Pb(II) strip sensor detection process.
The first step was to optimize the solid-to-liquid ratio that was used to adsorb Pb(II) with coexisting ions. As shown in , when the solid-to-liquid ratio increased from 0.02 to 0.25 g/L, Pb(II) showed an increase from 67.6% to 93.3%. Although Al(III) showed nearly 100% adsorption, the Al(III) concentration was around 20 ng/mL in lake water, which could not interfere with adsorption of Pb(II).
Figure 5. Optimize the solid-to-liquid ratio for Pb(II) adsorption by Fe3O4@NH2 with coexisting ions. Reaction conditions: Fe3O4@NH2 concentration: 0.25 g/L to 0.02 g/L; solution pH: 7; solution volume: 5 mL; ion strength: 50 mg/L NaCl; equilibrium time: 30 min; temperature: room temperature.
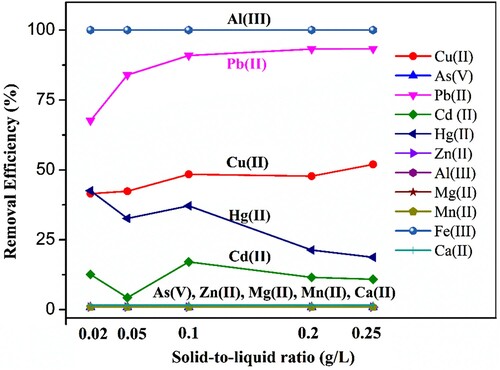
In this study, to make sure that the Pb(II) in lake water was completely adsorbed, 1.25 mg Fe3O4@NH2 (0.25 g/L) was used to concentrate the 5 mL sample solution in the following experiments. Large volume water samples make magnetic separation difficult and may cause adsorbent mass loss. A low solid-to-liquid ratio (0.02 g/L) adsorbent was not recommended because of its associated problems of long experimental time and possible mass loss in the magnetic separation process. As a control, 0.05 g/L (0.25 mg/5 mL) Fe3O4 and 0.05 g/L (0.25 mg/5 mL) Fe3O4-GO were also evaluated. At this solid-to-liquid ratio, Zn(II), Hg(II), Cd(II), and Mn(II) were adsorbed with low removal efficiency due to the competitive effect from other metal ions, as shown in Figures S4 and S5.
As described in the previous section, Pb(II), Cd(II), Hg(II), Cu(II), and As(V) showed suppressed adsorption onto Fe3O4@NH2 at pH 2, which implied that acid treatment was a feasible approach to regeneration and recovery. First, 1.25 mg Fe3O4@NH2 was used to concentrate a 5 mL 100 ng/mL lake water sample for 30 min. Desorption experiments were then done after magnetic separation. In the desorption experiments, 1 mL 0.02 M HCl could elute Pb(II) completely within 2 min. The effect is shown in and proved favourable for the onsite detection method described below. As for Fe3O4 and Fe3O4-GO, their elution efficiencies were around 20% at the same condition. Chelating agents (i.e. ITCBE) showed low Pb(II) elution efficiency (<10%).
Table 2. Pb(II) elution from Fe3O4@NH2 with different time.
Use of Fe3O4@NH2 for adsorption and desorption of Pb(II) in lake water by LFIA was also investigated. For fast onsite detection, 0.2 mg Fe3O4@NH2 were added into 5 mL lake water spiked with 20 ng/mL Pb(II) for 10 min adsorption, and ∼87% of the Pb(II) was adsorbed. 1 mL 0.02 M HCl was used to elute for 2 min. 180 µL elution was mixed with 20 µL 0.1 M Tris pH 10 containing 1 mM ITCBE, which could form complexes with Pb(II) and adjust the solution pH. 0.05 mg Fe3O4 and 0.05 mg Fe3O4-GO were also used in control experiments.
First, the Pb(II) concentration in the supernatant was evaluated by the strip. As shown in , the test lines on strips 2, 4, and 6 had almost the same colour intensities as those on strips 1, 3, and 5, indicating successful adsorption of Pb(II) by Fe3O4, Fe3O4-GO, and Fe3O4@NH2. The elution was then tested according to the previously described process; the test lines on strips 8 and 10 were slightly weaker than those on strips 7 and 9, indicating incomplete desorption of Pb(II) on Fe3O4 and Fe3O4-GO by 0.02 M HCl. Compared with strip 11, strip 12 showed only the control line, which meant a higher desorption efficiency of Pb(II) on Fe3O4@NH2. The strip results showed that the Pb(II) adsorption and desorption performance of the three type of nanomaterials were consistent with instrument results.
Figure 6. Sensitive detection of Pb(II) in supernatant (A and C) and elution (B and D) by immunostrip. The Pb(II) strip responses to the supernatant of negative lake water and lake water spiked with 20 ng/mL by Fe3O4 (strip 1 and strip 2), by Fe3O4-GO (strip 3 and strip 4), by Fe3O4@NH2 (strip 5 and strip 6). The Pb(II) strip responses to 0.02 M HCl elution from Fe3O4 (strip 7 and strip 8), from Fe3O4-GO (strip 9 and strip 10), from Fe3O4@NH2 (strip 11 and strip 12).
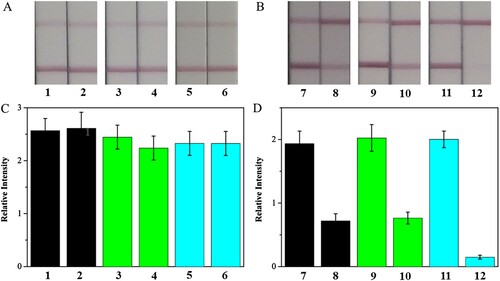
Furthermore, a series of experiments involving Pb(II) (0, 2, 5, 10, 20, and 50 ng/mL) spiked in lake water were performed to determine the cut-off value of this Fe3O4@NH2-combined LFIA method. In , the cut-off value was 20 ng/mL, indicating that the sensitivity had increased five times and that the detection result for 10 ng/mL Pb(II) was obviously observable by the naked eye or a strip reader machine. The sensitivity of the Fe3O4@NH2 enriched Pb(II) strip sensor was due primarily to the sensitivity of the antibody and the selective enrichment process, whereas selectivity was due to antibody specificity.
Figure 7. Sensitive detection of Pb(II) enriched from lake water by Fe3O4@NH2. The Pb(II) strip (inset) responses to negative lake water without Pb(II) added (strip 1), to different concentration of Pb(II) spiked (strip 2: 2 ng/mL; strips 3: 5 ng/mL; strip 4: 10 ng/mL; strip 5: 20 ng/mL; strip 6: 50 ng/mL).
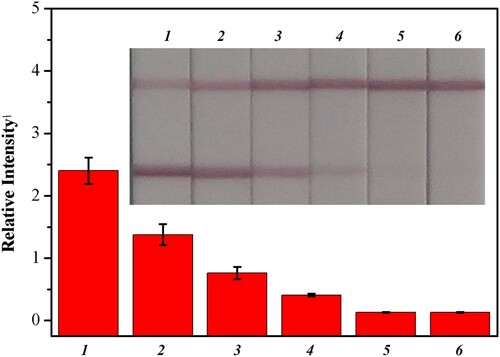
Conclusions
This is the first report to investigate the adsorption behaviour of different types of adsorbents in solutions of multiple metal ions, including Pb(II), Hg(II), Cu(II), Zn(II), As(V), and Cd(II) and apply these nanomaterials for developing a low cost, environmentally friendly, and portable heavy-metal LFIA test.Fe3O4–GO showed a general adsorption ability for metal ions. Fe3O4@NH2 decreased interference from other cations, including Cd(II), Hg(II), Zn(II), Mg(II), Mn(II), Fe(III), and Ca(II). A simple and robust Fe3O4@NH2 enrichment process combined with LFIA could specifically detect trace Pb(II) in lake water. New methods could be simply designed by allowing heavy metals to be adsorbed and detected on the surfaces of magnetic materials to achieve high enhancement. In the future, the Fe3O4 composite as a convenient tool can perhaps be used to extract heavy metals combined in food matrices, which will be a major research challenge.
Disclosure statement
No potential conflict of interest was reported by the authors.
Additional information
Funding
References
- Amuda, O. S., Giwa, A. A., & Bello, I. A. (2007). Removal of heavy metal from industrial wastewater using modified activated coconut shell carbon. Biochemical Engineering Journal, 36(2), 174–181. doi: https://doi.org/10.1016/j.bej.2007.02.013
- Anbia, M., & Lashgari, M. (2009). Synthesis of amino-modified ordered mesoporous silica as a new nano sorbent for the removal of chlorophenols from aqueous media. Chemical Engineering Journal, 150(2), 555–560. doi: https://doi.org/10.1016/j.cej.2009.02.023
- Bargar, J. R., Brown, G. E., & Parks, G. A. (1997). Surface complexation of Pb(II) at oxide-water interfaces: II. XAFS and bond-valence determination of mononuclear Pb(II) sorption products and surface functional groups on iron oxides. Geochimica et Cosmochimica Acta, 61(13), 2639–2652. doi: https://doi.org/10.1016/S0016-7037(97)00125-7
- Chandra, V., Park, J., Chun, Y., Lee, J. W., Hwang, I.-C., & Kim, K. S. (2010). Water-dispersible magnetite-reduced graphene oxide composites for arsenic removal. ACS Nano, 4(7), 3979–3986. doi: https://doi.org/10.1021/nn1008897
- Cheng, S., Yang, Y., Ni, X., Peng, J., & Lai, W. (2017). Fluorescent microspheres lateral flow assay for sensitive detection of the milk allergen casein. Food and Agricultural Immunology, 28(6), 1017–1028.
- Cong, H. P., He, J. J., Lu, Y., & Yu, S. H. (2010). Water-soluble magnetic-functionalized reduced graphene oxide sheets: In situ synthesis and magnetic resonance imaging applications. Small, 6(2), 169–173. doi: https://doi.org/10.1002/smll.200901360
- Cui, L., Wang, Y., Gao, L., Hu, L., Yan, L., Wei, Q., & Du, B. (2015). EDTA functionalized magnetic graphene oxide for removal of Pb(II), Hg(II) and Cu(II) in water treatment: Adsorption mechanism and separation property. Chemical Engineering Journal, 281, 1–10. doi: https://doi.org/10.1016/j.cej.2015.06.043
- Deng, J., Yang, M., Wu, J., Zhang, W., & Jiang, X. (2018). A self-contained chemiluminescent lateral flow assay for point-of-care testing. Analytical Chemistry, 90, 9132–9137. doi: https://doi.org/10.1021/acs.analchem.8b01543
- Dixit, S., & Hering, J. G. (2003). Comparison of arsenic(V) and arsenic(III) sorption onto iron oxide minerals: Implications for arsenic mobility. Environmental Science & Technology, 37(18), 4182–4189. doi: https://doi.org/10.1021/es030309t
- Dong, Y. L., Zhang, H. G., Rahman, Z. U., Su, L., Chen, X. J., Hu, J., & Chen, X. G. (2012). Graphene oxide-Fe3O4 magnetic nanocomposites with peroxidase-like activity for colorimetric detection of glucose. Nanoscale, 4(13), 3969–3976. doi: https://doi.org/10.1039/c2nr12109c
- Fried, T., Shemer, G., & Markovich, G. (2001). Ordered Two-dimensional arrays of ferrite nanoparticles. Advanced Materials, 13(15), 1158–1161. doi: https://doi.org/10.1002/1521-4095(200108)13:15<1158::AID-ADMA1158>3.0.CO;2-6
- Geng, Z., Lin, Y., Yu, X., Shen, Q., Ma, L., Li, Z., … Wang, X. (2012). Highly efficient dye adsorption and removal: A functional hybrid of reduced graphene oxide–Fe3O4 nanoparticles as an easily regenerative adsorbent. Journal of Materials Chemistry, 22(8), 3527. doi: https://doi.org/10.1039/c2jm15544c
- Gu, D., & Fein, J. B. (2015). Adsorption of metals onto graphene oxide: Surface complexation modeling and linear free energy relationships. Colloids and Surfaces A: Physicochemical and Engineering Aspects, 481, 319–327. doi: https://doi.org/10.1016/j.colsurfa.2015.05.026
- Han, Q., Wang, Z., Xia, J., Chen, S., Zhang, X., & Ding, M. (2012). Facile and tunable fabrication of Fe3O4/graphene oxide nanocomposites and their application in the magnetic solid-phase extraction of polycyclic aromatic hydrocarbons from environmental water samples. Talanta, 101, 388–395. doi: https://doi.org/10.1016/j.talanta.2012.09.046
- Kanel, S. R., Manning, B., Charlet, L., & Choi, H. (2005). Removal of arsenic(III) from groundwater by nanoscale zero-valent iron. Environmental Science & Technology, 39(5), 1291–1298. doi: https://doi.org/10.1021/es048991u
- Kassaee, M. Z., Motamedi, E., & Majdi, M. (2011). Magnetic Fe3O4-graphene oxide/polystyrene: Fabrication and characterization of a promising nanocomposite. Chemical Engineering Journal, 172(1), 540–549. doi: https://doi.org/10.1016/j.cej.2011.05.093
- Kuang, H., Xing, C., Hao, C., Liu, L., Wang, L., & Xu, C. (2013). Rapid and highly sensitive detection of lead ions in drinking water based on a strip immunosensor. Sensors, 13(4), 4214–4224. doi: https://doi.org/10.3390/s130404214
- Li, X., Wang, S., Liu, Y., Jiang, L., Song, B., Li, M., … Ding, Y. (2017). Adsorption of Cu(II), Pb(II), and Cd(II) ions from acidic aqueous solutions by diethylenetriaminepentaacetic acid-modified magnetic graphene oxide. Journal of Chemical & Engineering Data, 62(1), 407–416. doi: https://doi.org/10.1021/acs.jced.6b00746
- Li, J., Zhang, S., Chen, C., Zhao, G., Yang, X., Li, J., & Wang, X. (2012). Removal of Cu(II) and fulvic acid by graphene oxide nanosheets decorated with Fe3O4 nanoparticles. ACS Applied Materials & Interfaces, 4(9), 4991–5000. doi: https://doi.org/10.1021/am301358b
- Li, P., Zhang, X-Q., Chen, Y-J., Bai, T-Y., Lian, H-Z., & Hu, X. (2014). One-pot synthesis of thiol- and amine-bifunctionalized mesoporous silica and applications in uptake and speciation of arsenic. RSC Advances., 4(90), 49421–49428. doi: https://doi.org/10.1039/C4RA06563H
- Liu, J., Sun, Z., Deng, Y., Zou, Y., Li, C., Guo, X., … Zhao, D. (2009). Highly water-dispersible biocompatible magnetite particles with low cytotoxicity stabilized by citrate groups. Angewandte Chemie, 48(32), 5875–5879. doi: https://doi.org/10.1002/anie.200901566
- Liu, J.-f., Zhao, Z.-s., & Jiang , G.-b. (2008). Coating Fe3O4 magnetic nanoparticles with humic acid for high efficient removal of heavy metals in water. Environmental Science & Technology, 42(18), 6949–6954. doi: https://doi.org/10.1021/es800924c
- Madadrang, C. J., Kim, H. Y., Gao, G., Wang, N., Zhu, J., Feng, H., … Hou, S. (2012). Adsorption behavior of EDTA-graphene oxide for Pb (II) removal. ACS Applied Materials & Interfaces, 4(3), 1186–1193. doi: https://doi.org/10.1021/am201645g
- Manning, B. A., Hunt, M. L., Amrhein, C., & Yarmoff, J. A. (2002). Arsenic(III) and arsenic(V) reactions with zerovalent iron corrosion products. Environmental Science & Technology, 36(24), 5455–5461. doi: https://doi.org/10.1021/es0206846
- Najafi, M., Yousefi, Y., & Rafati, A. A. (2012). Synthesis, characterization and adsorption studies of several heavy metal ions on amino-functionalized silica nano hollow sphere and silica gel. Separation and Purification Technology, 85, 193–205. doi: https://doi.org/10.1016/j.seppur.2011.10.011
- Nassar, N. N. (2010). Rapid removal and recovery of Pb(II) from wastewater by magnetic nanoadsorbents. Journal of Hazardous Materials, 184(1), 538–546. doi: https://doi.org/10.1016/j.jhazmat.2010.08.069
- Ngah, W. S. W., & Fatinathan, S. (2010). Adsorption characterization of Pb(II) and Cu(II) ions onto chitosan-tripolyphosphate beads: Kinetic, equilibrium and thermodynamic studies. Journal of Environmental Management, 91(4), 958–969. doi: https://doi.org/10.1016/j.jenvman.2009.12.003
- Pan, S., Zhang, X., Qian, J., Lu, Z., Hua, M., Cheng, C., & Pan, B. (2017). A new strategy to address the challenges of nanoparticles in practical water treatment: Mesoporous nanocomposite beads via flash freezing. Nanoscale, 9(48), 19154–19161. doi: https://doi.org/10.1039/C7NR06980D
- Peng, X., Kang, L., Pang, F., Li, H., Luo, R., Luo, X., & Sun, F. (2018). A signal-enhanced lateral flow strip biosensor for ultrasensitive and on-site detection of bisphenol A. Food and Agricultural Immunology, 29(1), 216–227. doi: https://doi.org/10.1080/09540105.2017.1365822
- Peng, W., Li, H., Liu, Y., & Song, S. (2017). A review on heavy metal ions adsorption from water by graphene oxide and its composites. Journal of Molecular Liquids, 230, 496–504. doi: https://doi.org/10.1016/j.molliq.2017.01.064
- Perreault, F., Fonseca de Faria, A., & Elimelech, M. (2015). Environmental applications of graphene-based nanomaterials. Chemical Society Reviews, 44(16), 5861–5896. doi: https://doi.org/10.1039/C5CS00021A
- Ren, X., Wu, Q., Xu, H., Shao, D., Tan, X., Shi, W., … Wang, X. (2016). New insight into GO, cadmium(II), phosphate interaction and Its role in GO colloidal behavior. Environmental Science and Technology, 50(17), 9361–9369. doi: https://doi.org/10.1021/acs.est.6b02934
- Shahbazi, A., Younesi, H., & Badiei, A. (2011). Functionalized SBA-15 mesoporous silica by melamine-based dendrimer amines for adsorptive characteristics of Pb(II), Cu(II) and Cd(II) heavy metal ions in batch and fixed bed column. Chemical Engineering Journal, 168(2), 505–518. doi: https://doi.org/10.1016/j.cej.2010.11.053
- Shen, J., Hu, Y., Shi, M., Li, N., Ma, H., & Ye, M. (2010). One step synthesis of graphene oxide-magnetic nanoparticle composite. The Journal of Physical Chemistry C, 114(3), 1498–1503. doi: https://doi.org/10.1021/jp909756r
- Stankovich, S., Dikin, D. A., Dommett, G. H. B., Kohlhaas, K. M., Zimney, E. J., Stach, E. A., … Ruoff, R. S. (2006). Graphene-based composite materials. Nature, 442, 282–286. doi: https://doi.org/10.1038/nature04969
- Su, J., Cao, M., Ren, L., & Hu, C. (2011). Fe3O4–graphene nanocomposites with improved lithium storage and magnetism properties. The Journal of Physical Chemistry C, 115(30), 14469–14477. doi: https://doi.org/10.1021/jp201666s
- Sun, J., Liang, Q., Han, Q., Zhang, X., & Ding, M. (2015). One-step synthesis of magnetic graphene oxide nanocomposite and its application in magnetic solid phase extraction of heavy metal ions from biological samples. Talanta, 132(Suppl. C), 557–563. doi: https://doi.org/10.1016/j.talanta.2014.09.043
- Vilela, D., Parmar, J., Zeng, Y., Zhao, Y., & Sanchez, S. (2016). Graphene-Based microbots for toxic heavy metal removal and recovery from water. Nano Letters, 16(4), 2860–2866. doi: https://doi.org/10.1021/acs.nanolett.6b00768
- Wang, H., Yuan, X., Wu, Y., Chen, X., Leng, L., Wang, H., … Zeng, G. (2015). Facile synthesis of polypyrrole decorated reduced graphene oxide–Fe3O4 magnetic composites and its application for the Cr(VI) removal. Chemical Engineering Journal, 262(Suppl. C), 597–606. doi: https://doi.org/10.1016/j.cej.2014.10.020
- Wang, H., Yuan, X., Wu, Y., Huang, H., Zeng, G., Liu, Y., … Qi, Y. (2013). Adsorption characteristics and behaviors of graphene oxide for Zn(II) removal from aqueous solution. Applied Surface Science, 279(Suppl. C), 432–440. doi: https://doi.org/10.1016/j.apsusc.2013.04.133
- Wang, D., Zhang, G., Zhou, L., Wang, M., Cai, D., & Wu, Z. (2017a). Synthesis of a multifunctional graphene oxide-based magnetic nanocomposite for efficient removal of Cr(VI). Langmuir : the ACS Journal of Surfaces and Colloids, 33(28), 7007–7014. doi: https://doi.org/10.1021/acs.langmuir.7b01293
- Wang, Z., Zheng, Q., Guo, L., Suryoprabowo, S., Liu, L., & Kuang, H. (2017b). Preparation of an anti-dexamethasone monoclonal antibody and its use in development of a colloidal gold immunoassay. Food and Agricultural Immunology, 28(6), 958–968.
- Wang, J., Zheng, S., Shao, Y., Liu, J., Xu, Z., & Zhu, D. (2010). Amino-functionalized Fe(3)O(4)@SiO(2) core-shell magnetic nanomaterial as a novel adsorbent for aqueous heavy metals removal. Journal of Colloid and Interface Science, 349(1), 293–299. doi: https://doi.org/10.1016/j.jcis.2010.05.010
- Xin, X., Wei, Q., Yang, J., Yan, L., Feng, R., Chen, G., … Li, H. (2012). Highly efficient removal of heavy metal ions by amine-functionalized mesoporous Fe3O4 nanoparticles. Chemical Engineering Journal, 184(Suppl. C), 132–140. doi: https://doi.org/10.1016/j.cej.2012.01.016
- Xing, C., Jing, X., Zhang, X., & Yuan, J. (2017). Ultrasensitive indirect competitive ELISA and strip sensor for detection of furazolidone metabolite in animal tissues. Food and Agricultural Immunology, 28(6), 1269–1282.
- Xing, C., Liu, L., Song, S., Feng, M., Kuang, H., & Xu, C. (2015). Ultrasensitive immunochromatographic assay for the simultaneous detection of five chemicals in drinking water. Biosensors and Bioelectronics, 66(0), 445–453. doi: https://doi.org/10.1016/j.bios.2014.12.004
- Xing, C., Liu, L., Zhang, X., Kuang, H., & Xu, C. (2014). Colorimetric detection of mercury based on a strip sensor. Analytical Methods, 6(16), 6247–6253. doi: https://doi.org/10.1039/C3AY42002G
- Xiong, C., Wang, W., Tan, F., Luo, F., Chen, J., & Qiao, X. (2015). Investigation on the efficiency and mechanism of Cd(II) and Pb(II) removal from aqueous solutions using MgO nanoparticles. Journal of Hazardous Materials, 299, 664–674. doi: https://doi.org/10.1016/j.jhazmat.2015.08.008
- Xu, L., Xing, C., Liu, L., Song, S., Kuang, H., & Xu, C. (2016). Quick, easy, cheap, effective, rugged and safe strategy for quantifying cadmium polluted rice. Food and Agricultural Immunology, 27, 1–13. doi: https://doi.org/10.1080/09540105.2015.1055554
- Yang, X., Zhang, X., Ma, Y., Huang, Y., Wang, Y., & Chen, Y. (2009). Superparamagnetic graphene oxide-Fe3O4 nanoparticles hybrid for controlled targeted drug carriers. Journal of Materials Chemistry, 19(18), 2710–2714. doi: https://doi.org/10.1039/b821416f
- Zhang, H., Dang, Q., Liu, C., Cha, D., Yu, Z., Zhu, W., & Fan, B. (2017a). Uptake of Pb(II) and Cd(II) on chitosan microsphere surface successively grafted by methyl acrylate and diethylenetriamine. ACS Applied Materials & Interfaces, 9(12), 11144–11155. doi: https://doi.org/10.1021/acsami.7b00480
- Zhang, X., Wu, M., Dong, H., Li, H., & Pan, B. (2017b). Simultaneous oxidation and sequestration of As(III) from water by using redox polymer-based Fe(III) oxide nanocomposite. Environmental Science & Technology, 51(11), 6326–6334. doi: https://doi.org/10.1021/acs.est.7b00724
- Zhang, J., Zhai, S., Li, S., Xiao, Z., Song, Y., An, Q., & Tian, G. (2013a). Pb(II) removal of Fe3O4@SiO2–NH2 core–shell nanomaterials prepared via a controllable sol–gel process. Chemical Engineering Journal, 215-216, 461–471. doi: https://doi.org/10.1016/j.cej.2012.11.043
- Zhang, S., Zhang, Y., Liu, J., Xu, Q., Xiao, H., Wang, X., … Zhou, J. (2013b). Thiol modified Fe3O4@SiO2 as a robust, high effective, and recycling magnetic sorbent for mercury removal. Chemical Engineering Journal, 226, 30–38. doi: https://doi.org/10.1016/j.cej.2013.04.060
- Zhao, D., Gao, X., Wu, C., Xie, R., Feng, S., & Chen, C. (2016). Facile preparation of amino functionalized graphene oxide decorated with Fe3O4 nanoparticles for the adsorption of Cr(VI). Applied Surface Science, 384(Suppl. C), 1–9. doi: https://doi.org/10.1016/j.apsusc.2016.05.022
- Zhao, G., Ren, X., Gao, X., Tan, X., Li, J., Chen, C., … Wang, X. (2011). Removal of Pb(ii) ions from aqueous solutions on few-layered graphene oxide nanosheets. Dalton Transactions, 40(41), 10945–10952. doi: https://doi.org/10.1039/c1dt11005e
- Zhou, J., Song, H., Ma, L., & Chen, X. (2011). Magnetite/graphene nanosheet composites: Interfacial interaction and its impact on the durable high-rate performance in lithium-ion batteries. RSC Advances, 1(5), 782. doi: https://doi.org/10.1039/c1ra00402f
- Zhu, H., Tan, X., Tan, L., Zhang, H., Liu, H., Fang, M. … Wang,, X. (2018). Magnetic porous polymers prepared via high internal phase emulsions for efficient removal of Pb2+ and Cd2+. ACS Sustainable Chemistry & Engineering, 6, 5206–5213. doi: https://doi.org/10.1021/acssuschemeng.7b04868
- Zou, Y., Wang, X., Khan, A., Wang, P., Liu, Y., Alsaedi, A., … Wang, X. (2016). Environmental remediation and application of nanoscale zero-valent iron and its composites for the removal of heavy metal ions: A review. Environmental Science & Technology, 50(14), 7290–7304. doi: https://doi.org/10.1021/acs.est.6b01897
- Zubir, N. A., Yacou, C., Motuzas, J., Zhang, X., & Diniz da Costa, J. C. (2015). Structural and functional investigation of graphene oxide-Fe3O4 nanocomposites for the heterogeneous Fenton-like reaction. Scientific Reports, 4, 3201. doi: https://doi.org/10.1038/srep04594