ABSTRACT
Vascular endothelial growth factor (VEGF) is well recognized as an essential component of angiogenesis and the increased proliferation and migration of endothelial cells. Bone marrow-derived endothelial progenitor cells (EPCs) are involved in VEGF-induced vessel formation during physiological and pathological states. Soya-cerebroside, an extract from Cordyceps militaris, reduces synovial inflammation and prevents cartilage damage in an osteoarthritis model. However, the role of soya-cerebroside in VEGF-regulated EPC angiogenesis is uncertain. Records from the Oncomine database demonstrate higher levels of VEGF in cancerous tissue compared with normal tissue. This study describes VEGF-induced promotion of EPC-associated angiogenesis in vivo and how the treatment of EPCs with soya-cerebroside inhibited VEGF-facilitated migration and tube formation. The study evidence shows that the c-Src, FAK and Runx2 signalling pathways are involved in the inhibitory effects of soya-cerebroside. This novel agent may therefore be used to inhibit EPC-associated angiogenesis.
1. Introduction
The development of new blood capillaries through angiogenesis (Folkman, Citation2006) is crucial to key physiological features such as tissue remodelling, bone formation and reproduction (MacDonald et al., Citation2018; Viallard & Larrivee, Citation2017). Angiogenesis is also associated with pathological conditions including tumor development and metastasis, retinopathy and inflammatory diseases (Carmeliet, Citation2005). Inhibiting angiogenesis is therefore an important strategy in cancer treatment and other angiogenesis-associated diseases (Carmeliet & Jain, Citation2000; Jain, Citation2014; Lii et al., Citation2016). Vascular endothelial growth factor (VEGF) is well recognized for its ability to increase endothelial cell proliferation and migration, and facilitate angiogenesis. Thus, VEGF and VEGF receptor (VEGFR) small molecule inhibitors or anti-angiogenic antibodies have been approved for clinic use in the treatment of cancer and angiogenesis-related disorders (Bikfalvi, Citation2004; Shibuya, Citation2015).
Endothelial progenitor cells (EPCs) are also involved in the physiological and pathological progression of new vessel formation (Kiewisz, Kaczmarek, Pawlowska, Kmiec, & Stompor, Citation2016; Patel, Donovan, & Khosrotehrani, Citation2016). EPCs contain cell surface markers CD133, CD34 and vascular endothelial growth factor receptor-2 (VEGFR2), which facilitate postnatal vasculogenesis (Asahara et al., Citation1999) and exert regenerative effects. Importantly, tumour-secreted VEGF promotes EPC mobilization and thus facilitates the development and angiogenesis of several types of tumours (Peters et al., Citation2005). EPCs serve as a critical component of the angiogenic switch that controls tumour proliferation and metastasis (Jain & Carmeliet, Citation2012).
The development of anti-angiogenic agents from natural compounds is demonstrating promise as a new strategy for preventing tumour progression and treating metastasis (Lee, Chen, et al., Citation2019; Lee, Wang, et al., Citation2019). For example, the entomopathogenic fungus Cordyceps militaris is used in traditional Chinese medicine to treat inflammatory disorders (Brent et al., Citation2016). We have previously shown how soya-cerebroside, an extract from Cordyceps militaris, effectively inhibits inducible nitric oxide synthase and cyclooxygenase-2 production in macrophages (Chiu et al., Citation2016). Soya-cerebroside also inhibits monocyte chemoattractant protein-1 production in vitro and in vivo and thus reduces synovial inflammation and prevents cartilage damage (Liu et al., Citation2017, Citation2019). Glucocerebroside also an extract from Cordyceps militaris, reduces angiogenesis (Lee, Wang, et al., Citation2019). Up until now, the role of soya-cerebroside in VEGF-induced EPC angiogenesis has been unclear. Records from the Oncomine database demonstrate higher levels of VEGF in cancerous tissue compared with normal tissue. Our data have shown that soya-cerebroside inhibits EPC migration and tube formation activities and it is known that the inhibitory effects of soya-cerebroside are achieved through the c-Src, FAK and Runx2 signalling pathways. This study examined whether soya-cerebroside shows potential in the treatment of EPC-associated angiogenesis.
2. Materials and methods
2.1. Materials
We obtained antibodies against p-c-Src, p-FAK, p-PI3 K, p-Akt, p-Raf, p-MEK, p-c-Jun, c-Src, FAK, PI3 K, Akt, Raf, MEK, c-Jun, Runx2 and β-actin from Santa Cruz Biotechnology (CA, USA). Human VEGF was purchased from PeproTech (Rocky Hill, NJ, USA).
2.2. Analysis of the Oncomine dataset
Gene expression profile records were obtained from the Oncomine database for normal tissue samples and tumour tissue samples for analysis of VEGF expression.
2.3. Cell culture
Human primary EPCs were prepared according to our previous reports (Wu et al., Citation2014; Wang et al., Citation2015). Cells were maintained in a humidified incubator at 37°C, 5% CO2.
2.4. Western blot analysis
Extracted proteins were resolved by SDS-PAGE and transferred to Immobilon® PVDF membranes. Western blot analysis was performed according to our previous reports (Su et al., Citation2018; Wu, Lin, Tsai, Huang, & Tang, Citation2018; Yang et al., Citation2019).
2.5. EPC migration and tube formation assays
EPCs were incubated with soya-cerebroside (1–10 μM) for 24 h. EPC migration and tube formation was assayed using the methods described in our previous work (Liu et al., Citation2014).
2.6. Chick embryo chorioallantoic membrane (CAM) assay
The CAM assay used fertilized chicken eggs and followed the protocol as detailed in our previous research (Lien & Tang, Citation2019).
2.7. Matrigel plug angiogenesis assay
Nude male mice were subcutaneously injected with VEGF-containing Matrigel (BD Biosciences; Bedford, MA, USA). The matrigel plug angiogenesis assay and immunohistochemical (IHC) staining were performed as described in our previous reports (Chen et al., Citation2019; Liu et al., Citation2018; Wang et al., Citation2019).
2.8. Statistics
All values are presented as the mean ± standard error of the mean (SEM). Differences between the two experimental groups were assessed for significance using the Student’s t-test. The difference was considered to be significant if the p value was < 0.05.
3. Results
3.1. Correction of VEGF expression in human cancer tissue
Levels of VEGF expression in cancerous tissue increase as proliferative and invasive activities of cancer cells increase (Liu et al., Citation2014; Pang et al., Citation2004). Our analysis of Oncomine database records revealed higher levels of VEGF mRNA expression amongst breast, lung, colon, prostate and liver cancer specimens than in healthy tissue samples (), suggesting that VEGF expression correlates with human cancer progression and development.
3.2. VEGF facilitates EPC-induced angiogenesis in vivo
It is well established that VEGF facilitates aberrant tumour angiogenesis in vitro (Stevens & Oltean, Citation2019). We examined whether VEGF promotes EPC angiogenesis in vivo. The CAM and Matrigel plug assays showed that VEGF stimulation increased vessel formation in vivo ((A,B)), while IHC staining indicated that VEGF enhanced levels of the vessel marker CD31 and EPC-specific markers CD34 and CD133 ((B)). Our results demonstrate that VEGF increases EPC-induced angiogenesis in vivo.
Figure 2. VEGF promotes EPC angiogenesis in vivo. (A) Five-day-old fertilized chick embryos were treated with VEGF. After 3 days, the CAMs were examined by microscopy and photographed. (B) Matrigel plugs were treated with VEGF and subcutaneously injected into the flanks of nude male mice. After 7 days, the plugs were photographed and immunostained with CD31, CD34, and CD133 antibodies. Data represent the mean ± S.E.M. *, p < 0.05 compared with the control group.
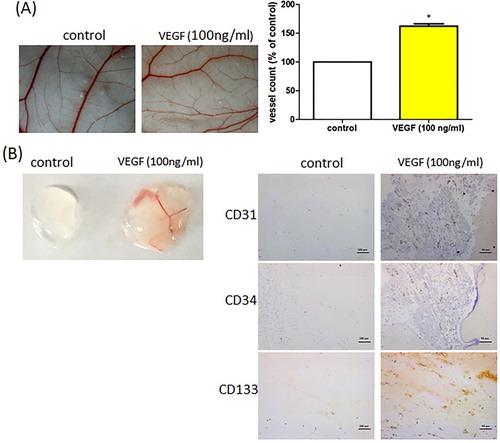
3.3. Soya-cerebroside inhibits VEGF-induced EPC angiogenesis
Next, we investigated whether soya-cerebroside inhibits VEGF-enhanced EPC angiogenesis. It has been reported that cerebroside derivatives have apoptotic effects in human cancer cells (Yazama et al., Citation2015), so we used the MTT assay to analyze the apoptotic effects of soya-cerebroside in EPCs. Stimulation of soya-cerebroside (1–10 μM) for 24 or 48 h did not affect EPC viability (), so this concentration range was used for further experiments. Incubation of EPCs with soya-cerebroside concentration-dependently diminished VEGF-induced cell migration () and formation of capillary-like structures (). These results indicated that soya-cerebroside suppresses VEGF-induced EPC angiogenesis.
Figure 3. Soya-cerebroside does not induce cell death in EPCs. EPCs were treated with varying concentrations of soya-cerebroside (1–10 μM) for 24 or 48 h and cell viability was determined using the MTT assay. Data represent the mean ± S.E.M.
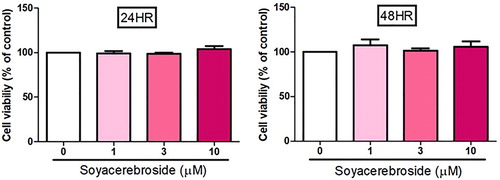
Figure 4. Soya-cerebroside inhibits VEGF-facilitated EPC migration. EPCs were incubated with VEGF and soya-cerebroside (1–10 μM) for 24 h. Cell migration was examined by the Transwell assay. Data represent the mean ± S.E.M. *, p < 0.05 compared with the control group; #, p < 0.05 compared with the VEGF-treated group.
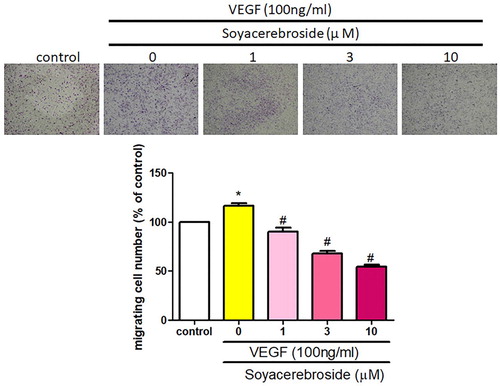
Figure 5. Soya-cerebroside inhibits VEGF-facilitated EPC tube formation. EPCs were incubated with VEGF and soya-cerebroside (1–10 μM) for 24 h. Capillary-like structure formation was examined using the tube formation assay. Data represent the mean ± S.E.M. *, p < 0.05 compared with the control group; #, p < 0.05 compared with the VEGF-treated group.
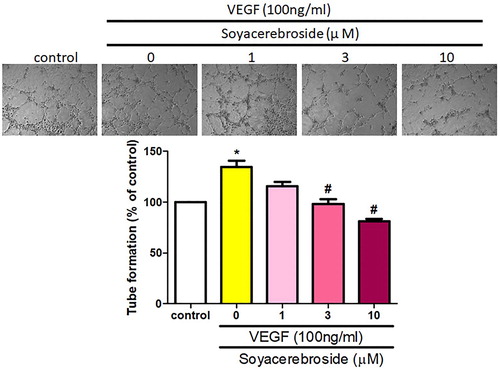
3.4. Soya-cerebroside inhibits VEGF-induced promotion of the c-Src, FAK and Runx2 pathway
The c-Src and FAK signalling pathway plays a critical role in VEGF-regulated angiogenesis (Wang et al., Citation2017). Incubation of EPCs with VEGF increased c-Src phosphorylation, while soya-cerebroside inhibited VEGF-induced c-Src activation ((A)). Similarly, soya-cerebroside diminished VEGF-facilitated FAK phosphorylation ((A)). The PI3K/Akt and Raf/MEK signalling cascades are common downstream signalling events of VEGF stimulation (Chen et al., Citation2019; Huang, Huang, Li, & Zeng, Citation2018). However, we failed to find any evidence showing that soya-cerebroside had significant effects upon PI3K/Akt and Raf/MEK phosphorylation ((B,C)), indicating that the c-Src/FAK pathway, and not the PI3K/Akt and Raf/MEK signalling cascades, regulated the soya-cerebroside-induced suppression of VEGF-enhanced EPC angiogenesis.
Figure 6. Soya-cerebroside inhibits VEGF-facilitated c-Src and FAK phosphorylation. EPCs were incubated with VEGF and soya-cerebroside (1–10 μM) for 24 h. c-Src, FAK, PI3 K, Akt, Raf and MEK phosphorylation was examined by Western blot analysis.
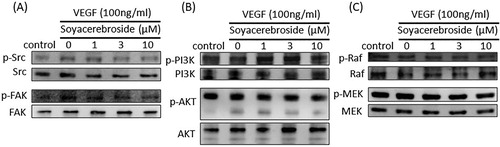
Runx2 is an important transcriptional factor that controls EPC angiogenesis during bone metabolism (Li et al., Citation2019). We therefore investigated whether Runx2 mediates the inhibitory effects of soya-cerebroside. Incubation of EPCs with soya-cerebroside diminished VEGF-augmented Runx expression ((A)). c-Jun activation has also been reported to regulate VEGF-controlled EPC angiogenesis (Tsai et al., Citation2019). Here, we failed to find that soya-cerebroside markedly affected VEGF-promoted c-Jun phosphorylation ((B)), suggesting that Runx2, but not c-Jun, regulates soya-cerebroside-inhibited EPC angiogenesis.
4. Discussion
Increasingly, research has focused on the role of angiogenesis in tumour cell growth, motility and metastasis. Tumour-associated angiogenesis arises through an imbalance between pro- and anti-angiogenic mediators (Carmeliet, Citation2005). VEGF is a chief regulator of EPC angiogenesis, facilitating tumour proliferation and metastasis (Krishna Priya et al., Citation2016). Levels of VEGF expression correlate with the clinical stages of many cancer types (Ribatti, Citation2016). In the current study, our analysis of records from the Oncomine dataset confirmed that VEGF levels are higher in human tissue samples of breast, lung, colon, prostate and liver cancer than in tissue from healthy individuals. Our in vitro and animal data from CAM and Matrigel plug assays demonstrated that VEGF facilitates increases in EPC expression and new vessel formation. We also found that soya-cerebroside has anti-angiogenic properties at non-cytotoxic concentrations. Our evidence demonstrated the involvement of the c-Src, FAK and Runx2 signalling pathways in soya-cerebroside-induced inhibition of EPC angiogenesis, indicating that this compound may have promise as a therapeutic candidate in angiogenesis-associated disorders.
The c-Src/FAK, PI3 K/Akt and Raf/MEK signalling pathways regulate VEGF-mediated angiogenesis (Ribatti, Citation2016; Wu et al., Citation2018). In this study, we found that soya-cerebroside diminished VEGF-induced phosphorylation of c-Src and FAK, indicating that the c-Src/FAK pathway is involved in the inhibitory effects of soya-cerebroside on angiogenesis. Our lack of evidence for any significant effects of soya-cerebroside upon VEGF-induced increases in PI3K, Akt, Raf, or MEK phosphorylation indicates that c-Src/FAK, but not PI3 K/Akt or Raf/MEK, is a key player in soya-cerebroside-induced inhibition of angiogenesis. Similarly, other researchers have reported that the inhibitory effects of glucocerebroside upon angiogenesis occur via the c-Src and FAK pathways (Lee, Wang, et al., Citation2019). The EGF-like ligand, amphiregulin, binds to EGFR and increases VEGF-dependent angiogenesis through c-Src and FAK activation (Wang et al., Citation2017), while D-pinitol inhibits prostate cancer metastasis by suppressing the c-Src and FAK pathways (Lin et al., Citation2013). This combined evidence indicates important roles for the c-Src and FAK pathways in angiogenesis and metastasis.
Runx2, a key transcription factor, controls bone remodelling and mineralization (Komori, Citation2017) and may promote angiogenesis that leads to bone formation (Li et al., Citation2019). In the current study, we found that stimulation of soya-cerebroside diminished VEGF-enhanced Runx2 upregulation. It has been reported that c-Jun plays an important role in EPC angiogenesis (Tsai et al., Citation2019). However, we failed to find any effects of soya-cerebroside upon VEGF-enhanced phosphorylation of c-Jun. Therefore, the transcription factor Runx2, but not AP-1, is implicated in the downregulating effects of soya-cerebroside in EPC angiogenesis.
Cordyceps militaris is traditionally used in Chinese medicine to treat inflammatory disease (Brent et al., Citation2016). In our previous study, we isolated soya-cerebroside from Cordyceps militaris extract and found evidence of anti-inflammatory activity (Chiu et al., Citation2016). As far as we are aware, the current investigation is the first to show that soya-cerebroside inhibits VEGF-induced promotion of EPC angiogenesis. We have detailed mechanisms involving c-Src, FAK and Runx2 in the inhibitory effects of soya-cerebroside in angiogenesis (). We suggest that soya-cerebroside may be a novel agent for the treatment of angiogenesis-regulated disorders.
Acknowledgments
This work was supported by grants from the Ministry of Science and Technology of Taiwan (MOST-108-2314-B-039-034-MY3), China Medical University (CMU108-ASIA-09), China Medical University Hospital (DMR-109-008) and by funding from China Medical University under the Higher Education Sprout Project, Ministry of Education, Taiwan (CMRC-CHM-3-1).
Disclosure statement
No potential conflict of interest was reported by the authors.
Additional information
Funding
References
- Asahara, T., Masuda, H., Takahashi, T., Kalka, C., Pastore, C., Silver, M., … Isner, J. M. (1999). Bone marrow origin of endothelial progenitor cells responsible for postnatal vasculogenesis in physiological and pathological neovascularization. Circulation Research, 85(3), 221–228. doi: 10.1161/01.RES.85.3.221
- Bikfalvi, A. (2004). Recent developments in the inhibition of angiogenesis: Examples from studies on platelet factor-4 and the VEGF/VEGFR system. Biochemical Pharmacology, 68(6), 1017–1021. doi: 10.1016/j.bcp.2004.05.030
- Brent, C. S., Miyasaki, K., Vuong, C., Miranda, B., Steele, B., Brent, K. G., & Nath, R. (2016). Regulatory roles of biogenic amines and juvenile hormone in the reproductive behavior of the western tarnished plant bug (Lygus hesperus). Journal of Comparative Physiology B, 186(2), 169–179. doi: 10.1007/s00360-015-0953-1
- Carmeliet, P. (2005). Angiogenesis in life, disease and medicine. Nature, 438(7070), 932–936. doi: 10.1038/nature04478
- Carmeliet, P., & Jain, R. K. (2000). Angiogenesis in cancer and other diseases. Nature, 407(6801), 249–257. doi: 10.1038/35025220
- Chen, S. S., Tang, C. H., Chie, M. J., Tsai, C. H., Fong, Y. C., Lu, Y. C., … Wang, S. W. (2019). Resistin facilitates VEGF-A-dependent angiogenesis by inhibiting miR-16-5p in human chondrosarcoma cells. Cell Death & Disease, 10(1), 31. doi: 10.1038/s41419-018-1241-2
- Chiu, C. P., Liu, S. C., Tang, C. H., Chan, Y., El-Shazly, M., Lee, C. L., … Wu, Y. C. (2016). Anti-inflammatory cerebrosides from cultivated Cordyceps militaris. Journal of Agricultural and Food Chemistry, 64(7), 1540–1548. doi: 10.1021/acs.jafc.5b05931
- Folkman, J. (2006). Angiogenesis. Annual Review of Medicine, 57, 1–18. doi: 10.1146/annurev.med.57.121304.131306
- Huang, M., Huang, B., Li, G., & Zeng, S. (2018). Apatinib affect VEGF-mediated cell proliferation, migration, invasion via blocking VEGFR2/RAF/MEK/ERK and PI3K/AKT pathways in cholangiocarcinoma cell. BMC Gastroenterology, 18(1), 169. doi: 10.1186/s12876-018-0870-3
- Jain, R. K. (2014). Antiangiogenesis strategies revisited: From starving tumors to alleviating hypoxia. Cancer Cell, 26(5), 605–622. doi: 10.1016/j.ccell.2014.10.006
- Jain, R. K., & Carmeliet, P. (2012). Snapshot: Tumor angiogenesis. Cell, 149(6), 1408–1408.e1. doi: 10.1016/j.cell.2012.05.025
- Kiewisz, J., Kaczmarek, M. M., Pawlowska, A., Kmiec, Z., & Stompor, T. (2016). Endothelial progenitor cells participation in cardiovascular and kidney diseases: A systematic review. Acta Biochimica Polonica, 63(3), 475–482. doi: 10.18388/abp.2016_1284
- Komori, T. (2017). Roles of Runx2 in skeletal development. Advances in Experimental Medicine and Biology, 962, 83–93. doi: 10.1007/978-981-10-3233-2_6
- Krishna Priya, S., Nagare, R. P., Sneha, V. S., Sidhanth, C., Bindhya, S., Manasa, P., & Ganesan, T. S. (2016). Tumour angiogenesis-origin of blood vessels. International Journal of Cancer, 139(4), 729–735. doi: 10.1002/ijc.30067
- Lee, H. P., Chen, P. C., Wang, S. W., Fong, Y. C., Tsai, C. H., Tsai, F. J., … Tang, C. H. (2019). Plumbagin suppresses endothelial progenitor cell-related angiogenesis in vitro and in vivo. Journal of Functional Foods, 52, 537–544. doi: 10.1016/j.jff.2018.11.040
- Lee, H. P., Wang, S. W., Wu, Y. C., Tsai, C. H., Tsai, F. J., Chung, J. G., … Tang, C. H. (2019). Glucocerebroside reduces endothelial progenitor cell-induced angiogenesis. Food and Agricultural Immunology, 30(1), 1033–1045. doi: 10.1080/09540105.2019.1660623
- Li, N., Wang, W. B., Bao, H., Shi, Q., Jiang, Z. L., Qi, Y. X., & Han, Y. (2019). MicroRNA-129-1-3p regulates cyclic stretch-induced endothelial progenitor cell differentiation by targeting Runx2. Journal of Cellular Biochemistry, 120(4), 5256–5267. doi: 10.1002/jcb.27800
- Lien, M. Y., & Tang, C. H. (2019). The MCP-1/CCR2 axis enhances VEGF-A-dependent angiogenesis by directly downregulating miR-29c expression in oral squamous cell carcinoma. Cancer Research, 79(13), doi: 10.1158/1538-7445.AM2019-200
- Lii, C. K., Chang, J. W., Chen, J. J., Chen, H. W., Liu, K. L., Yeh, S. L., … Li, C. C. (2016). Docosahexaenoic acid inhibits 12-O-tetradecanoylphorbol-13- acetate-induced fascin-1-dependent breast cancer cell migration by suppressing the PKCdelta- and Wnt-1/beta-catenin-mediated pathways. Oncotarget, 7(18), 25162–25179. doi: 10.18632/oncotarget.7301
- Lin, T. H., Tan, T. W., Tsai, T. H., Chen, C. C., Hsieh, T. F., Lee, S. S., … Tang, C. H. (2013). D-pinitol inhibits prostate cancer metastasis through inhibition of alphaVbeta3 integrin by modulating FAK, c-Src and NF-kappaB pathways. International Journal of Molecular Sciences, 14(5), 9790–9802. doi: 10.3390/ijms14059790
- Liu, S. C., Chiu, C. P., Tsai, C. H., Hung, C. Y., Li, T. M., Wu, Y. C., & Tang, C. H. (2017). Soya-cerebroside, an extract of Cordyceps militaris, suppresses monocyte migration and prevents cartilage degradation in inflammatory animal models. Scientific Reports, 7, 43205. doi: 10.1038/Srep43205
- Liu, S. C., Chuang, S. M., Hsu, C. J., Tsai, C. H., Wang, S. W., & Tang, C. H. (2014). CTGF increases vascular endothelial growth factor-dependent angiogenesis in human synovial fibroblasts by increasing miR-210 expression. Cell Death & Disease, 5, e1485–e1485. doi: 10.1038/cddis.2014.453
- Liu, J. F., Lee, C. W., Tsai, M. H., Tang, C. H., Chen, P. C., Lin, L. W., … Chao, C. C. (2018). Thrombospondin 2 promotes tumor metastasis by inducing matrix metalloproteinase-13 production in lung cancer cells. Biochemical Pharmacology, 155, 537–546. doi: 10.1016/j.bcp.2018.07.024
- Liu, S. C., Tsai, C. H., Wu, T. Y., Tsai, C. H., Tsai, F. J., Chung, J. G., … Tang, C. H. (2019). Soya-cerebroside reduces IL-1β-induced MMP-1 production in chondrocytes and inhibits cartilage degradation: Implications for the treatment of osteoarthritis. Food and Agricultural Immunology, 30(1), 620–632. doi: 10.1080/09540105.2019.1611745
- MacDonald, I. J., Liu, S. C., Su, C. M., Wang, Y. H., Tsai, C. H., & Tang, C. H. (2018). Implications of angiogenesis involvement in arthritis. International Journal of Molecular Sciences, 19(7), 2012. doi: 10.3390/ijms19072012
- Pang, S. T., Flores-Morales, A., Skoog, L., Chuan, Y. C., Nordstedt, G., & Pousette, A. (2004). Regulation of matrix metalloproteinase 13 expression by androgen in prostate cancer. Oncology Reports, 11(6), 1187–1192.
- Patel, J., Donovan, P., & Khosrotehrani, K. (2016). Concise review: Functional definition of endothelial progenitor cells: A molecular perspective. STEM CELLS Translational Medicine, 5(10), 1302–1306. doi: 10.5966/sctm.2016-0066
- Peters, B. A., Diaz, L. A., Polyak, K., Meszler, L., Romans, K., Guinan, E. C., … Lengauer, C. (2005). Contribution of bone marrow-derived endothelial cells to human tumor vasculature. Nature Medicine, 11(3), 261–262. doi: 10.1038/nm1200
- Ribatti, D. (2016). Tumor refractoriness to anti-VEGF therapy. Oncotarget, 7(29), 46668–46677. doi: 10.18632/oncotarget.8694
- Shibuya, M. (2015). VEGF-VEGFR system as a target for suppressing inflammation and other diseases. Endocrine, Metabolic & Immune Disorders-Drug Targets, 15(2), 135–144. doi: 10.2174/1871530315666150316121956
- Stevens, M., & Oltean, S. (2019). Modulation of receptor tyrosine kinase activity through alternative splicing of ligands and receptors in the VEGF-A/VEGFR axis. Cells, 8(4), 288. doi: 10.3390/cells8040288
- Su, C. M., Tang, C. H., Chi, M. J., Lin, C. Y., Fong, Y. C., Liu, Y. C., … Wang, S. W. (2018). Resistin facilitates VEGF-C-associated lymphangiogenesis by inhibiting miR-186 in human chondrosarcoma cells. Biochemical Pharmacology, 154, 234–242. doi: 10.1016/j.bcp.2018.05.001
- Tsai, H. C., Cheng, S. P., Han, C. K., Huang, Y. L., Wang, S. W., Lee, J. J., … Tang, C. H. (2019). Resistin enhances angiogenesis in osteosarcoma via the MAPK signaling pathway. Aging, 11(21), 9767–9777. doi: 10.18632/aging.102423
- Viallard, C., & Larrivee, B. (2017). Tumor angiogenesis and vascular normalization: Alternative therapeutic targets. Angiogenesis, 20(4), 409–426. doi: 10.1007/s10456-017-9562-9
- Wang, M., Chao, C. C., Chen, P. C., Liu, P. I., Yang, Y. C., Su, C. M., … Tang, C. H. (2019). Thrombospondin enhances RANKL-dependent osteoclastogenesis and facilitates lung cancer bone metastasis. Biochemical Pharmacology, 166, 23–32. doi: 10.1016/j.bcp.2019.05.005
- Wang, C. Q., Huang, Y. W., Wang, S. W., Huang, Y. L., Tsai, C. H., Zhao, Y. M., … Tang, C. H. (2017). Amphiregulin enhances VEGF-A production in human chondrosarcoma cells and promotes angiogenesis by inhibiting miR-206 via FAK/c-Src/PKCdelta pathway. Cancer Letters, 385, 261–270. doi: 10.1016/j.canlet.2016.10.010
- Wang, S. W., Liu, S. C., Sun, H. L., Huang, T. Y., Chan, C. H., Yang, C. Y., … Tang, C. H. (2015). CCL5/CCR5 axis induces vascular endothelial growth factor-mediated tumor angiogenesis in human osteosarcoma microenvironment. Carcinogenesis, 36(1), 104–114. doi: 10.1093/carcin/bgu218
- Wu, M. H., Huang, C. Y., Lin, J. A., Wang, S. W., Peng, C. Y., Cheng, H. C., & Tang, C. H. (2014). Endothelin-1 promotes vascular endothelial growth factor-dependent angiogenesis in human chondrosarcoma cells. Oncogene, 33(13), 1725–1735. doi: 10.1038/onc.2013.109
- Wu, T. J., Lin, C. Y., Tsai, C. H., Huang, Y. L., & Tang, C. H. (2018). Glucose suppresses IL-1β-induced MMP-1 expression through the FAK, MEK, ERK, and AP-1 signaling pathways. Environmental Toxicology, 33(10), 1061–1068. doi: 10.1002/tox.22618
- Yang, Y. C., Chiou, P. C., Chen, P. C., Liu, P. Y., Huang, W. C., Chao, C. C., & Tang, C. H. (2019). Melatonin reduces lung cancer stemness through inhibiting of PLC, ERK, p38, β-catenin, and Twist pathways. Environmental Toxicology, 34(2), 203–209. doi: 10.1002/tox.22674
- Yazama, H., Kitatani, K., Fujiwara, K., Kato, M., Hashimoto-Nishimura, M., Kawamoto, K., … Okazaki, T. (2015). Dietary glucosylceramides suppress tumor growth in a mouse xenograft model of head and neck squamous cell carcinoma by the inhibition of angiogenesis through an increase in ceramide. International Journal of Clinical Oncology, 20(3), 438–446. doi: 10.1007/s10147-014-0734-y