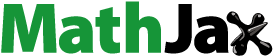
ABSTRACT
Ochratoxin A (OTA) is a highly toxic potential contaminant of most agricultural products, and OTA is difficult to remove in general food production processes. In this study, we immobilized polyvinyl pyrrolidone-coated carboxypeptidase A (CPA) into zeolitic imidazolate framework material to improve the ability for OTA degradation. In this method, carboxypeptidase A was dispersed well in the material and maintained its stability and ability to degrade OTA, under complex matrix conditions with better repeated use performance than free carboxypeptidase A. The substrate conversion rate of CPA increased 3 times after immobilization. After repeated use of 10 cycles, the composite enzyme still retained 71.37% of its initial activity. The degradation rate of OTA was 30.69% higher than that of free CPA. This method may play an important role in the degradation of mycotoxins.
1. Introduction
Ochratoxin is a class of toxic secondary metabolites mainly produced by moulds such as Aspergillus ochratoxin, A. sulphureus, and Penicillium verrucosum. Ochratoxin A (OTA) is one of the most toxic and widely distributed ochratoxin species. OTA is difficult to remove in general food production and processing due to its high chemical and thermal stability. The detection of OTA mainly depends on HPLC-based analysis (Cubero-Leon et al., Citation2017; Mohammad, Citation2018; Yoshimitsu et al., Citation2019) or immunological detection system based on highly sensitive antigen-antibody interactions (Hao et al., Citation2018; Kong et al., Citation2016). Although highly sensitive detection methods avoid accidental OTA contamination (Liu et al., Citation2018), these detection methods cannot avoid the resulting significant economic loss. Therefore, it is of considerable practical significance to find an efficient and stable degradation method for reducing OTA contamination. Detoxification and degradation of OTA is commonly achieved using physical, chemical, and biological methods. However, the processing cost is high, the operation is complicated, and the most critical aspect is the safety consideration of the method (Calado et al., Citation2018; Kamber et al., Citation2017). The safety of chemical reagents and reaction by-products still limits the application of chemical and physical methods in food processing. As a useful green and sustainable methodology, enzymatic catalysis has good selectivity, thermal stability, and environmentally friendly properties; this has attracted much attention in the fields of food processing (Zhou et al., Citation2019), biosensing, biomedical devices, and degradation of environmental contaminants (Nadar & Rathod, Citation2019).
Carboxypeptidase A (CPA) is located in the mammalian pancreas and has a relative molecular weight of 34.6 kDa. As a zinc-dependent carboxypeptidase, CPA can specifically catalyse the degradation of OTA into OTα and l-β-phenylalanine, thus greatly reducing its toxicity. The degradation product OTα has about 1000 times lower toxicity and one-tenth half-life period than OTA (Abrunhosa & Venâncio, Citation2007; Deberghes et al., Citation1995; Pitout, Citation1969; Xiong et al., Citation2017). There are reports on the degradation of OTA with CPA (Deberghes et al., Citation1995); however, the application of this enzyme has been limited due to the lack of stability, recyclability, and recuperability (Majewski et al., Citation2017). These problems can be solved by enzyme immobilization, which is an effective technique for improving catalytic stabilization, separation, and reutilization over multiple cycles (Jiandong et al., Citation2018).
The best supporting material for protein immobilization should have a hierarchical pore structure containing both large pores for immobilization of proteins and smaller free pores used to transfer reactants and products during biocatalysis (Lian et al., Citation2017). Recently, crystalline metal–organic frameworks (MOFs) have been utilized as promising candidates to encapsulate and protect enzymes from harsh conditions (Wu et al., Citation2019). MOFs are composed of both inorganic and organic components, which can support different kinds of interactions with the enzyme, such as hydrogen bonding, van der Waals forces, and covalent and/or coordinative bonding (Majewski et al., Citation2017). Moreover, due to the richness of the geometry and connectivity of metal nodes and ligands, the structure and topology of MOFs can be tailored for specific applications (Lian et al., Citation2017). The most common means of enzyme encapsulation by MOFs are the adsorption method and biomineralization method (Wu et al., Citation2019). Zeolitic imidazolate framework (ZIF) materials comprise an important class of MOFs with tuneable pore size and have the classical chemical functionality of MOFs, exceptional chemical stability, and structural zeolitic diversity (Cao et al., Citation2017). Previously, ZIF-8 was reported as a promising carrier for enzyme immobilization, because enzymes encapsulated in ZIF-8 usually have greatly increased stability (Zhang et al., Citation2017).
In this study, we immobilized polyvinylpyrrolidone coated CPA in ZIF-8 (CPA/PVP/ZIF-8) by the biomineralization method. The use of immobilized CPA can not only improve the degradation ability but also enhance the stability of CPA to achieve effective degradation of OTA. Hence, the synthetic operations and enzymatic performance are discussed in detail below.
2. Materials and methods
2.1. Reagents and materials
2-Methylimidazole and zinc acetate were purchased from Shanghai Maclean Biochemical Technology Co., Ltd. (Shanghai, China). CPA was purchased from Sigma-Aldrich (Shanghai, China). Z-Phe-Leu, l-leucine, ninhydrin, cadmium chloride, and coomassie brilliant blue G250 were purchased from Aladdin Industrial Co., Ltd. (Shanghai, China). OTA was purchased from J&K Scientific, Ltd. (Shanghai, China). The OTA ELISA detection kit was purchased from Hua’an Maike Biotechnology Co., Ltd. (Beijing, China). All chemicals were purchased from commercial sources and used without purification.
2.2. Synthesis of CPA/PVP/ZIF-8
2.2.1. Preparation of ZIF-8
2-methylimidazole and zinc acetate were dissolved in deionized water (2.4 M, 8 mL; 0.32 M, 2 mL), respectively. These two solutions were mixed under stirring continuously for 24 h at room temperature. The mixture was centrifuged at 10,000 r/min for 5 min and then subjected to three centrifugation-wash cycles using deionized water.
2.2.2. Biomimetic mineralization of CPA/PVP/ZIF-8
The CPA/PVP/ZIF-8 were synthesized by coprecipitation method following reported procedure with some modifications (Lyu et al., Citation2014). Free CPA was added to 475 µL of PVP solution (5–45 mg/mL) for 15 min ultrasonic treatment and then centrifuged to obtain the CPA/PVP complex. The CPA/PVP (8.75–33.75 mg) was mixed with 2-Methylimidazole and zinc acetate solution in a 20-mL flask by stirring for 24 h at room temperature. The precipitate was obtained by centrifugation at 10,000 r/min for 5 min and then subjected to three centrifugation-wash cycles using deionized water.
Transmission electron microscopy (TEM), Fourier transform infrared (FTIR) spectroscopy, powder X-ray diffraction (PXRD) and nitrogen adsorption–desorption isotherms (BET) were used to confirm the structure of the materials.
The enzyme activity of free CPA, CPA/PVP, and CPA/PVP/ZIF-8 complex were analyzed using modified Cd-Ninhydrin method with Z-Phe-Leu as substrate (Doi et al., Citation1981).
2.3. Activity measurement of CPA/PVP/ZIF-8
The enzyme activity of free CPA and CPA/PVP/ZIF-8 complex were analyzed at different temperatures, pH values, organic reagents, and recycling times.
The substrate solution was prepared in PBS buffer. The thermal stability was investigated at 25°C, 35°C, 45°C, 55°C, and 65°C. The pH of the substrate solution was adjusted to 5, 6, 7.5, 9.4, or 10.5 to evaluate pH stability. The CPA/PVP/ZIF-8 complex and free CPA was incubated with substrate which was dissolved in organic solvent (15%, 20%, 30%, 40%, or 50% ethanol in PBS buffer) at 35°C for 12 h to investigate the stability in organic solvent. The reusability was evaluated by incubating at 35°C for 12 h in ultrafiltration tube (free CPA) or common tube (CPA/PVP/ZIF-8). The residual reaction solution of free CPA or complex was removed by ultrafiltration or centrifugation, respectively. The sediment was washed separately using potassium phosphate buffer and then added to the substrate solution for repeated incubation to determine the product content.
The conversion (%) was calculated according to EquationEq. (1(1)
(1) ):
(1)
(1) where qe (µmol/L) is the concentration of the produced l-leucine; qm (µmol/L) is the concentration of substrate solution; m is the dilution factor; and V (L) is the volume of the solution.
2.4. Study on the degradation of OTA in practical sample
The degradation product of OTA by CPA/PVP/ZIF-8 complex was analyzed by LC-MS method, and the degradation process was monitored by UPLC method. The result was provided by the Academy of National Food and Strategic Reserves Administration, Beijing, China.
For sample analysis, OTA was added into watermelon juice at 250 ng/mL (pH 5.7). The experimental setup included five groups: control (without CPA and ZIF-8), free CPA, CPA/PVP/ZIF-8 composite, mixture of free CPA and ZIF-8, and pure ZIF-8. For each group, the analysis was taken under the same protein or ZIF-8 concentration. The degradation result was analyzed by ELISA detection kit and UPLC.
3. Results and discussion
3.1. Structural characterization of CPA/PVP/ZIF-8
3.1.1. Optimum condition for CPA/PVP/ZIF-8 preparation
PVP is a kind of nonionic polymer compound with the hydrophilic group, which can render the particles charged and prevent reassociation. The activity of CPA/PVP would be decreased when the PVP concentration was too high or low. It is possible that an excess of PVP reduced the affinity between the particles and the substrate, and there is no significant influence with low PVP concentration. The best PVP concentration for CPA/PVP complex was analyzed under optimal degradation conditions (pH 7.5, 35°C, 15 µg CPA, 4 mM substrate solution, Figure S1). The result was shown in Figure S2(A) that the best PVP concentration for CPA/PVP complex was 15 mg/mL because the highest catalytic activity of CPA/PVP was taken with the 15 mg/mL PVP solution.
The loading amount and immobilization yield of CPA were also optimized to obtain the CPA/PVP/ZIF-8 complex with the optimum load effect. Different amount of CPA/PVP were used for coprecipitation, and the mixture gradually turned from transparent to milky white. The result was shown in Figure S2(B), and the best immobilization yield was 91.24% with 23.75 mg of CPA/PVP loaded with 0.465 mg of ZIF-8, the loading amount is 46.60 mg/mg (CPA/PVP:ZIF-8)
3.1.2. Structural characterization of CPA/PVP/ZIF-8
The synthesis of ZIF-8 was formed through a process from triangular to quadrilateral and finally a rhombic dodecahedron crystal. And the CPA/PVP can be immobilized into ZIF-8 during this process. The size and morphology of ZIF-8 were investigated by TEM: at 2 min with diameters approximately 160–200 nm ((A)); at 10 min with diameters range of 210–260 nm ((B)); at 24 h with diameters between 240 and 360 nm as a rhombic dodecahedron crystal ((C)).
Figure 1. TEM images for ZIF-8 preparation: (A) Reaction for 2 min; (B) Reaction for 10 min; (C) Reaction for 24 h.
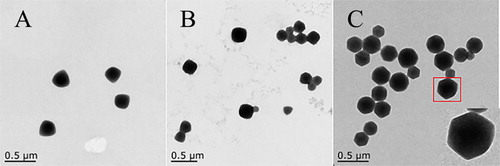
To establish the location of CPA/PVP immobilized into ZIF-8, a calcination process at 170°C for 7 h was carried out to remove protein molecules from the composite. The result of TEM images were shown in . In (A) (ZIF-8) and (B) (CPA/PVP/ZIF-8), both of these two materials have the similar size about 340 nm. However, the surface of CPA/PVP/ZIF-8 is not as smooth as pure ZIF-8 because of the immobilization of CPA/PVP. The cavities with sizes ranging from 25 to 35 nm were existence shown in the (D), which is resulted from the removal of CPA/PVP immobilized into ZIF-8. In contrast, such cavities do not appear in the TEM image of ZIF-8 after the same calcination treatment ((C)). It is clear that the CPA/PVP composite is partially embedded or completely encapsulated in ZIF-8.
Figure 2. TEM images for materials calcination: (A) ZIF-8; (B) CPA/PVP/ZIF-8; (C) ZIF-8 composites after calcination; (D) CPA/PVP/ZIF-8 composites after calcination.
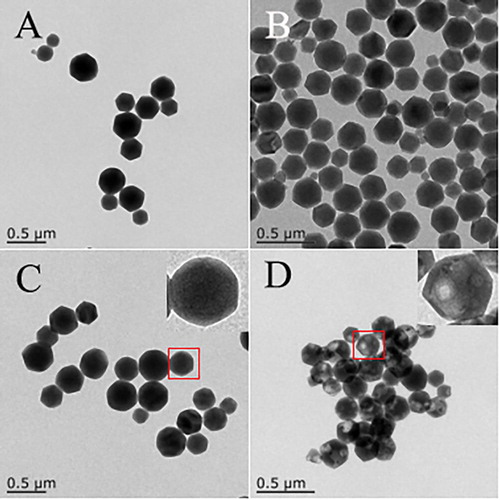
The FTIR spectra of pure CPA, PVP, ZIF-8, and the CPA/PVP/ZIF-8 composite are shown in (A). For ZIF-8, the band at 2927.09 cm−1 is ascribed to the aliphatic stretching of 2-methylimidazole (Patil & Yadav, Citation2018). Two peaks at 1308.23 cm−1 and 1458.55 cm−1 were attributed to C–H and C = C bond stretching (Cui et al., Citation2018). The vibrations at 759 cm−1 and 1421.69 cm−1 are corresponding to the Hmim ring (Adnan, Li, & Wang et al., Citation2018; Liang et al., Citation2015). The specific peaks from 836.3 to 1308.23 cm−1 was attributed to the in-plane bending of 2-methylimidazole (Liang et al., Citation2015). With the pure CPA, peaks at 1659.24 and 1541.12 cm−1 were corresponding to amide I (mainly from C = O stretching mode) and II band (mainly from a combination between of NH bending and CN stretching modes), respectively. However, spectra of CPA/PVP/ZIF-8 composites show the peak at 1666.75 and 1583.03cm−1, which was slightly shifted towards higher wavenumbers in the case of immobilized form possibly due to amide vibrations (Patil & Yadav, Citation2018). Furthermore, the vibrational band at about 1655.12 cm−1 corresponds to C = O stretching of PVP. There are interactions between protein and ZIF-8 while coordinating carbonyl group of proteins with Zn2+ cations of ZIF-8, indicating that the CPA/PVP/ZIF-8 composites were prepared successfully.
Figure 3. (A) Fourier transform infrared (FT-IR) spectra of samples; (B) PXRD patterns of ZIF-8 and CPA/PVP/ZIF-8 composites; (C) N2 adsorption-desorption isotherm for ZIF-8 and CPA/PVP/ZIF-8 composites; (D) BJH pore size distribution for ZIF-8 and CPA/PVP/ZIF-8 composites.
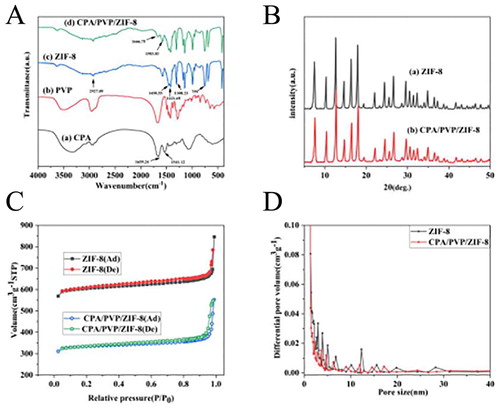
The crystalline structures of ZIF-8 and the CPA/PVP/ZIF-8 composite were identified by PXRD measurements ((B)). The characteristic peaks at 7.48°,10.30°, 12.64°, 14.62°, 16.38°, 17.96°, 24.44°, and 26.60° are assigned to the (011), (002), (112), (022), (113), (222), (233), and (134) planes of crystalline ZIF-8, with a sodalite topology, in good agreement with previous reports (Schejn et al., Citation2014; Wang et al., Citation2019). In contrast, the peaks of the CPA/PVP/ZIF-8 complex shifted slightly to the right by 0.1°, and the intensity of the composite is lower than that of pure ZIF-8, resulting from decreased crystalline interplanar spacing (Adnan, Li, & Wang et al., Citation2018; Adnan, Li, & Xu et al., Citation2018). This result shows that there is a small decrease in the crystallinity of CPA/PVP/ZIF-8 composites after immobilization, and the encapsulation of the biomacromolecules (CPA/PVP) results in modification of the morphological properties of ZIF-8.
The surface area and pore properties were evaluated by nitrogen adsorption–desorption isotherm. The test results for ZIF-8 and CPA/PVP/ZIF-8 composite were shown in (C), which were between Type I and IV (Liang et al., Citation2015). It indicated that the ZIF-8 and CPA/PVP/ ZIF-8 composite contained micropores and mesopores. As shown in (D), the synthesized ZIF-8 material had irregular disordered pores (Abdelghany et al., Citation2015). The BET surface area and pore volume of ZIF-8 were calculated as 566.34 m2/g and 0.56 cm3/g, respectively. However these values decreased to 361.13 m2/g and 0.45 cm3/g after immobilization. The enzyme molecules occupied the original pores and destroyed other pores after immobilization, leading to the pore size to be smaller. These results were resulted from the role of the enzyme in modulating the morphological properties of ZIF-8 during the immobilization process.
3.2. Activity measurement of CPA/PVP/ZIF-8
3.2.1. Activity comparison in optimum condition
The catalytic activity of free CPA and CPA/PVP/ZIF-8 was analyzed at pH 7.5 and 35°C with 2 mL substrates solution (4 mM) and 15 µg CPA (or CPA/PVP/ZIF-8 complex with the same CPA content), and the result was shown in . For free enzyme, the conversion rate was achieved 20.45% at 10 min, and reached to the degradation equilibrium with 26.59% at 30 min. For CPA/PVP/ZIF-8, the conversion rate was lower than free CPA in the first 3 h. However, the conversion rate was 1.2-fold higher than that of the free CPA in the 4th hour. With incubation time increased, the conversion rate of the complex increased to 84.42% at 24 h. For free CPA, the active site is exposed to the substrate solution to achieve a high conversion rate at first, however, prolonged exposure may hurt the site activity. The ZIF-8 can plays a protective role in the enzyme activity for long time catalytic activity of CPA, however, it may hinder the mass transfer at the initial stage. The enzyme activity parameters of free CPA and CPA/PVP/ZIF-8 complexes were calculated according to the Lineweaver-Burk equation (Figure S3). The apparent Km values for CPA/PVP/ZIF-8 composites was higher than that of free CPA, suggesting a lower substrate affinity of immobilized CPA compared to free CPA and the apparent Vmax values of CPA/PVP/ZIF-8 composites was found to be lower than that of native forms (Table S3). It indicated that diffusion limitations were increased after immobilization.
3.2.2. Activity comparison in extreme conditions
Because of the complex conditions of food processing and production, the enzyme activity of free CPA and CPA/PVP/ZIF-8 complex were compared under different conditions. The reaction time was chosen as 12 h because the highest conversion rate of free CPA and CPA/PVP/ZIF-8 complex can be achieved at this time. The substrate concentration was 4 mM, and 15 µg enzyme or equivalent protein content complex was used.
Under the different buffer solution pH values ranged from 5 to 10.5, the analysis results were shown in the (A). The highest conversion rate of both free CPA and CPA/PVP/ZIF-8 were achieve at pH 7.5. However, CPA/PVP/ZIF-8 complex was taken higher conversion rate which is 55.47% higher than that of free CPA. These results indicated that the CPA/PVP/ZIF-8 complex was more stable and can led to broad pH tolerance.
Figure 5. Stability optimization (A) pH stability; (B) temperature-stability; (C) organic reagent content; (D) repeated cycles.
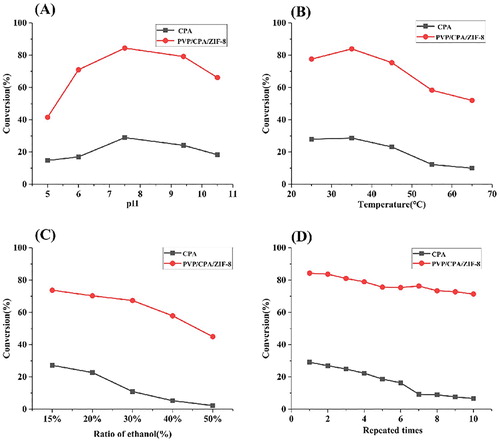
The influence of temperature was studied in the range of 2–65°C. The results were shown in (B). For free CPA and the CPA/PVP/ZIF-8 complex, the varying tendencies of conversion rate were the same with the rise in temperature, and maximizing at 35°C. However, the average conversion rate of CPA/PVP/ZIF-8 complex was 5 times higher than that of free CPA, which indicated that the encapsulation of CPA/PVP in ZIF-8 can protect the enzyme from heat injury and enhance the catalytic capacity. This may be because of the physical and chemical properties of the immobilized enzymes.
The organic solvent stability of the free CPA and immobilized CPA/PVP is shown in (C). The conversion rate of the CPA/PVP/ZIF-8 composite decreased more slowly and resulted in a higher conversion rate than free CPA under the same organic solvent concentration. When the proportion of ethanol reached 50%, the conversion catalysed by free CPA was only 2.27%, whereas the CPA/PVP/ZIF-8 complex converted 44.94%. These results indicated that the ZIF-8 material can effectively protect the structure of CPA from organic solvent, thus preserving enzyme activity.
The reusability of the CPA/PVP/ZIF-8 complex is presented in (D). It was observed that the yield of the CPA/PVP/ZIF-8 complex was retained at 71.37% yield after 10 cycles, whereas the yield of free CPA was only 6.64%. It was mainly because that the free CPA was difficult to recycle. And the mechanical treatment may take physical damage to the enzyme, thus reduce the catalytic activity. Obviously, these results indicate that immobilizing CPA significantly increases its reusability.
3.3. Results of OTA degradation by CPA/PVP/ZIF-8 complex
3.3.1. Degradation ability to OTA
OTA can be degraded into OTα and l-β-phenylalanine by CPA. The degradation product of CPA/PVP/ZIF-8 complex to OTA was analyzed by LC-MS and the result was shown in Figure S4. The obtained degradation product appears at 10.49 min with the molecular weight as 257, which is the desired target substance OTα. Therefore, it can be certain that the OTA can be degraded into OTα by CPA/PVP/ZIF-8 complex.
The degradation process of CPA/PVP/ZIF-8 complex to OTA was monitoring by UPLC. With the extension of degradation time, the OTA content was gradually decrease and the OTα content was gradually increase, which was shown in Figure S5(A). The OTA was degraded into OTα by CPA/PVP/ZIF-8 complex, and taken 19.94% residual after 24 h degradation. Then, free CPA and CPA/PVP/ZIF-8 complex were both used for 24 h OTA degradation, and the result was also analyzed by UPLC. The data was shown in Figure S5(B). The CPA/PVP/ZIF-8 complex was taken higher degradation velocity than free CPA. However, both of free CPA and CPA/PVP/ZIF-8 complex cannot completely degraded the OTA in 24 h.
3.3.2. Actual sample analysis
Five groups sample were analyzed by both UPLC and ELISA kit, the result was shown in and . The OTA did not spontaneously decompose in the control group. After adding ZIF-8 into the sample, the content of OTA decreased slightly during the test, which may be caused by the slight adsorption of the ZIF-8 material to OTA. Free CPA can degraded nearly half of the OTA after 24 h. The mechanical mixing of free CPA and ZIF-8 makes the result of OTA degradation rate a little higher than that of free CPA, which may be caused by simultaneous degradation and adsorption. The CPA/PVP /ZIF-8 composite has the greatest ability to catalyse the degradation of OTA in the sample, and the degradation nearly reached to four fifths at 24 h, which proves that ZIF-8 encapsulation of CPA effectively protected the catalytic activity of free CPA and improved the degradation ability, which has specific practical value. These analysis results were consistent with those of UPLC monitoring result.
Table 1. Detection of OTA degradation rate in different control groups by ELISA kit (n = 3).
Table 2. Detection of OTA degradation rate in different control groups by UPLC.
4. Conclusions
We successfully immobilized PVP coated CPA in ZIF-8 materials by coprecipitation. The substrate conversion rate of CPA increased 3 times after immobilization, and the encapsulated enzyme retained 71.37% activity after repeated use for 10 cycles. In the liquid sample, the results also show that the composite has better degradation capability of OTA than free enzyme. The degradation rate of OTA by the immobilized CPA complex nearly reached four fifths, which was nearly thirty percent more than free CPA. Therefore, the use of organometallic frameworks to protect mycotoxin degradation enzymes may have specific applications for mycotoxin degradation; the application scope and safety of the encapsulated catalysts need to be further explored.
Supplemental Material
Download MS Word (1.5 MB)Acknowledgments
This study was supported by Natural Science Foundation of Jiangsu Province, China (grant number BK20180979), The Natural Science Research of Jiangsu Higher Education Institutions of China (grant number 18KJB550003, 19KJB550006), Doctoral Research start-up Funds of Jiangsu university of science and technology (grant number 1182931801), Emerging science and technology innovation team funding of JUST (grant number 1182921902), Zhenjiang Scientific and Technological Foundation for Social Development (grant number SH2017053), National Natural Science Foundation of China (No. 31901799). Postgraduate Research & Practice Innovation Program of Jiangsu Province (No. SJCX19_0606)
Disclosure statement
No potential conflict of interest was reported by the author(s).
Additional information
Funding
References
- Abdelghany, A. M., Mekhail, M. S., Abdelrazek, E. M., & Aboud, M. M. (2015). Combined DFT/FTIR structural studies of monodispersed PVP/gold and silver nano particles. Journal of Alloys and Compounds, 646, 326–332. https://doi.org/10.1016/j.jallcom.2015.05.262
- Abrunhosa, L., & Venâncio, A. (2007). Isolation and purification of an enzyme hydrolyzing ochratoxin A from Aspergillus niger. Biotechnology Letters, 29(12), 1909–1914. https://doi.org/10.1007/s10529-007-9479-2
- Adnan, M., Li, K., Wang, J., Xu, L., & Yan, Y. (2018). Hierarchical ZIF-8 toward immobilizing Burkholderia cepacia lipase for application in biodiesel preparation. International Journal of Molecular Sciences, 19(5), 1424. https://doi.org/10.3390/ijms19051424
- Adnan, M., Li, K., Xu, L., & Yan, Y. (2018). X-shaped ZIF-8 for immobilization rhizomucor miehei lipase via encapsulation and its application toward biodiesel production. Catalysts, 8(3), 96. https://doi.org/10.3390/catal8030096
- Calado, T., Luisa, F., Verde, S., Venancio, A., & Abrunhosa, L. (2018). Gamma irradiation effects on ochratoxin A: Degradation, cytotoxicity and application in food. Food Chemistry, 240, 463–471. https://doi.org/10.1016/j.foodchem.2017.07.136
- Cao, S.-L., Xu, H., Lai, L.-H., Gu, W.-M., Xu, P., Xiong, J., Yin, H., Li, X.-H., Ma, Y.-Z., Zhou, J., Zong, M.-H., & Lou, W.-Y. (2017). Magnetic ZIF-8/cellulose/Fe3O4 nanocomposite: Preparation, characterization, and enzyme immobilization. Bioresources and Bioprocessing, 4(1), 56. https://doi.org/10.1186/s40643-017-0186-0
- Cubero-Leon, E., Bouten, K., Senyuva, H., & Stroka, J. (2017). Determination of ochratoxin A in black and white pepper, nutmeg, spice mix, cocoa, and drinking chocolate by high-performance liquid chromatography coupled with fluorescence detection: Collaborative study. Journal of Aoac International, 100(5), 1458–1468. https://doi.org/10.5740/jaoacint.16-0430
- Cui, J., Feng, Y., & Jia, S. (2018). Silica encapsulated catalase@ metal-organic framework composite: A highly stable and recyclable biocatalyst. Chemical Engineering Journal, 351, 506–514. https://doi.org/10.1016/j.cej.2018.06.121
- Deberghes, P., Betbeder, A. M., Boisard, F., Blanc, R., Delaby, J. F., Krivobok, S., Steiman, R., Seigle-Murandi, F., & Creppy, E. E. (1995). Detoxification of ochratoxin A, a food contaminant: Prevention of growth of Aspergillus ochraceus and its production of ochratoxin A. Mycotoxin Research, 11(1), 37–47. https://doi.org/10.1007/BF03192060
- Doi, E., Shibata, D., & Matoba, T. (1981). Modified colorimetric ninhydrin methods for peptidase assay. Analytical Biochemistry, 118(1), 173–184. https://doi.org/10.1016/0003-2697(81)90175-5
- Hao, K., Suryoprabowo, S., Song, S. S., Liu, L. Q., & Kuang, H. (2018). Rapid detection of zearalenone and its metabolite in corn flour with the immunochromatographic test strip. Food and Agricultural Immunology, 29(1), 498–510. https://doi.org/10.1080/09540105.2017.1406461
- Jiandong, C., Yuxiao, F., & Shiru, J. (2018). Silica encapsulated catalase@metal-organic framework composite: A highly stable and recyclable biocatalyst. Chemical Engineering Journal, 351, 506–514. https://doi.org/10.1016/j.cej.2018.06.121.
- Kamber, U., Gülbaz, G., Aksu, P., & Doğan, A. (2017). Detoxification of aflatoxin B1 in red pepper (Capsicum annuum L.) by ozone treatment and its effect on microbiological and sensory quality. Journal of Food Processing and Preservation, 41(5), e13102. https://doi.org/10.1111/jfpp.13102
- Kong, D., Liu, L., Song, S., Steven, S., Li, A., Kuang, H., Wang, L., & Xu, C. (2016). A gold nanoparticle-based semi-quantitative and quantitative ultrasensitive paper sensor for the detection of twenty mycotoxins. Nanoscale, 8(9), 5245–5253. https://doi.org/10.1039/C5NR09171C
- Lian, X., Fang, Y., Joseph, E., Wang, Q., Li, J., Banerjee, S., Lollar, C., Wang, X., & Zhou, H.-C. (2017). Enzyme–MOF (metal–organic framework) composites. Chemical Society Reviews, 46(11), 3386–3401. https://doi.org/10.1039/C7CS00058H
- Liang, K., Ricco, R., Doherty, C. M., Styles, M. J., Bell, S., Kirby, N., Mudie, S., Haylock, D., Hill, A. J., Doonan, C. J., & Falcaro, P. (2015). Biomimetic mineralization of metal-organic frameworks as protective coatings for biomacromolecules. Nature Communications, 6, 7240. https://doi.org/10.1038/ncomms8240.
- Liu, L. Q., Xu, L. G., Suryoprabowo, S., Song, S. S., & Kuang, H. (2018). Development of an immunochromatographic test strip for the detection of ochratoxin A in red wine. Food and Agricultural Immunology, 29(1), 434–444. https://doi.org/10.1080/09540105.2017.1401043
- Lyu, F., Zhang, Y., Zare, R. N., Ge, J., & Liu, Z. (2014). One-pot synthesis of protein-embedded metal–organic frameworks with enhanced biological activities. Nano Letters, 14(10), 5761–5765. https://doi.org/10.1021/nl5026419
- Majewski, M. B., Howarth, A. J., Li, P., Wasielewski, M. R., Hupp, J. T., & Farha, O. K. (2017). Enzyme encapsulation in metal-organic frameworks for applications in catalysis. CrystEngComm, 19(29), 4082–4091. https://doi.org/10.1039/C7CE00022G
- Mohammad, A. (2018). Determination of ochratoxin A in fruit juice by high-performance liquid chromatography after vortex-assisted emulsification microextraction based on solidification of floating organic drop. Mycotoxin Research, 34(1), 15–20. https://doi.org/10.1007/s12550-017-0294-x
- Nadar, S. S., & Rathod, V. K. (2019). Immobilization of proline activated lipase within metal organic framework (MOF). International Journal of Biological Macromolecules. https://doi.org/10.1016/j.ijbiomac.2019.10.199.
- Patil, P. D., & Yadav, G. D. (2018). Rapid in situ encapsulation of laccase into metal-organic framework support (ZIF-8) under biocompatible conditions. ChemistrySelect, 3(17), 4669–4675. https://doi.org/10.1002/slct.201702852
- Pitout, M. J. (1969). The hydrolysis of ochratoxin A by some proteolytic enzymes. Biochemical Pharmacology, 18(2), 485–491. https://doi.org/10.1016/0006-2952(69)90224-X
- Schejn, A., Balan, L., Falk, V., Aranda, L., Medjahdi, G., & Schneider, R. (2014). Controlling ZIF-8 nano- and microcrystal formation and reactivity through zinc salt variations. CrystEngComm, 16(21), 4493–4500. https://doi.org/10.1039/C3CE42485E
- Wang, J., Yu, S., Feng, F., & Lu, L. (2019). Simultaneous purification and immobilization of laccase on magnetic zeolitic imidazolate frameworks: Recyclable biocatalysts with enhanced stability for dye decolorization. Biochemical Engineering Journal, 107285. https://doi.org/10.1016/j.bej.2019.107285.
- Wu, X., Yue, H., Zhang, Y., Gao, X., Li, X., Wang, L., Cao, Y., Hou, M., An, H., Zhang, L., & Li, S. (2019). Packaging and delivering enzymes by amorphous metal-organic frameworks. Nature Communications, 10(1), 1–8. https://doi.org/10.1038/s41467-018-07882-8
- Xiong, L., Wang, X., & Liang, Z. (2017). Functions of detoxification and application prospect of carboxypeptidase A. Biotechnology Bulletin, 33(8), 20–25. https://doi.org/10.13560/j.cnki.biotech.bull.1985.2017-0163.
- Yoshimitsu, M., Kiyota, K., Kajimura, K., & Yamano, T. (2019). Development of an LC-MS/MS-based analytical method for quantification of soybean allergen Gly m 4 in soybean grains and processed foods. Food and Agricultural Immunology, 30(1), 25–33. https://doi.org/10.1080/09540105.2018.1540553
- Zhang, C., Wang, X., Hou, M., Li, X., Wu, X., & Ge, J. (2017). Immobilization on metal–organic framework engenders high sensitivity for enzymatic electrochemical detection. ACS Applied Materials & Interfaces, 9(16), 13831–13836. https://doi.org/10.1021/acsami.7b02803
- Zhou, B., Yuan, X., Hu, Y., Fan, J., Yang, W., Guo, M., Zhang, Y., Li, W., & Zhang, J. (2019). Detection of deoxynivalenol (DON) using europium chelates and magnetic nanoparticles. Food and Agricultural Immunology, 30(1), 87–94. doi: 10.1080/09540105.2018.1548577