ABSTRACT
Artemisia rupestris L. polysaccharides have immune enhancement effects with low toxicity in the previous studies. The effect of crude polysaccharides from cultivated Artemisia rupestris L. (CPCAR) on immunity has not been explored. Here, the adjuvant potential of CPCAR was investigated in vivo and its underlying mechanism in vitro was explored through activation of bone marrow-derived dendritic cells (BMDCs). After intramuscular immunizations, CPCAR elevated IgG levels with a TH1-biased response, lymphocyte proliferation and increased CD4 and CD8 T cells in spleen and lymph nodes. IFN-γ and IL-4 of CD8 or CD4 T cells were specifically increased. CPCAR improved DC maturation and diminished Treg frequency. BMDCs treated with CPCAR in vitro showed the up-regulation of CD40, CD86, IL-12 and TNF-α. BMDMs pretreated with TLR4 and NF-kB inhibitors decreased the expressions of CD40 and CD86 and produced less cytokines. These data demonstrated that CPCAR could boost TH1-biased response through DC activation in TLR4/NF-κB signalling.
1. Introduction
Natural components from traditional Chinese medicine (TCM) provide many potential compounds to prevent infectious diseases, such as influenza and emerging virus diseases such as COVID-19 (Ren et al., Citation2021; Riaz et al., Citation2019; Tomar et al., Citation2018). Herbal polysaccharides have important immune-regulatory functions with low toxicity, including antiviral effects. Herbal polysaccharides can regulate the levels of antibodies, lymphocytes, cytokines and mucosal immunity. Many polysaccharides from TCM can be used as vaccine adjuvants, such as AdvaxTM adjuvant for influenza vaccine, West Nile virus vaccine, African horse fever virus vaccine, HIV vaccine and HBV vaccine, Astragalus polysaccharide adjuvant for foot-and-mouth disease vaccine, influenza vaccine and HBV vaccine and ginseng polysaccharide adjuvant for chicken H5N1 vaccine (Garcia-Vello et al., Citation2020; Jiang et al., Citation2010; Sun et al., Citation2018). Therefore, herbal polysaccharides have exhibited great potential as adjuvants in the development of preventive and therapeutic vaccines.
Artemisia rupestris L. has been used as TCM in Xinjiang for a long time. Various pharmacological components in A. rupestris L. have been examined, such as polysaccharides, flavonoids and rupestonic acid (Ma et al., Citation2005). Among them, polysaccharides showed potential immunomodulatory activities, such as anti-virus, antibacterial and anti-inflammatory effects (Bora & Sharma, Citation2011; Park et al., Citation2013). In our previous study, crude polysaccharides from wild A. rupestris L. as an adjuvant-induced a long-term and sustainable immune response eliciting antigen-specific TH1/TH2 immune responses with induction of DCs activation in the influenza vaccine and foot-and-mouth disease vaccine (Wang et al., Citation2019; Zhang, Wang, et al., Citation2017). The preventive and therapeutic effects of wild A. rupestris L. extracts on various immune disorders have been reported in several studies (Jin et al., Citation2014; Sahu et al., Citation2018). Wild A. rupestris L. was a rare plant, cultivated A. rupestris L. has been successfully planted in a large area in Xinjiang and clinically used as TCM. However, the adjuvant potential of crude polysaccharides from cultivated A. rupestris L. (CPCAR) remains obscure.
DCs are activated to increase the levels of surface molecules, produce immunoregulatory cytokines, such as TH1 cytokine IL-12, proinflammatory cytokine TNF-α and subsequently present antigens to trigger T cell response (Banchereau & Steinman, Citation1998). Herbal polysaccharides have been indicated to enhance innate immunity and cytokine production through toll-like receptor (TLR)/NF-kB signalling. TLR4 on DCs is a receptor required for many signalling events in response to herbal polysaccharides. In particular, herbal polysaccharide-stimulated DCs can induce a variety of cytokines through activated NF-κB signalling and regulate adaptive immune responses against infection (Akira & Takeda, Citation2004; Li et al., Citation2015; Zhu et al., Citation2016).
In the study, we investigated the influences of CPCAR on humoral and cellular immune responses, T cell phenotype in secondary lymphoid organs and the function of DCs in animal models. In addition, the possible mechanism of CPCAR in vitro was discussed in order to reveal the immunogenicity induction mechanism of CPCAR.
2. Materials and methods
2.1. Preparation of CPCAR and FT-IR determination
Cultivated A. rupestris L. (A voucher specimen No. AR15063002) was identified and provided by Professor Jiang He from Xinjiang Institute of Materia Medica. The investigated CPCAR was obtained by hot water extraction according to the previous method with some modifications (Zhang, Yang, et al., Citation2017). Briefly, dried powder of cultivated A. rupestris L. was defatted with 95% ethanol and then extracted with distilled water at 80°C. The aqueous extraction was precipitated with ethanol overnight. The precipitate was collected to obtain crude polysaccharides. Extraneous proteins were then removed with the Sevag method (Li et al., Citation2011). The content of polysaccharides was detected to be 32.39% with the phenol-sulfuric acid method. CPCAR with KBr was finely ground and pressed into pellets to test infrared spectrum in the wavenumber range from 4000 cm−1 to 400 cm−1.
2.2. Experimental animals and protocols
Animal experimental procedures were performed according to the guidelines of the Committee on the Ethics of Animal Experiments of Xinjang Key Laboratory of Biological Resources and Genetic Engineering (BRGE-AE001) in Xinjiang University. ICR and C57BL/6 mice (7–9-week-old female mice from Animal Experimental Center of Xinjiang Medical University, Urumqi, China) were used for all animal experiments.
ICR mice were intramuscularly vaccinated at Weeks 0 and 2 and the injection volume of vaccination was 100 μL. Alum (Imject Alum Adjuvant, Thermo) was used as the positive control. Saline administration was used as the negative control. Mice were randomised into six groups, which were respectively vaccinated with 0.9% NaCl, OVA (10 μg per dose), CPCAR-L/OVA (50 μg CPCAR and 10 μg OVA), CPCAR-M/OVA (200 μg CPCAR and 10 μg OVA), CPCAR-H/OVA (400 μg CPCAR and 10 μg OVA) and Alum/OVA (100 μg Alum and 10 μg OVA). Each experimental group contained five mice. Blood samples were harvested via retro-orbital venous plexus after immunisation for the determination of OVA-specific IgG response. According to the same protocol of immunisation, lymphocyte proliferation was performed for the determination of T cell subsets, cytokines, DC surface molecules and Treg.
2.3. Antibody ELISAs
Immune serum was obtained after vaccination for the determination of OVA-specific IgG, IgG1 and IgG2a antibodies. OVA was coated into 96-well microplates overnight. After blocking for 60 min, the diluted serum was incubated for 60 min. HRP-labeled goat anti-mouse antibodies (Southern Biotech) were added at different dilution ratios for 60-min incubation. The colorimetric reaction was developed with tetramethyl-benzidine (TMB) reagent (Sangon Biotech, China) and finally terminated by 2 M H2SO4. Optical density (OD) at 450 nm was tested with a microplate reader (Bio-Rad).
2.4. Lymphocyte proliferation test
To assess splenocyte proliferation, splenocytes (1 × 106 cell/mL, 96-well plate) were collected from immunised ICR mice in seven days after final vaccination and co-incubated with or without OVA (10 μg/mL), LPS (5 μg/mL, Sigma) and ConA (5 μg/mL, Sigma) for 48 h. MTT solution (5 mg/mL, Sigma) was added to each well and incubated for 4 h. The OD490 nm of plates was read. Stimulation index (SI) was calculated as: SI = (OD490 nm of stimulated cells − OD490 nm of medium)/(OD490 nm of non-stimulated cells − OD490 nm of medium).
2.5. Immunophenotypes of T cells
When the mice were vaccinated twice according to two intramuscular doses, tissues were collected. After erythrocyte lysis, cells from spleen and lymph nodes were incubated with Fc-block (BD) for 20 min, followed by washing and staining with antibodies to CD3, CD4, CD8, CD44 and CD62L (BD). Samples were detected on flow cytometers (BD) and the detected data were analysed with FlowJo software.
2.6. Intracellular cytokine staining
The levels of IFN-γ and IL-4 were detected to identify TH1 and TH2 cells in the CD4+ and CD8+ T cells, respectively. The previous intracellular cytokine detection procedure was used (Zhang et al., Citation2011). Briefly, in seven days after the final vaccination, the prepared splenocytes were stimulated with OVA (10 μg/mL) for 4 h and Golgi stop (BD) was added for 12-h treatment. Then, the samples were collected for further surface and intracellular staining before flow cytometric analysis.
2.7. Surface markers on DCs and Treg assay
Cell suspensions from the spleen were prepared after 21 days for determining DCs maturation and Treg frequency. Cells were stained with anti-mouse CD11c, CD40, CD80, CD86 and MHC-II for DCs maturation. Treg assessment was performed with Mouse Regulatory T Cell Staining Kit (eBioscience) according to the manufacturer’s instructions.
2.8. Cell culture and treatment
In vitro experiments, bone marrow-derived dendritic cells (BMDCs) of C57BL/6 mice were generated and incubated for seven days with RPMI-1640 complete medium (Gibco) containing 20 ng/mL GM-CSF (PeproTech) in 24-well plates. For DC activation, BMDCs were harvested and then treated with CPCAR (10–200 μg/mL) or LPS (100 ng/mL, positive control) for 12 h. Three replicates from three mice were arranged for each sample. BMDCs were collected for surface molecular staining. To rule out the possibility of endotoxin contamination, CPCAR solutions or LPS were pre-incubated with PMB (100 μg/mL) for 60 min.
2.9. Determination of co-stimulatory molecules and cytokines in BMDCs
In inhibitor experiments, BMDCs were pretreated with 5 μM TAK-242 (TLR4 inhibitor, MedChemExpress, China) or 30 μM PDTC (NF-kB inhibitor, Beyotime Biotechnology, China) for 1 h and then stimulated with CPCAR (10 and 100 μg/mL) or LPS (100 ng/mL) for 12 h. The expression of co-stimulatory molecules and IL-12 secretion in BMDCs were determined by flow cytometers and the yield of TNF-α in the supernatants from BMDCs was assessed with an ELISA kit according to the protocol provided by the manufacturer.
2.10. Statistical analysis
Experimental data were expressed as mean values ± SD and subjected to statistical analysis through ANOVA and Tukey’s Multiple-Comparison Test with Prism 5 (GraphPad Software). Statistical significance was determined at levels of P < 0.05 and P < 0.01.
3. Results
3.1. FT-IR analysis of CPCAR
The infrared spectra of CPCAR in the range of 4000–400 cm−1 were observed (). IR bands at 3367, 2935 and 1609 cm−1 respectively corresponded to O–H stretching vibration, C–H stretching vibration, and the absorption of C=C or C=O group. IR bands at 800–1200 cm−1 corresponded to the region of carbohydrates and were characteristic absorption bands of polysaccharides.
3.2. Antigen-specific humoral response
To determine the efficacy of CPCAR adjuvant, we tested the serum OVA-specific total IgG, IgG1 and IgG2a response. In seven days after the first vaccination ((A)), the CPCAR-H/OVA group showed a higher IgG level than the groups of OVA alone and Alum/OVA (P < 0.05), indicating that CPCAR elicited quickly IgG response. In 14 and 21 days after the first vaccination ((B)), the group of OVA alone induced a relatively low level of IgG response. In the CPCAR groups, OVA-specific IgG level was increased significantly (P < 0.01), and showed no significant difference compared with the group of Alum/OVA (P > 0.05). In 21 days after the first vaccination ((C,D)), the analysis result of antibody isotype showed a statistically significant enhancement of IgG1 and IgG2a responses (P < 0.01). IgG2a/IgG1 ratio in the group of CPCAR-M/OVA was significantly higher than that in the groups of OVA alone and Alum/OVA (P < 0.01, (E)). In the experiment ((F)), abnormal behaviours or side effects were not observed in immunised mice. Body weight showed no significant difference between CPCAR groups and other groups (P > 0.05).
Figure 2. OVA-specific antibody response. IgG antibodies were measured at 7, 14 and 21 days after intramuscular immunisation (n = 5). Body weight of mice was monitored after immunisation (n = 5). (A) IgG after 7 days. (B) IgG after 14 and 21 days. (C) IgG1 and IgG2a after 21 days. (E) IgG2a/IgG1 ratio after 21 days. (F) Changes in body weight of mice. Bar graph presents the mean ± SD. *P < 0.05, **P < 0.01 and ***P < 0.001 compared with OVA; #P < 0.05 and ##P < 0.01 compared with Alum/OVA.
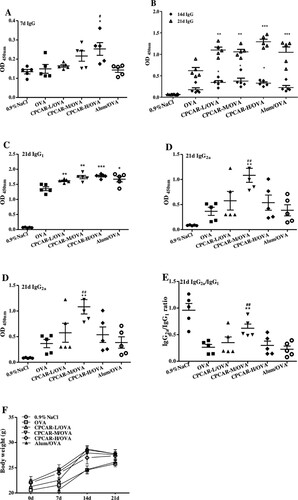
3.3. Antigen-specific lymphocyte proliferation in OVA-immunised mice
Splenocyte proliferation was determined with the MTT method after 21 days. In the groups with different concentrations of CPCAR ((A)), the group of CPCAR-H/OVA showed a significant increase of OVA-specific splenocyte proliferation when the mice were re-stimulated with OVA (P < 0.05). Similarly, the groups of CPCAR-M/OVA and CPCAR-H/OVA enhanced significantly lymphocyte proliferation under ConA or LPS restimulation (P < 0.05) and the groups of OVA alone and Alum/OVA induced a relatively low level of lymphocyte proliferation response ((B,C)).
Figure 3. OVA-specific lymphocyte proliferation response. Cells from spleen of immunised mice were isolated to measure splenocyte proliferation by MTT method after 21 days (n = 5). (A) Stimulation index of OVA. (B) Stimulation index of ConA. (C) Stimulation index of LPS. Bar graph presents the mean ± SD.*P < 0.05 and **P < 0.01 compared with OVA; #P < 0.05 and ##P < 0.01 compared with Alum/OVA.
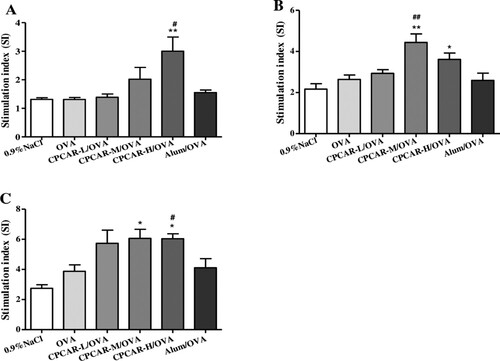
3.4. Phenotypic changes of T cells in the spleen and draining lymph nodes
CPCAR enhanced antibody response after vaccination and the induction of T cell immunity is important in the protection function against infection. Thus, we explored the impact of CPCAR on the effector/memory T cells in the spleen and lymph nodes. In the group of CPCAR-H/OVA, the ratio of CD4+ T cells to CD8+ T cells under CD3+-positive conditions and the ratio of CD44+ T cells to CD62L+ T cells under CD4+- or CD8+-positive conditions were significantly higher than those in the spleen in other groups (P < 0.05, (A–F)). A similar increasing trend was also observed in the draining lymph nodes compared with the groups of OVA alone and Alum/OVA (P < 0.05, (G–J)).
Figure 4. Activation of T cells in OVA-immunised mice. Mouse lymphocytes from spleen and draining lymph nodes were isolated for T cell subsets after 21 days (n = 5). (A–F) The percentage of CD4, CD8, CD44 and CD62L T cell subsets from spleen. (G–J) The percentage of CD4, CD8 and CD44 T cell subsets from lymph nodes. Bar graph presents the mean ± SD. *P < 0.05 and **P < 0.01 compared with OVA; #P < 0.05 and ##P < 0.01 compared with Alum/OVA.
3.5. Antigen-specific cytokines in OVA-immunised mice
In seven days after the final vaccination, cytokines in immunised animals were examined. The group of CPCAR-H/OVA showed a significant increase of IL-4 in CD4+ T cells (P < 0.05, (A)). The levels of IFN-γ in CD4+ and CD8+ T cells, the marker of TH1-biased response, in the group of CPCAR-H/OVA were significantly higher than those in the groups of OVA and Alum/OVA alone (P < 0.05, (B,C)). Alum/OVA exhibited minimal effects on only IFN-γ in CD8+ T cells.
Figure 5. Cytokine levels in OVA-immunised mice. Splenocytes from the immunised mice were stimulated with OVA and cytokines were examined after 21 days by intracellular cytokine staining (n = 5). (A) CD4+ T cell IL-4 secretion. (B) CD4+ T cell IFN-γ secretion. (C) CD8+ T cell IFN-γ secretion. Bar graph presents the mean ± SD. *P < 0.05 and **P < 0.01 compared with OVA; #P < 0.05 and ##P < 0.01 compared with Alum/OVA.
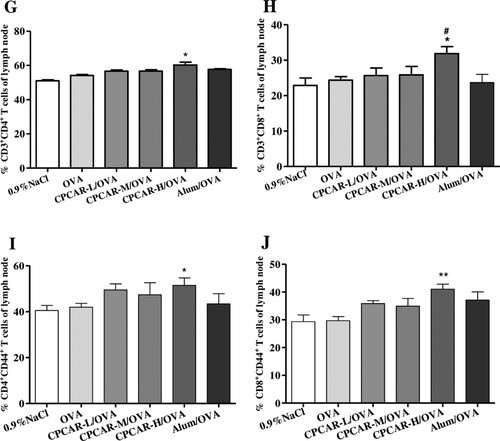
3.6. DCs maturation and Treg frequency in OVA-immunised mice
CPCAR stimulated mouse splenocytes to produce TH1/TH2 cytokines. We further explored whether CPCAR could activate adaptive immunity by regulating DC maturation and Treg frequency. The expression of surface molecules of DCs and CD4+CD25+Foxp3+ T cells in the spleen from immunised mice was detected by flow cytometers in seven days after the final vaccination. The levels of CD40, CD80, CD86 and MHC-II in the groups of CPCAR -M/OVA and CPCAR -H/OVA were higher than those in the groups of OVA and Alum/OVA (P < 0.05, (A–D)). In addition, the frequency of CD4+CD25+ Foxp3+ cells in the group of CPCAR -H/OVA was significantly lower than that in the group of OVA alone (P < 0.01, (E)).
Figure 6. Assays of DC maturation and Treg frequency in OVA-immunised mice. Splenocytes were isolated at days 21 to detect surface markers of DCs and Treg cells (n = 5). (A–D) The percentage of CD40, CD80, CD86 and MHC-II in total CD11c+ cells. (E) The percentage of CD25+ Foxp3+ in total CD11c+ cells. Bar graph presents the mean ± SD. *P < 0.05 and **P < 0.01 compared with OVA. #P < 0.05 and ##P < 0.01 compared with Alum/OVA.
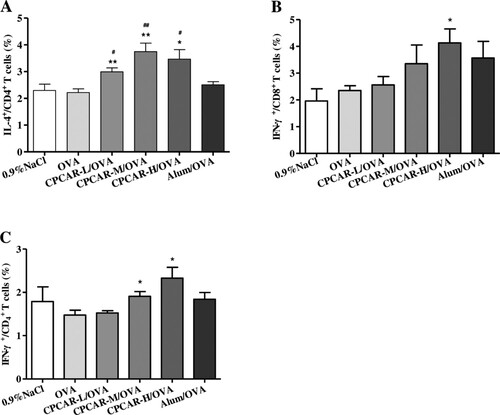
3.7. Maturation of BMDCs in vitro
To elucidate the influence of CPCAR on immune processes in antigen-presenting cells, BMDCs treated with different concentrations of CPCAR were obtained to determine the levels of surface markers. shows the ability of CPCAR to stimulate BMDC maturation. After 12-h post-stimulation, the expression levels of CD11c, CD40 and CD80 were assessed. The dendritic cell population was encircled and the subsets of CD11c+ cells were gated ((A)). The histogram profiles of double positive CD11c+CD40+ and CD11c+CD80+ in different experimental groups are exhibited in (B,D) and The quantitative representation of CD40 and CD80 positive cells displayed is provided in (C,E). Under the stimulation conditions of CPCAR, the expression levels of the two co-stimulatory molecules were significantly higher than those in the untreated group (P < 0.05), but there was no significant difference between the CPCAR group and the LPS group (P > 0.05). The expression level of CD40 or CD86 showed no significant difference between CPCAR-treated BMDCs with or without PMB pretreatment (P > 0.05, (F–I)). However, the expression levels of CD40 and CD86 was dramatically decreased in LPS-stimulated BMDCs with or without PMB pretreatment (P < 0.01).
Figure 7. Effects of CPCAR on DC maturation. BMDCs were treated with CARCP (10, 50, 100, 200 μg/mL) or LPS (100 ng/mL) for 12 h, and the levels of CD40 and CD86 on CD11c+ DCs were detected by flow cytometer (n = 3). CARCP (10, 50, 100 μg/mL) or LPS (100 ng/mL) was pre-treated with or without PMB (100 μg/mL) for 1 h and then was used to stimulate BMDCs for 12 h, and the levels of CD40 and CD86 were detected (n = 3). (A) Subsets of CD11c+ cells were gated. (B–C) The profiles of CD11c+CD40+ cells. (D–E) The profiles of CD11c+CD86+ cells. (F–G) The profiles of CD11c+CD40+ cells with or without PMB. (H–I). The profiles of CD11c+CD86+ cells with or without PMB. Bar graph presents the mean ± SD. *P < 0.05, **P < 0.01, and ***P < 0.001 compared with control group.
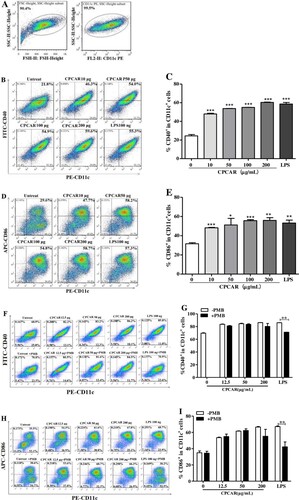
3.8. CPCAR regulated DC activation via TLR4 in vitro
To explore whether TLR4 was related to CPCAR-mediated activation of BMDCs, BMDCs were treated with TLR4 inhibitor before CPCAR or LPS stimulation. The expression levels of CD40 and CD86 have significantly up-regulated after CPCAR or LPS stimulation, but the levels of CD40 and CD86 were significantly down-regulated by the inhibition of TLR4 (P < 0.01, (A,B)). The levels of secreted cytokines (IL-12 and TNF-α) were also significantly reduced by TLR4 blockers (P < 0.01, (C,D)). The above results strongly suggested a role of TLR4 in BMDCs activation in response to CPCAR.
Figure 8. Effects of CPCAR on BMDC activation via TLR4. BMDCs were pre-treated with or without of TAK-242 for 1 h, and then incubated with CARCP (10 and 100 μg/mL) or LPS (100 ng/mL) for 12 h. The expression of CD40, CD86 on CD11c+ DCs and IL-12 were detected by flow cytometer and the level of TNF-α was analysed by ELISA kit (n = 3). (A) The percentage of CD11c+CD40+ cells. (B) The percentage of CD11c+CD86+ cells. (C) The percentage of IL-12. (D) The yield of TNF-α. Bar graph presents the mean ± SD. *P < 0.05, **P < 0.01 and ***P < 0.001 compared with TAK-242 group.
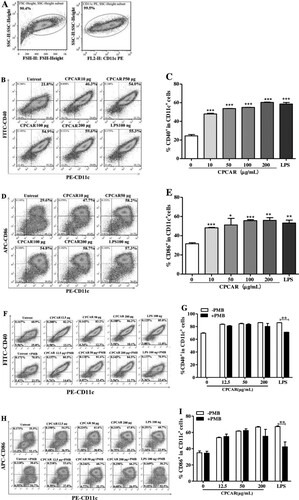
3.9. CPCAR induced DC activation via NF-κB pathway
Next, in order to assess whether CPCAR would change the levels of molecules involved in the TLR4-mediated NF-κB pathway, BMDCs were stimulated with CPCAR in the presence of an NF-κB inhibitor. PDTC significantly inhibited the expression of CD40, CD80 and MHC-II in response to CPCAR or LPS (P < 0.05, (A–C)) and the levels of secreted IL-12 and TNF-a induced by CPCAR or LPS were markedly decreased in BMDCs for PDTC pretreatment (P < 0.05, (D,E)).
Figure 9. Effects of CPCAR on NF-κB pathway in BMDCs. After pre-incubated with PDTC for 1 h, BMDCs were treated with CARCP (10 and 100 μg/mL) or LPS (100 ng/mL) for 12 h. The expressions of CD40, CD80, MHC-II on CD11c+ DCs and IL-12 were detected by flow cytometer and the yield of TNF-α detected by ELISA kit (n = 3). (A) The percentage of CD11c+CD40+ cells. (B) The percentage of CD11c+CD80+ cells. (C) The percentage of CD11c+MHC-II + cells. (D) The percentage of IL-12. (E) The yield of TNF-α. Bar graph presents the mean ± SD. *P < 0.05, **P < 0.01 and***P < 0.001 compared with PDTC group.
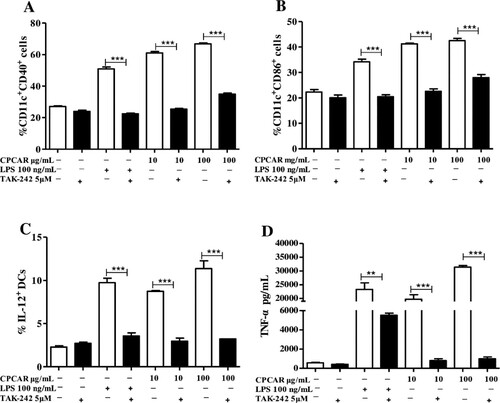
4. Discussion
According to different design strategies, adjuvants have been developed based on disease types and required immune responses. Currently, only limited aluminum-free adjuvants have been approved by FDA for the vaccines of infectious diseases, such as MF59, AS03, AS04, AS01 and CpG ODN, due to the limited exploration of new adjuvants and their mechanisms (Mckee & Marrack, Citation2017; Shi et al., Citation2019). It is widely known that herbal polysaccharides are biologically active components and may be used as immunopotentiators (Li & Wang, Citation2015). Recent discoveries have clearly demonstrated the beneficial effects of A. rupestris L. extracts on enhancing immune functions as well as ameliorating diseases including influenza and antitumor activity (Ma et al., Citation2016; Taleghani et al., Citation2020). Here, we explored the efficacy of CPCAR as an enhancer on improving immune responses and inducing DC activation by using in vitro and in vivo models. The infrared spectra of CPCAR revealed typical absorption peaks of polysaccharides. The mouse experimental data of CPCAR formulated with OVA confirmed that they could stimulate the stronger antigen-specific immune responses including the increased response of IgG, enhanced lymphocyte proliferation, increased cytokines and effector T cells and improved DC maturation. Based on these results, the mechanisms of CPCAR adjuvanticity were preliminarily discussed to explain its immunostimulatory activity in BMDCs. Measurement results of the co-stimulatory molecules and secreted cytokines indicated that blocking TLR4 and NF-κB changed the profile of BMDCs activation and led to the lower immune response, implying that CPCAR enhanced phenotypic and functional maturation of BMDC.
In order to improve the adjuvant efficacy, an appropriate dose of adjuvant is required to elicit an antigen-specific immune response and cell types. Thus, to explore the dose-response relationships, three doses of CPCAR were arranged in immunisation experiments. The kinetic profile of IgG response indicated that CPCAR increased OVA-specific antibody and subtypes and promoted the shift of antibody subtypes from TH2 (IgG1) to TH1 (IgG2a) to induce a biased TH1-type immune response. Cellular responses observed in lymphocyte proliferation assays indicated therapeutic interventions in certain diseases. Ideally, an adjuvant should be effective and safe enough to minimise side effects for clinical applications. In this study, compared to Alum adjuvant, CPCAR resulted in lower reactogenicity in the injection site. Abnormal behaviours or side effects were not observed in immunised mice in the experiment, confirming the safety improvements by intramuscular injection of CPCAR.
In addition to humoral and cellular responses, T cells play an essential role in the induction of immunity against infection. Therefore, phenotypic determinations of CD4, CD8, CD44 and CD62L T cell subsets in the spleen and draining lymph nodes of mice were also assessed in the study. CPCAR activated and potentially enhanced various types of T cells, indicating that some significant changes might account for the elevated humoral immune response and higher levels of effector T cells. Although T cell activation is crucial in the protection function against certain infections, the differential induction of TH1 response and/or TH2 response is required. Cytokines can regulate the functions of TH cells. TH1 responses with IFN-γ production is required for the protective immunity such as viruses and bacteria. TH2 responses with IL-4 production can trigger B cell proliferation and differentiation against bacterial and viral infections (Rivino, Citation2016). In this study, CPCAR improved the expression of IL-4 in CD4+ T cells, indicating that CPCAR could more efficiently induce the amplification and differentiation of CD4+ T cells in a TH2-biased response. CPCAR could remarkably increase IFN-γ production in CD4+ and CD8+ T cells and IFN-γ could induce the differentiation of CD4+ and CD8+ T cells into TH1 cells. Therefore, CPCAR was an enhancer for inducing TH1 responses, as indicated by its enhancement effect on IgG2a and IFN-γ secretion.
The above results indicated a significant increase in adaptive immune response after CPCAR administration. Moreover, CPCAR promoted the migration of T cells to secondary lymphoid organs. Therefore, it could be assumed that CPCAR might also induce DC maturation and decrease Treg frequency. DC maturation could initiate the adaptive immunity to prime T cells. The reduction of Treg could enhance humoral and cellular responses and provide a balanced immune response to vaccinations. In the study, DC maturation and Treg of spleen from immunised mice were further investigated. CPCAR up-regulated the expression levels of CD40, CD80, CD86 and MHC-II on DCs in mice, manifesting that CPCAR activated DCs and led to DC maturation, which was correlated with T cell subtype differentiation. In addition, the frequency of CD4+CD25+Foxp3+ cells was inhibited in CPCAR -treated mice, indicating that CPCAR enhanced the antigen-specific immune response by improving DC maturation and decreasing Treg frequency.
The results of in vivo experiments showed that CPCAR was an efficient stimulator of antibody responses and T cell activation. CPCAR at specific doses demonstrated immune activation. The dose-response relationship of CPCAR did not always show the dose dependency, displaying a Gauss-like curve. These data provided crucial information on the appropriate administration dose of CPCAR. The dose-response relationship of CPCAR may be associated with direct or indirect interactions with cellular and molecular events, such as DCs determining the strength and type of immunity (Kapsenberg, Citation2003). In vitro experiments provided some insight into the possible mechanism that CPCAR modulated DC function. Firstly, CPCAR -treated BMDCs markedly induced the expression levels of CD40 and CD86. The PMB pretreatment experiment showed that CPCAR was free of LPS contamination. The induction effect of CPCAR was supported by the up-regulation of the expression of co-stimulatory molecules on DCs of the spleen in mice. It was reported that the activation of immune responses by herbal polysaccharides was mediated primarily through specific receptors on DCs, such as TLR4 (Chen et al., Citation2017; Tian et al., Citation2019). Next, we then elucidated whether CPCAR treatment altered the expression levels of TLR4-mediated co-stimulatory molecules and cytokines on BMDCs. The expression levels of CD40, CD80, IL-12 and TNF-α in CPCAR -treated BMDCs were higher than those in medium-treated BMDCs and TLR4 inhibitor interrupted the up-regulation of surface molecules and cytokines on BMDCs. These results showed that activation of CPCAR -treated BMDCs was mediated by TLR4. Triggering TLRs could eventually lead to the activation of NF-κB to drive the expression of inflammatory genes (Kawai & Akira, Citation2007). The role of NF-κB in CPCAR -treated BMDCs was also assessed with an NF-κB inhibitor. CPCAR-mediated NF-κB pathway played a crucial role in the activation of phenotypic and functional maturation of BMDCs. These results demonstrated that CPCAR could induce DC activation and stimulate cytokine production via TLR4/NF-κB pathway. The effects of CPCAR were consistent with its ability to promote the differentiation of effector T cells and enhance adaptive immunity, especially TH1 response (IL-12 by murine DCs in vitro).
Overall, CPCAR as an adjuvant could promote the immunostimulatory effects of antigens, enhance the immune responses, especially TH1-biased response, and activate DCs with low toxicity and limited side effects. CPCAR could facilitate the expression of co-stimulatory molecules and cytokines on BMDCs through TLR4/NF-κB pathway (). However, the effects of CPCAR are highly complex and regulated by many factors. We will further purify CPCAR and investigate the most active compounds responsible for immunomodulatory activities for designing more effective and safer adjuvants against infectious diseases.
Acknowledgements
The authors thank Dr. Jiong Huang and Hui Cao from Tecon Biology Co., Ltd for experiment’s help. The authors wish to thank Prof. J He (Urumqi, China) for kindly providing materials. Xiang Weng and Quanxiao Li equally contributed to this work. Conceptualisation: Zhang A; Data curation: Weng X, Li Q, Feng S; Formal analysis: Weng X, Li Q; Funding acquisition: Zhang A; Investigation: Zhang A; Methodology: Zhang A, Wang B; Project administration: Zhang A; Resources: Zhang A; Software: Weng X; Supervision: Zhang A; Validation: Zhang A; Visualisation: Zhang A; Writing-original draft: Li Q, Weng X, Zhang A; Writing-review & editing: Zhang A. The animal experiments had been approved by the Committee on the Ethics of Animal Experiments of Xinjang Key Laboratory of Biological Resources and Genetic Engineering of Xinjiang University (BRGE-AE001).
Disclosure statement
No potential conflict of interest was reported by the author(s).
Additional information
Funding
References
- Akira, S., & Takeda, K. (2004). Toll-like receptor signalling. Nature Reviews Immunology, 4(7), 499–511. https://doi.org/10.1038/nri1391
- Banchereau, J., & Steinman, R. M. (1998). Dendritic cells and the control of immunity. Nature, 392(6673), 245–252. https://doi.org/10.1038/32588
- Bora, K. S., & Sharma, A. (2011). The genus Artemisia: A comprehensive review. Pharmaceutical Biology, 49(1), 101–109. https://doi.org/10.3109/13880209.2010.497815
- Chen, Y., Li, H., Li, M., Niu, S., Wang, J., Shao, H., Li, T., & Wang, H. (2017). Salvia miltiorrhiza polysaccharide activates T lymphocytes of cancer patients through activation of TLRs mediated -MAPK and -NF-κB signaling pathways. Journal of Ethnopharmacology, 200, 165–173. https://doi.org/10.1016/j.jep.2017.02.029
- Garcia-Vello, P., Speciale, I., Chiodo, F., Molinaro, A., & De Castro, C. (2020). Carbohydrate-based adjuvants. Drug Discovery Today: Technologies, 35-36, 57–68. https://doi.org/10.1016/j.ddtec.2020.09.005
- Jiang, M.-H., Zhu, L., & Jiang, J.-G. (2010). Immunoregulatory actions of polysaccharides from Chinese herbal medicine. Expert Opinion on Therapeutic Targets, 14(12), 1367–1402. https://doi.org/10.1517/14728222.2010.531010
- Jin, M., Zhao, K., Huang, Q., & Shang, P. (2014). Structural features and biological activities of the polysaccharides from Astragalus membranaceus. International Journal of Biological Macromolecules, 64, 257–266. https://doi.org/10.1016/j.ijbiomac.2013.12.002
- Kapsenberg, M. L. (2003). Dendritic-cell control of pathogen-driven T-cell polarization. Nature Reviews Immunology, 3(12), 984–993. https://doi.org/10.1038/nri1246
- Kawai, T., & Akira, S. (2007). Signaling to NF-κB by toll-like receptors. Trends in Molecular Medicine, 13(11), 460–469. https://doi.org/10.1016/j.molmed.2007.09.002
- Li, J., Li, J., & Zhang, F. (2015). The immunoregulatory effects of Chinese herbal medicine on the maturation and function of dendritic cells. Journal of Ethnopharmacology, 171, 184–195. https://doi.org/10.1016/j.jep.2015.05.050
- Li, P., & Wang, F. (2015). Polysaccharides: Candidates of promising vaccine adjuvants. Drug Discovery Today: Technologies, 9, 88–93. https://doi.org/10.5582/ddt.2015.01025
- Li, X., Zhao, R., Zhou, H. L., & Wu, D. H. (2011). Deproteinization of polysaccharide from the Stigma Maydis by Sevag method. Advanced Materials Research, 340, 416–420. https://doi.org/10.4028/www.scientific.net/AMR.340.416
- Ma, L.-L., Wang, H.-Q., Wu, P., Hu, J., Yin, J.-Q., Wu, S., Ge, M., Sun, W.-F., Zhao, J.-Y., Aisa, H. A., Li, Y.-H., & Jiang, J.-D. (2016). Rupestonic acid derivative YZH-106 suppresses influenza virus replication by activation of heme oxygenase-1-mediated interferon response. Free Radical Biology and Medicine, 96, 347–361. https://doi.org/10.1016/j.freeradbiomed.2016.04.021
- Ma, Y., Aisha, H.A., Liao, L., Aibai, A., Zhang, T., & Ito, Y. (2005). Preparative isolation and purification of rupestonic acid from the Chinese medicinal plant Artemisia rupestris L. by high-speed counter-current chromatography. Journal of Chromatography A, 1076, 198–201.
- Mckee, A. S., & Marrack, P. (2017). Old and new adjuvants. Current Opinion in Immunology, 47, 44–51. https://doi.org/10.1016/j.coi.2017.06.005
- Park, J.-M., Hahm, K.-B., Kwon, S.-O., & Kim, E.-H. (2013). The anti-inflammatory effects of acidic polysaccharide from Artemisia capillaris on Helicobacter pylori Infection. Journal of Cancer Prevention, 18(2), 161–168. https://doi.org/10.15430/JCP.2013.18.2.161
- Ren, W., Liang, P., Ma, Y., Sun, Q., Pu, Q., Dong, L., Luo, G., Mazhar, M., Liu, J., Wang, R., & Yang, S. (2021). Research progress of traditional Chinese medicine against COVID-19. Biomedicine & Pharmacotherapy, 137(12), 111310. https://doi.org/10.1016/j.biopha.2021.111310
- Riaz, M., Rahman, N. U., Zia-Ul-Haq, M., Jaffar, H. Z. E., & Manea, R. (2019). Ginseng: A dietary supplement as immune-modulator in various diseases. Trends in Food Science & Technology, 83, 12–30. https://doi.org/10.1016/j.tifs.2018.11.008
- Rivino, L. (2016). T cell immunity to dengue virus and implications for vaccine design. Expert Review of Vaccines, 15(4), 443–453. https://doi.org/10.1586/14760584.2016.1116948
- Sahu, N., Meena, S., Shukla, V., Chaturvedi, P., Kumar, B., Datta, D., & Arya, K. R. (2018). Extraction, fractionation and re-fractionation of Artemisia nilagirica for anticancer activity and HPLC-ESI-QTOF-MS/MS determination. Journal of Ethnopharmacology, 213, 72–80. https://doi.org/10.1016/j.jep.2017.10.029
- Shi, S., Zhu, H., Xia, X., Liang, Z., Ma, X., & Sun, B. (2019). Vaccine adjuvants: Understanding the structure and mechanism of adjuvanticity. Vaccine, 37(24), 3167–3178. https://doi.org/10.1016/j.vaccine.2019.04.055
- Sun, B., Yu, S., Zhao, D., Guo, S., Wang, X., & Zhao, K. (2018). Polysaccharides as vaccine adjuvants. Vaccine, 36(35), 5226–5234. https://doi.org/10.1016/j.vaccine.2018.07.040
- Taleghani, A., Emami, S. A., & Tayarani-Najaran, Z. (2020). Artemisia: A promising plant for the treatment of cancer. Bioorganic & Medicinal Chemistry, 28(1), 115180. https://doi.org/10.1016/j.bmc.2019.115180
- Tian, H., Liu, Z., Pu, Y., & Bao, Y. (2019). Immunomodulatory effects exerted by poria cocos polysaccharides via TLR4/TRAF6/NF-κB signaling in vitro and in vivo. Biomedicine & Pharmacotherapy, 112, 108709. https://doi.org/10.1016/j.biopha.2019.108709
- Tomar, J., Patil, H. P., Bracho, G., Tonnis, W. F., Frijlink, H. W., Petrovsky, N., Vanbever, R., Huckriede, A., & Hinrichs, W. L. J. (2018). Advax augments B and T cell responses upon influenza vaccination via the respiratory tract and enables complete protection of mice against lethal influenza virus challenge. Journal of Controlled Release, 288, 199–211. https://doi.org/10.1016/j.jconrel.2018.09.006
- Wang, D., Cao, H., Li, J., Zhao, B., Wang, Y., Zhang, A., & Huang, J. (2019). Adjuvanticity of aqueous extracts of Artemisia rupestris L. for inactivated foot-and-mouth disease vaccine in mice. Research in Veterinary Science, 124, 191–199. https://doi.org/10.1016/j.rvsc.2019.03.016
- Zhang, A., Li, J., Zhao, G., Geng, S., Zhuang, S., Wang, B., & Zhang, F. (2011). Intranasal co-administration with the mouse zona pellucida 3 expressing construct and its coding protein induces contraception in mice. Vaccine, 29(39), 6785–6792. https://doi.org/10.1016/j.vaccine.2010.12.061
- Zhang, A., Wang, D., Li, J., Gao, F., & Fan, X. (2017a). The effect of aqueous extract of Xinjiang Artemisia rupestris L. an influenza virus vaccine adjuvant) on enhancing immune responses and reducing antigen dose required for immunity. PLoS One, 12, e0183720. https://doi.org/10.1371/journal.pone.0183720
- Zhang, A., Yang, Y., Wang, Y., Zhao, G., Yang, X., Wang, D., & Wang, B. (2017b). Adjuvant-active aqueous extracts from Artemisia rupestris L. improve immune responses through TLR4 signaling pathway. Vaccine, 35(7), 1037–1045. https://doi.org/10.1016/j.vaccine.2017.01.002
- Zhu, N., Lv, X., Wang, Y., Li, J., Liu, Y., Lu, W., Yang, L., Zhao, J., Wang, F., & Zhang, L. W. (2016). Comparison of immunoregulatory effects of polysaccharides from three natural herbs and cellular uptake in dendritic cells. International Journal of Biological Macromolecules, 93, 940–951. https://doi.org/10.1016/j.ijbiomac.2016.09.064