Abstract
Heavy metals in the site received industrial effluents were investigated to assess the pollution levels, distribution of metal among solid-phase fractions and possible metal sources. The soil samples at different depths of 0–5, 5–25 and 25–50 cm were collected and analyzed for Fe, Mn, Cd, Zn, Cu, Ni and Pb. Among all metals, Cd content was not detected in all soil samples. The average contents of Pb and Zn are higher than the corresponding values of common range in earth crust. Meanwhile, the maximum contents of Cu and Zn are higher than those of Dutch optimum value but lower that the Dutch protection act target value. The maximum contents of Cu, Pb and Zn are higher than the average shale value. The most investigated heavy metals are mostly found in the potentially labile pool (>50.0%) including metal bound to carbonate, Fe/Mn oxides, or organically fractions. Enrichment factor (EF) in combination with multivariate analysis including principal component analysis (PCA) and hierarchical cluster analysis (HCA) suggest that Mn and Ni associated with Fe in the soil samples were primarily originated from lithogenic sources. Pb was largely derived only from anthropogenic source, while Cu and Zn in the soil samples were controlled by the mixed natural and anthropogenic sources. These results suggest that discharging the industrial effluents into dumping site increased pollution level of Pb, Zn and Cu as well as enhanced their potentially labile pool that may be responsible for occurring potential toxic impacts on environmental quality.
1. Introduction
Urban areas contain high levels of pollutants due to high human population density and activities, and it has been reported that the sources of environmental pollution in urban areas are varied such as traffic, industrial emissions and waste, energy production processes, burning of fossil fuels and municipal wastes, disposal of urban wastes, and various other human activities [Citation1–3]. Among anthropogenic sources, solid and liquid wastes derived from industrial processes are considered to be one of the most dangerous outcomes. These wastes contain industrial pollutants including greases, acids, alkalis, suspended solids, heavy metals, salts, detergents, cyanide, solvents, halogenated organic matter and nutrients, and other chemicals. Industrial emissions and wastes could represent the most important source of soil pollution [Citation4,5]. The accumulation of heavy metals in soils can significantly reduce environmental quality with public health implications, mainly due to their toxicity and nature of non- biodegradability [Citation3].
The industrial effluents contain solid suspends, which vary in their size and chemical composition as well as physical characteristics. Therefore, in addition to increasing heavy metals levels, the accumulation of such effluents on the soil surface might change the physico-chemical properties of the soil, which are responsible for altering the forms of heavy metals and thus increased or decreased heavy metals mobility. The soil received industrial effluents can be considered a source of pollutants as well as they have the ability to transport contaminants into the groundwater and the food chain, creating serious human health issues [Citation5,6]. Several investigators reported that the disposal of industrial effluents into soil leads to the accumulation of heavy metals in soil and subsequently, it could result in higher than normal concentrations of heavy metals in plant tissues [Citation6–8]. Therefore, potential toxic effects of heavy metals are possible on environmental quality. In a study conducted by Awomeso et al. [Citation6] to investigate impact of industrial effluents on water, soils and plants, industrial effluents resulted in increasing metal levels higher than the recommended limits in effluent discharges in all the investigated samples in the study area. So, it is of importance to assess the levels and mobility of heavy metals in dumpsite soils located in urban areas for the prevention and control soil and ground water from long term pollution loading.
In addition to quantify their concentrations, it is of importance to identify the heavy metals sources. Heavy metals in different environmental compartments can be originated from lithogenic and/or anthropogenic sources [Citation9]. It has been reported that there are several methods to identify metal sources such as metal solid-phase fractions, profile distribution, and soil spatial variability. However, these methods might be not sufficiently reliable to identify the sources based on heavy metal content [Citation10]. Recently, correlation matrix and multivariate analyses including the principal component analysis (PCA) and hierarchical cluster analysis (HCA) have been introduced to make distinguishing between lithogenic and anthropogenic origin of heavy metals [Citation9,11–16].
Due to intense urbanization and industrialization, the environmental pollution with heavy metals is a serious problem in Saudi Arabia. To our knowledge, in Saudi Arabia, there is insufficient information and research on heavy metals content and their mobility in dumpsites received industrial effluents. Thus, it is very necessary to assess dumping site soils of industrial effluents to ensure environmental protection and sustainability for long periods. Therefore, the objectives of the current study are: (1) to assess the heavy metals levels of dumpsite soils received industrial effluents in the city of Riyadh, Saudi Arabia, (2) to determine the distribution of heavy metals among various solid-phase fractions as well as their overall lability, and (3) to identify heavy metals sources using enrichment factor and multivariate statistical analyses.
2. Materials and method
2.1. Areas of study, soil sampling and analysis
There are two industrial cities including more than 2150 factories at Riyadh city, Saudi Arabia. In the current study, the investigated area that received industrial effluents is located at the northern second industrial city in Riyadh. This dumping site for industrial effluents is estimated to 727.4 km2. The investigated dumping site is considered as an area for receiving liquid waste derived from pulp, textile, or plating industries [Citation17]. This area is located on longitude (E) 46° 55′, and latitude (N) 24° 32′.
For soil sampling, the dumping site area was divided into 17 square grids (Figure ). Soil samples of the study area were collected from the three depths (0–5, 5–25, and 25–50 cm). From each square grid, five soil samples of each depth were collected and then, mixed together for one large representative sample at each depth. A total of fifty-one soil samples were collected from study area. The soil samples were air-dried at room temperature, and sieved through a 2 mm screen. The particle size distribution was determined by the hydrometer method [Citation18]. Soil pH was measured using a glass electrode in a suspension of 1:5 ratio of soil to water. Electrical conductivity (EC) was measured in the soil extracts of soil to water ratio of 1:5. Calcium carbonate (CaCO3) content was determined using a calcimeter. Physico-chemical soil properties in the studied area are presented in Table . The average pH values of soil samples accounted for 7.6, 7.7 and 7.8 at 0–5, 5–25 and 25–50 cm depth. The EC values ranged between 0.2–4.4, 0.2–4.3 and 0.1–6.4 dSm−1 at 0–5, 5–25 and 25–50 cm depth, respectively. The most soil samples have soil texture of loamy sand or sandy loam. The content of CaCO3 varied in the studied soil samples from 8.8 to 54.4% with an average of 18.0, 19.9 and 21.7% at 0–5, 5–25 and 25–50 cm depth, respectively.
Table 1. Descriptive statistics of physico-chemical soil properties.
The total metal (Fe, Mn, Cd, Zn, Cu, Ni and Pb) content in soils was determined following the digestion method of Hossner (HF–H2SO4–HClO4) [Citation19] and their concentrations were analyzed using Inductively Coupled Plasma Optical Emission Spectrometer (ICP-OES, Perkin Elmer Optima 4300 DV, USA). The chemical fractionation method according to Kashem and Singh [Citation20] and modified from Tessier et al. [Citation21] was applied in the current study. This method fractionated heavy metals into six fractions including water extractable metal (F1), exchangeable form (F2), carbonate bound metal (F3), Fe/Mn oxides bound metal (F4), organically bound metal (F5) and residual fraction (F6).
2.2. Enrichment factor
In this study, enrichment factors (EF) for soils were calculated with respect to Fe, using the following definition [Citation22].
where (Cx/CFe) soil is the ratio of concentration of the tested metal in the soil sample (Cx) to that of (CFe) in the soil sample; and (Cx/CFe) earth’s crust is the ratio of metal concentration Cx to Fe in the reference Earth’s crust according to Taylor [Citation23]. In this study, earth crust was selected as the reference value because the background values of heavy metals have not yet been reported for the study area. Five pollution levels based on Enrichment values have been proposed to be as follows: EF ≤ 2 represents no or minimal pollution; 2 < EF < 5 moderate pollution; 5 < EF < 20, significant pollution; 20 < EF < 40, strong pollution and EF > 40 indicates extreme pollution.
2.3. Lithogenic and anthropogenic contributions
The heavy metal contribution of lithogenic and anthropogenic were calculated using the equation by Hernandez et al. [Citation24]. To calculate the metal content of lithogenic, the following equation was used:
where [M]lithogenic: Metal concentration of lithogenic origin in the sample (mg kg−1); [Fe]sample: the total content of Fe in soil samples (mg kg−1); and ([M]/[Fe])lithogenic: the ratio of metal concentration and iron in the earth’s crust. Moreover, the anthropogenic heavy metal content was calculated using the following equation:
where [M]anthropogenic: the anthropogenic heavy metal content; and [M]total: the total content of heavy metal measured in soil samples.
2.4. Quality assurance and quality control
The collected soil samples were digested and analyzed in four replicates. For quality control of soil heavy metals analysis, four reference soils (Till-1 and Till-4) were used in the current study. The recoveries of heavy metals by the Hossner digestion method for Till-1 and Till-4 were 66 and 66% for Fe, 83 and 79% for Mn, 85 and 87% for Zn, 132 and 92% for Cu, 163 and 173% for Ni, and 29 and 50% for Pb, respectively. However, Cd content of Till-1 and Till-4 was under detection limit of ICP-OES. To ensure the analytical performance of the ICP-OES, a standard of heavy metals concentrations was run after every ten samples.
2.5. Statistical analysis
The values of minimum, maximum, mean, median, standard deviation (±SD), skewness and coefficient of variation for the obtained data are calculated and reported. The multivariate analysis including the PCA and HCA was performed to identify the relationship among investigated heavy metals and their sources. The statistical analysis was performed by using statistical software Statistica for Windows [Citation25].
3. Results and discussion
3.1. Descriptive statistics of soil heavy metal content
The statistical summary of the total metal content in soil samples with depth is given in Table . The data show that Fe is the dominant heavy metal but Cd was not detectable in the collected soil samples. Among other investigated metals, Pb, Cu and Ni have lower content than Mn and Zn. Based on maximum and mean content, the highest Cu and Pb were pronounced at the surface soil sample of 0–5 cm depth, while the highest Zn was recorded at the second layer of 5–25 cm depth. Due to its higher mobility, Zn may be easily transferred downward with percolating water and thus, accumulation in subsurface layers of the soil [Citation26]. It was observed that the concentrations of Cu, Pb and Zn showed high coefficient of variations and positive skewness, suggesting that there is a strong skewness factor for these heavy metal. However, Fe and Mn content in surface soil samples show much lower skewness with negative skewness values than in subsurface soil samples.
Table 2. Descriptive statistics of heavy metal content in soil samples.
Table also shows that the average contents of Pb and Zn are higher than the corresponding values of common range in earth crust reported by Lindsay [Citation27]. In addition, the maximum contents of Cu and Zn are higher than those of Dutch optimum value but lower that the Dutch Protection Act target value [Citation28]. The maximum contents of Cu, Pb and Zn are higher than the average shale value [Citation29].
3.2. Solid-phase fractions and overall heavy metal lability
Heavy metals can be found in various solid-phase fractions in soil, exhibiting different soil physico-chemical behavior in relation with chemical reaction, lability, bioavailability and metal toxicity. Therefore, it is very important to quantify the metal species among various solid-phase compartments to assessing metal mobility and transport with changing environmental conditions. The major mechanisms and possesses responsible for metal accumulation and distribution in soils provide various forms of heavy metals including water soluble, exchangeable, bound to carbonate, bound to reducible phases (iron and manganese), bound to organic matter and residual. These six forms have different behaviors in relation with solubilization and remobilization with changing environmental conditions.
Figure shows percentage of heavy metals fractions among various solid-phases. The fractions 1 and 2 exhibit the soluble and exchangeable metal forms, respectively. The results showed the lowest quantity of these two fractions for all metals. However, the fraction in phase 3 bound to carbonate and in which there are higher percentages of Pb (16.6–32.3%), Mn (25.4–26.3%), Cu (6.3–18.8%) and Zn (9.6–17.6%) in comparison to Fe (<1%) and Ni (2.9–3.5%). This may be due to heavy metals affinity to co-precipitate with carbonate minerals under alkaline conditions [Citation30,31]. The results indicated that Cu, Zn and Pb bound to carbonate and exchangeable fractions were changed with soil sampling depth. It was observed that these three heavy metals bound to the carbonate and exchangeable fraction showed lower proportions in the third layer (25–50 cm depth) than those of surface layers (0–5 cm and 5–25 cm). The percentage of Cu bound to carbonate fraction decreased from 18.8% at the first layer (0–5 cm depth) of the soil to 12.0 and 6.34% at the second layer (5–25 cm depth) and the third layer (25–50 cm depth), respectively. The percentage of Pb bound to carbonate fraction accounted for 28.7% (at 0–5 cm depth) and 32.3% (at 5–25 cm depth), which decreased to 16.6% at the lowest layer of 25–50 cm depth. The percentage of Zn bound to carbonate fraction accounted for 17.0 and 17.7% (at 0–5 cm and 5–25 cm depth, respectively), which decreased to 9.6% at the lowest layer of 25–50 cm depth.
Figure 2. Percentage of heavy metals fractions among different solid phases (a: at soil sampling depth of 0–5 cm; b: at soil sampling depth of 5–25 cm; c: at soil sampling depth of 25–50 cm).
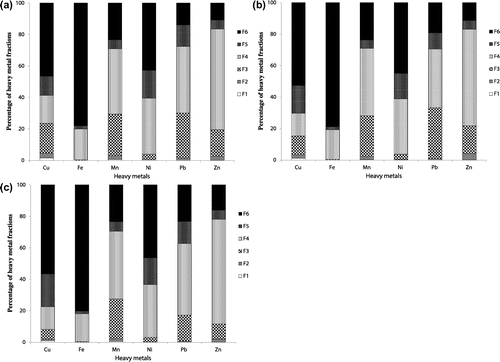
The fraction bound to the easily reducible Fe/Mn oxides has been reported to be in relation with anthropogenic discharges for heavy metals [Citation30,32,33]. The results showed that this fraction retained 61.3–66.4% of Zn followed by 41.4–42.8% of Mn and 37.2–45.4% of Pb, which were higher than those for Ni 33.5–35.5%, Fe 17.7–19.6%, and Cu 14.3–17.8%. This result indicates the highest quantity of Zn associated with Fe/Mn oxides among all heavy metals. Several other researchers have also reported that Zn was bound to Fe–Mn oxides [Citation34–36]. Our results suggest that metals associated with Fe–Mn oxides could be remobilized and released in case of reduced conditions. In the current study, there were no obvious changes in the content of most investigated heavy metals bound to the easily reducible Fe/Mn oxides fraction along the soil depth. However, some changes in Pb bound to the easily reducible Fe/Mn oxides fraction were observed along the soil depth. The results indicated that Pb bound to the easily reducible Fe/Mn oxides fraction showed the lowest percentage (37.2%) at the second layer (5–25 cm depth) and the highest percentage (45.4%) at the third layer (25–50 cm depth).
The metal in F5 is mainly bound to organic matter. It was found that organic matter fraction has a higher significant quantity of Cu (12.2–20.8%) and Ni (16.1–17.8%) than those for Pb 10.2–14.1%, Mn 5.4–6.1%, Fe 1.74–2.0% and Zn 5.5–5.9%. The results indicated that Cu bound to organic matter tended to increase with increasing soil sampling depth. The percentage of Cu bound to organic matter increased from 12.2% at the surface soil sample of 0–5 cm depth to 17.7% at the second layer of 0–5 cm depth and to 20.8% at the third layer of 25–50 cm depth. The relatively higher increases in Cu quantity associated with organically fraction might be attributed to its high affinity to organic matter through organo-metal complexes formation [Citation37–39].
Heavy metal bound to F6 is corresponding to the residual fraction (geochemical phase), indicating more chemically stable fraction and inactive in terms of biological processes. There are high percentages of Fe (78.0–80.2%), followed by Cu (46.5–56.6%) and Ni (42.8–46.4%) in comparison to Mn (23.4–23.7%), Pb (14.0–23.3%) and Zn (10.9–16.1%). Higher percentage of Fe and/or Cu associated with residual fraction were reported by other researchers [Citation35,40]. The high proportion of Cu in the residual fraction might be attributed to the ability of Cu to substitute Fe and Mg in the structure of alumino-silicate minerals [Citation34,41]. The results indicated also that Cu, Zn and Pb in phase 6 bound to residual fraction tended to increase with increasing soil sampling depth. The percentage of Cu, Zn and Pb bound to residual fraction increased from 46.5, 10.9 and 14.0% at the first layer (0–5 cm depth) of the soil to 52.6, 11.4 and 19.3% at the second layer (5–25 cm depth) and to 56.6, 16.1 and 23.3% at the third layer (25–50 cm depth), respectively.
Based on metal fractionation, the heavy metals fractions among solid-phase components were determined and grouped into three pools including the readily labile, potentially labile and non-labile pools to predict overall lability of heavy metals [Citation34]. The concentrations of heavy metals in water soluble and exchangeable fractions are considered as a readily labile pool, and those of the carbonate, Fe/Mn oxides, and organically fractions are considered as a potentially labile pool. However, the concentrations of heavy metals in the residual fraction are considered as a non-labile pool.
The results showed the lowest content of readily labile pool for all heavy metals, which varied in the studied soil samples from 0.051 to 4.7% (Figure ). A high soil pH and calcium carbonate content of soil samples could be responsible for lowering the concentrations of heavy metals in the readily labile pool (water soluble + exchangeable phase) through metal sorption, mainly by precipitation [Citation31,42]. However, the most investigated heavy metals are mostly found in the potentially labile fractions (>50.0%). The order of potentially labile fractions for the metals was Zn (81.9–86.5%) > Pb (76.0–84.6%) > Mn (73.4–73.9%) > Ni (53.4–56.6%) > Cu (41.4–48.8%) > Fe (19.7–21.9%) in overall soil samples depth. High percentage of mobile forms (non-residual fraction) in comparison with residual fraction (immobile form) has been reported by Ogundiran and Osibanjo [Citation43], mainly due to soil contamination by anthropogenic inputs. Among all investigated metals, Fe (78.0–80.2%) was found to be more in the residual fraction (non-labile form) of soil samples than those of potentially labile form. Additionally, the most quantity of Cu was distributed between potentially labile (41.4–48.8%) and residual (46.5–56.6%) fractions, depending on soil sampling depth. Our results suggest that the highest proportions of most investigated metals associated with the potentially labile pool including Fe–Mn oxide, carbonate and organic matter fractions may result in potential toxic impacts on environmental quality through solubilization and remobilization of heavy metals bound to this pool. In this context, heavy metals of the potentially labile pool are highly dependent upon soil properties and environmental factors including the redox potential and pH [Citation30].
3.3. Identification of heavy metal enrichment and sources
The enrichment factor (EF) has been reported to be as a measure for determining heavy metal source and comparing the earth crust metals abundance with those found in soil samples [Citation11,14]. Table shows descriptive statistics of heavy metal EF. It was observed that the average EF values of an individual metal in the soil samples were decreased in the following order: Zn (0.0–26.8) > Pb (0–15.3) > Cu (1.1–12.0) > Ni (1.4–3.0) > Mn (0.7–1.2). The results indicated that the values of EF for heavy metals were changed with soil sampling depth. It was observed that the average values of EF for Cu, Pb and Zn at the first surface layer (0–5 cm depth) and the second layer (5–25 cm depth) were higher than those of the third layer (25–50 cm depth). Based on the maximum values of EF, soil samples were found to present significant pollution for Zn, Pb and Cu, and to represent moderate pollution for Ni and no or minimal pollution for Mn. The average values of an EF for Zn, Pb and Cu were much higher than 2, suggesting that their contaminations may be of concern. It has been previously suggested that a value of EF ≤ 2 indicates that heavy metal could not be impacted by anthropogenic sources and it is mainly controlled by the parent materials of the soils and natural processes of weathering. On the contrary, soil samples having EF values greater than 2 suggest that the environmental existence of heavy metal is of human activities rather than the result of lithogenic origin [Citation24]. Thus, the heavy metals of Ni and Mn with much lower EF in soil samples may be as a consequence of the parent materials or natural soil forming factors and processes (Table and Figure ). However, the EF values of heavy metals of Zn, Pb and Cu greater than 2 in the most soil samples suggest that these three heavy metals were strongly impacted by anthropogenic inputs. In line with the results of EF values, the calculated lithogenic and anthropogenic proportion of these metals showed higher significant anthropogenic contribution >50% (especially Pb and Zn with much higher proportion). This suggests that these metals might be mainly introduced into the soils through anthropogenic sources and the environmental metal contamination of industrial effluents might be occurred.
Table 3. Descriptive statistics of heavy metal enrichment factor (EF).
Table shows the correlation matrix of the heavy metals at the 0.05 significance level in the soil samples. The simple correlation analysis showed that there is a positive significant correlation between Fe and Mn with correlation coefficient (r) accounting for 0.85, indicating a strong linear correlation. The strong correlations between these two metals suggest that they may come from the same source. It has been reported that Fe and Mn take place naturally at great quantities and are thus seldom affected by anthropogenic contributions [Citation14], inferring their strong correlation in the soil samples. Ni is also considered as an element of naturally occurrence in earth crust and its strong correlation with Fe (r = 0.74) and Mn (r = 0.68) indicate that its occurrence could mainly be naturally. Cu showed significant positive correlations with Fe (r = 0.33), Mn (r = 0.33) and Ni (r = 0.53). Similarly, Zn exhibited positive correlations with Fe (r = 0.56), Mn (r = 0.33) and Ni (r = 0.50). However, there is no relationship between Zn and Cu. Among all heavy metals, Pb did not correlate significantly with any metal, suggesting that its sources and behaviour may be different from those of the others.
Table 4. Pearson’s correlation matrix of heavy metals in the soil samples.
The PCA is documented to be a useful statistical tool to distinguish heavy metals sources [Citation11,12]. The results of PCA for the contents of heavy metals are shown in Table . Based on the obtained results of PCA, only the two first components having eigenvalues of >1.0 before and after the matrix rotation were presented. The two first components explained 69.07% of the total variance (Table ). The first component explained 51.75% of the total variance including Fe, Mn and Ni with factor loads of 0.91, 0.83, and 0.90, respectively. The positive factor loads for Fe, Mn and Ni suggests these three metals come from similar sources, mainly lithogenic contribution. The second component explained 17.50% of the total variance including only Pb. The 2-D plots (PC1 vs. PC2) of PCA showed that the investigated heavy metals can be classified into different sources (Figure ). The results indicated that the first principal component (PC1) is characterized by Fe, Mn, Ni and also Cu and Zn. The results also showed that Cu and Zn showed a lower contribution to PC1 than Fe, Mn, and Zn. Among these five metals, Fe, Mn and Ni in PC1 showed a high similarity with the highest significant loadings (>0.83), indicating a similar source. Similarly, several other researchers found that Fe, Mn or Ni were found to be associated with parent rocks, indicating that these heavy metals were related with the soil-forming factors and suggesting their natural origin [Citation9,11,14,15,44]. Additionally, in the obtained PCA loading 2-D plots of the current study, Cu and Zn and a group of Fe, Mn and Ni in PC1 are separated by a distance with significant correlation between them. Since Cu and Zn showed a combined relationship with Fe, Mn and Ni and lower contribution to PC1, they may have mixed origin. However, Pb and a group of other metals are separated by a long distance in PCA loading 2-D plots. Additionally, Pb showed the highest loading (0.98) in PC2 with no significant correlation with other investigated heavy metals of Fe, Mn, Cu, Zn and Ni, suggesting different behaviour and probably another different source. Our findings are almost comparable to other studies. In a study conducted by Almasoud et al. [Citation11] to assess heavy metal sources in the soils affected by industrial activities using multivariate analysis, there is a high loading of Fe, Mn, Ni, Co, and Cr, indicating that these heavy metals are is of lithogenic origin. However, the contents of Zn, Cu, and Pb are controlled by anthropogenic activity. Ayoubi et al. [Citation9] also found that Fe, Mn, Ni, Co and Cr were associated in the lithogenic factor (factor 1), while Pb, Cu and Zn showed high loadings in the anthropogenic factor (factor 2). These findings are also partially in agreement with the results reported by Taghipour et al. [Citation15], who found that the contents of Co, Cr, and Ni were associated with the lithogenic factor. Their results also indicated that both Zn and Pb contents were associated with the anthropogenic factor and Cu was associated with the lithogenic-anthropogenic factor. Hierarchical cluster analysis was also applied in the current study to identify the relatively homogeneous groups of heavy metals. Figure shows that Mn and Fe were significantly and strongly correlated with each other and formed a cluster. This cluster was associated with Ni. This indicates similar distribution pattern for Fe, Mn and Ni.
Table 5. Total variance and factor loadings of the contents of heavy metals for the first two components having eigenvalue of >1.
Figure 6. Cluster dendogram for heavy metals by Ward’s method (Pearson’s r) at overall soil sampling depths.
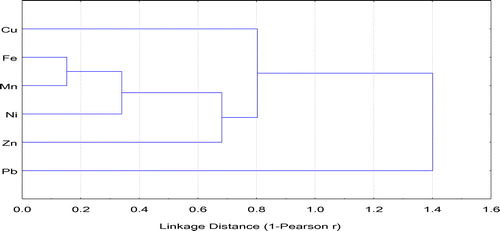
Based on PCA, HCA, EF and anthropogenic contribution analyses, the obtained results suggest that the heavy metals in the soils received industrial effluent could be classified into three groups including metals derived from lithogenic, anthropogenic and mixed sources. The first group consists of Fe, Mn and Ni, which is found in the first factor of PCA that is of lithogenic origin and their variability can mainly controlled by the parent materials of the soils. The median EF values of Mn and Ni in the overall soil sampling depth were about 0.9 and 2.0, respectively, with about 100 and 60% of the soil samples having EF values of less than 2 (Table and Figure ). Only about 40% of the soil samples had EF values of Ni greater than 2 (i.e. moderate pollution) but they mostly are still close to 2 and 60% of the soil samples having EF values of <2, indicating that soil contamination by Ni was limited. As indicated from the data of anthropogenic contribution, the three metals (Fe, Mn, and Ni) of the first factor in PCA showed a significant lithogenic contribution, being mainly controlled by soil-forming factors. The second group includes Pb, which showed the highest loading in PC2 and did not correlate significantly with any metal. The median EF values of Pb in the overall soil sampling depth varied from 4.3 to 8.7 with about 74.5% of the soil samples having EF values of >2 and anthropogenic contribution greater than 50%. The enriched levels of Pb in the investigated soil samples suggest that it is originated largely from anthropogenic sources, mainly due to discharge industrial effluents. Therefore, Pb can be termed the anthropogenic factor. The third group contains Cu and Zn, which may come from mixed natural and anthropogenic sources. It was noted that 84.3 and 90.2% soil samples, respectively, contained EF values of Zn and Cu greater than 2 with anthropogenic contribution greater than 50%, indicating anthropogenic origin. On the other hand, these two heavy metals showed significant correlation with Fe, Mn and Ni, indicating Cu and Zn can also be controlled and attributed to lithogenic sources. Therefore, a mixed source (lithogenic and anthropogenic inputs) is possible for Cu and Zn. Other researchers also confirmed high anthropogenic contribution and enrichment of Pb, Zn, and Cu in soils affected by industrial activities [Citation4,9,11]. Pandey et al. [Citation5] investigated the accumulation of heavy metals in soil irrigated with industrial effluent and their results revealed that Cr, Cu and Pb were in higher content in soils. They reported that these metals are mainly anthropogenic metals from industrial effluents, suggesting their direct impacts on accumulation of these metals in the plant. Awomeso et al. [Citation6] also found that the soil affected by industrial effluent contained higher concentrations of heavy metals such as Pb, Cu and Zn as compared with the reference soil. The results of the current study concluded that discharging the industrial effluents into soils increased enriched levels of Pb, Zn and Cu as well as enhanced their concentrations in the potentially labile pool that may be responsible for occurring potential toxic impacts on environmental quality through remobilization of such heavy metals.
4. Conclusions
The accumulation of Fe, Mn, Pb, Zn, Cu and Ni were investigated at site received industrial effluents. Based on maximum value of EF, soil samples were found to represent significant pollution for Zn, Pb and Cu, moderate pollution for Ni and no or minimal pollution for Mn. Enrichment factor in combination with correlation matrix and multivariate analyses suggests that the heavy metals in the soils received industrial effluents could be classified into three groups including metals derived from lithogenic (Fe, Mn, and Ni), anthropogenic (Pb) and mixed sources of lithogenic-anthropogenic (Cu and Zn). Therefore, the industrial effluents should be discharged into the environment after proper remediation to prevent and control soil and surrounding environment from long term pollution loading. Future studies in relation with dumpsites received industrial effluents should focus on the investigation of the pollution levels of heavy metals in the surrounding soils as well as groundwater.
Disclosure statement
No potential conflict of interest was reported by the authors.
Funding
This work was supported by King Abdulaziz City for Science and Technology and King Saud University.
Acknowledgments
The authors wish to thank King Abdulaziaz City for Science and Technology and King Saud University, the Deanship of Scientific Research, the College of Food and Agriculture Science, and the Research Centre for supporting the research work.
References
- Meuser H. Contaminated urban soils. Netherlands: Springer; 2010. (Environmental Pollution; vol. 18).10.1007/978-90-481-9328-8
- Karimi R, Ayoubi S, Jalalian A, et al. Relationships between magnetic susceptibility and heavy metals in urban topsoils in the arid region of Isfahan, central Iran. J Appl Geophys. 2011;74:1–7.10.1016/j.jappgeo.2011.02.009
- Dankoub Z, Ayoubi S, Khademi H, et al. Spatial distribution of magnetic properties and selected heavy metals in calcareous soils as affected by land use in the Isfahan Region, Central Iran. Pedosphere. 2012;22:33–47.10.1016/S1002-0160(11)60189-6
- Shen F, Liao R, Ali A, et al. Spatial distribution and risk assessment of heavy metals in soil near a Pb/Zn smelter in Feng county, China. Ecotox Environ Safe. 2017;139:254–262.10.1016/j.ecoenv.2017.01.044
- Pandey B, Suthar S, Singh V. Accumulation and health risk of heavy metals in sugarcane irrigated with industrial effluent in some rural areas of Uttarakhand, India. Process Saf Environ Prot. 2016;102:655–666.10.1016/j.psep.2016.05.024
- Awomeso JA, Ufoegbune GC, Oluwasanya GO, et al. Impact of industrial effluents on water, soils and plants in the Alakia industrial area of Ibadan, South West Nigeria. Toxicol Environ Chem. 2009;91:5–15.10.1080/02772240802074975
- Barman SC, Sahu RK, Bhargava SK, et al. Distribution of heavy metals in wheat, mustard and weed grains irrigated with industrial effluents. Bull Environ Contam Toxicol. 2000;64:489–496.10.1007/s001280000030
- Farahat E, Linderholm HW. The effect of long-term wastewater irrigation on accumulation and transfer of heavy metals in Cupressus sempervirens leaves and adjacent soils. Sci Total Environ. 2015;512–513:1–7.10.1016/j.scitotenv.2015.01.032
- Ayoubi S, Amiri S, Tajik S. Lithogenic and anthropogenic impacts on soil surface magnetic susceptibility in an arid region of Central Iran. Arch Agron Soil Sci. 2014;60:1467–1483.10.1080/03650340.2014.893574
- Zhou J, Ma D, Pan J, et al. Application of multivariate statistical approach to identify heavy metal sources in sediment and waters: a case study in Yangzhong, China. Environ Geol. 2008;54:373–380.10.1007/s00254-007-0824-5
- Almasoud FI, Usman ARA, Al-Farraj AS. Heavy metals in the soils of the Arabian Gulf coast affected by industrial activities: analysis and assessment using enrichment factor and multivariate analysis. Arab J Geosci. 2015;8:1691–1703.10.1007/s12517-014-1298-x
- Wu C, Zhang L. Heavy metal concentrations and their possible sources in paddy soils of a modern agricultural zone, south eastern China. Environ Earth Sci. 2010;60:45–56.10.1007/s12665-009-0168-4
- Krami LK, Amiri F, Sefiyanian A, et al. Spatial patterns of heavy metals in soil under different geological structures and land uses for assessing metal enrichments. Environ Monit Assess. 2013;185:9871–7888.10.1007/s10661-013-3298-9
- Hu Y, Liu X, Bai J, et al. Assessing heavy metal pollution in the surface soils of a region that had undergone three decades of intense industrialization and urbanization. Environ Sci Pollut Res. 2013;20:6150–6159.
- Taghipour M, Ayoubi S, Khademi H. Contribution of lithologic and anthropogenic factors to surface soil heavy metals in Western Iran using multivariate geostatistical analyses. Soil Sediment contam. 2011;20:921–937.10.1080/15320383.2011.620045
- Naimi S, Ayoubi S. Vertical and horizontal distribution of magnetic susceptibility and metal contents in an industrial district of central Iran. J Appl Geophys. 2013;96:55–66.10.1016/j.jappgeo.2013.06.012
- Al-Farraj AS, Al-Sewailem M, Aly A, et al. Assessment and heavy metal behaviors of industrial waste water: a case study of Riyadh city, Saudi Arabia. Proceedings of the International Academy of Ecology and Environmental Sciences. 2013;3:266–277.
- Gee GW, Bauder JW. Particle-size analysis. In: Klute A, editor. Methods of soil analysis: physical and mineralogical methods. Part 1, 2nd ed. Madison (WI): Soil Science Society of America and American Society of Agronomy; 1994. p. 383–409.
- Hossner LH. Dissolution for total elemental analysis. In: Sparks DL, Page AL, Helmke PA, Loeppert RH, editors. Methods of soil analysis: chemical methods. Part 3, 3rd ed. Madison (WI): Soil Science Society of America and American Society of Agronomy; 1994. p. 49–64.
- Kashem MA, Singh BR. Solid phase speciation of Cd, Ni and Zn in some contaminated and non-contaminated tropical soils. In: Iskandar IK, Krikham MB, editors. Trace elements in soil: bioavailability, flux and transfer. Boca Raton: Lewis Publishers, CRS Press; 2001. p. 213–227.
- Tessier A, Campbell PGC, Bisson M. Sequential extraction procedure for the speciation of particulate trace metals. Anal Chem. 1979;51:844–851.10.1021/ac50043a017
- Sutherland RA. Bed sediment associated trace metals in an urban stream, Oahu, Hawaii. Environ Geol. 2000;39:611–627.10.1007/s002540050473
- Taylor SR. Abundances of chemicals elements in the continental crust: a new table. Geochimica et Cosmochimica Acta. 1964;28:1273–1285.10.1016/0016-7037(64)90129-2
- Hernandez L, Probst A, Probst JL, et al. Heavy metal distribution in some French forest soils: evidence for atmosphere contamination. Sci Total Environ. 2003;312:195–210.10.1016/S0048-9697(03)00223-7
- StatSoft Inc. Statistica for windows (Computer Program Manual). Tulsa (OK): StatSoft,Inc.; 1992.
- Beesley L, Moreno-Jimenez E, Clemente R, et al. Mobility of arsenic, cadmium and zinc in a multi-element contaminated soil profile assessed by in situ soil pore water sampling, column leaching and sequential extraction. Environ Pollut. 2010;158:155–160.10.1016/j.envpol.2009.07.021
- Lindsay W. Chemical equilibrai in soils. 1st ed. New York (NY): Wiley-interscience publication; 1979.
- VROM. The new Dutch list. Soil quality standards, Netherlands ministry of housing, spatial planning and environment. The Hague: Department of Soil Protection; 2001.
- Turekian KK, Wedepohl KH. Distribution of the elements in some major units of the earth’s crust. Geol Soc Am Bull. 1961;72:175–192.10.1130/0016-7606(1961)72[175:DOTEIS]2.0.CO;2
- Singh KP, Mohan D, Singh VK, et al. Studies on distribution and fractionation of heavy metals in Gomti river sediments – a tributary of the Ganges, India. J Hydrol. 2005;312:14–27.10.1016/j.jhydrol.2005.01.021
- Simón M, Diez M, González V, et al. Use of liming in the remediation of soils polluted by sulphide oxidation: a leaching-column study. J Hazard Mater. 2010;180:241–246.10.1016/j.jhazmat.2010.04.020
- Modak DP, Singh AP, Chandra H, et al. Mobile and bound forms of trace metals in sediments of the lower ganges. Water Res. 1992;26:1541–1548.10.1016/0043-1354(92)90075-F
- Malik N, Biswas AK, Raju CB. Geochemical distribution and fractionation of heavy metals in sediments of a freshwater reservoir of India. Chem Spec Bioavailab. 2012;24:113–123.10.3184/095422912X13335538789245
- Usman ARA, Ghallab A. Heavy-metal fractionation and distribution in soil profiles short-term-irrigated with sewage wastewater. Chem Ecol. 2006;22:267–278.10.1080/02757540600812859
- Karathanasis AD, Pils JRV. Solid-phase chemical fractionation of selected trace metals in some Northern Kentucky soils. Soil Sediment Contam. 2005;14:293–308.
- Ma LQ, Rao GN. Chemical fractionation of cadmium, copper, nickel, and zinc in contaminated soils. J Environ Qual. 1997;26:259–264.10.2134/jeq1997.00472425002600010036x
- Tyler LD, McBride MB. Mobility and extractability of cadmium, copper, nickle, and zinc in organic and mineral soil columns. Soil Sci. 1982;134:198.10.1097/00010694-198209000-00009
- Ashworth DJ, Alloway BJ. Influence of dissolved organic matter on the solubility of heavy metals in sewage‐sludge‐amended soils. Commun Soil Sci Plant Anal. 2008;39:538–550.10.1080/00103620701826787
- Kotoky P, Bora PJ, Baruah NK, et al. Chemical fractionation of heavy metals in soils around oil installations, Assam. Chem Spec Bioavailab. 2003;15:115–126.10.3184/095422903782775181
- He Q, Ren Y, Mohamed I, et al. Assessment of trace and heavy metal distribution by four sequential extraction procedures in a contaminated soil. Soil Water Res. 2013;8:71–76.
- Han FX, Kingery WL, Selmi HM, et al. Accumulation of heavy metals in a long-term poultry waste-amended soil. Soil Sci. 2000;165:260–268.10.1097/00010694-200003000-00008
- Rieuwerts JS, Thornton I, Farago ME, et al. Factors influencing metal bioavailability in soils: preliminary investigations for the development of a critical loads approach for metals. Chem Spec Bioavailab. 1998;10:61–75.10.3184/095422998782775835
- Ogundiran MB, Osibanjo O. Mobility and speciation of heavy metals in soils impacted by hazardous waste. Chem Spec Bioavailab. 2009;21:59–69.10.3184/095422909X449481
- Chen T, Liu X, Zhu M, et al. Identification of trace element sources and associated risk assessment in vegetable soils of the urbane rural transitional area of Hangzhou, China. Environ Pollut. 2008;151:67–78.10.1016/j.envpol.2007.03.004