Abstract
Hickory and peanut hydrochars were chemically activated with KOH and H3PO4 and tested for their ability to remove methylene blue and lead from aqueous solutions. The physicochemical characteristics of the activated hydrochars determined were surface area, pore volume, and elemental composition. Kinetics and isotherm studies were then conducted on methylene blue adsorption. Compared to their nonactivated counterparts, the chemically activated hydrochars had higher surface areas and more functional groups. Activated hydrochars also had greater methylene blue and lead adsorption rates, which can be attributed to the improved physicochemical characteristics. H3PO4 activated hydrochars removed more contaminants than the corresponding KOH ones.
1. Introduction
There are needs for carbon based adsorbents, particularly activated carbon (AC), for the removal of aqueous contaminants. Water bodies are often contaminated due to discharges by industrial activity, and it is a major concern throughout the world due to the ecological and health risks it poses [Citation1]. AC may be produced from any organic feedstock, but it is usually commercially produced from coconut shells and coal [Citation2–4]. Since there is an abundance of agricultural and industrial wastes produced throughout the world, many studies have explored the processing of these waste materials into AC as a low-cost alternative to commercial AC [Citation3–6]. Some of the feedstocks tested include waste tires, coir pith, bagasse, olive mill waste, corn cobs, and apricot stones [Citation7–12]. These feedstocks have been found to produce ACs that are highly effective for the removal of dyes and heavy metals [Citation7–12].
In order to obtain the best properties for adsorption, the feedstock used must have a low ash content, as the presence of inorganic elements weakens the structure of the AC, causing pore collapse in the carbon matrix [Citation13]. When coals are used as feedstocks for AC, it has been found that the higher the rank of the coal, the more resistant it is to activation, whether physical or chemical [Citation14]. Lignite would thus be a better choice for AC production than anthracite. However, lignite has drawbacks including a high ash content and a high sulfur content, which is also considered a water contaminant [Citation15]. Hydrochar, therefore, could be considered as an alternative to lignite as feedstock to AC production. Its degree of coalification is similar to lignite, but it has a low ash and sulfur content. Hydrochar is also a poor adsorbent when used as is due to its low surface area and high volatiles content [Citation16,17], and activation would help improve its properties.
Previous studies have examined the sorptive properties of hydrochar for various contaminants [Citation18]. While several studies have examined the sorptive properties of hydrochar for various contaminants, only few have examined the effects of chemical modification on hydrochar’s physicochemical properties and its ability to adsorb contaminants [Citation18–23]. In the literature, the methods of chemical activation of carbon materials such as lignite have been well documented, which should be applicable to hydrochars [Citation24,25]. To the best of the authors’ knowledge, however, none of the previous studies has evaluate whether hydrochar can be used as an alternative feedstock for AC through chemical activation. Furthermore, the physicochemical property and sorptive ability of the chemically activated hydrochar is unknown too. It is thus not only novel but also crucial to study chemically activation of hydrochars and their potential applications.
The overarching objective of this study were to investigate the effects of H3PO4 and KOH chemical activation on the physicochemical characteristics of hickory and peanut hull hydrochars. H3PO4 and KOH were chosen because they have been well-established as highly effective activating agents and also so that the effects of both an acid and a base on the physicochemical characteristics of hydrochar can be investigated. The four AHCs were tested for their ability to adsorb methylene blue and lead, which are representative organic and metal contaminants, from aqueous solutions. Finally, the relationships between the activated hydrochar (AHC) physicochemical properties and their sorption characteristics were examined in order to identify predominant sorption mechanisms.
2. Materials and methods
2.1. Hydrochar production and activation
Hickory and peanut hull hydrochars were produced using the same procedure previously described [Citation17]. Briefly, feedstocks were milled to particle sizes of 0.5–1 mm and added to a pressurizable lidded stainless-steel pot to a height of about one inch from the top (50 g of hickory and 55 g of peanut hull). Deionized (DI) water was added to the same level (290 and 313 mL of water, respectively). The pots were then sealed and heated on a hotplate to 200 °C for six hours, which were the HTC conditions that produced hydrochar with the highest yield and surface area [Citation17]. The resulting hydrochars were rinsed for one hour by submersion in tap water and ten minutes in DI water to remove water soluble volatile matter, and oven dried for 24 h at 70 °C. For activation, the hydrochar was mixed in a 1:1 w/w ratio with either KOH solution or 85% H3PO4 solution. The mixture was stirred on top of a hotplate for 2 h at 85 °C, and the resulting slurry was oven dried for 24 h at 100 °C. The dried slurry was then activated in a tube furnace for 1 h at 600 °C under N2 gas flowing at a rate of 150 mL min−1. The AHCs were then allowed to cool to room temperature, and were washed with DI water in order to remove any residual chemicals. They were rinsed repeatedly with DI water until the pH of the AHC-water mixture stabilized.
2.2. Activated hydrochar characterization
A CHN Elemental Analyzer (Carlo-Erba NA-1500) was used to determine the total carbon, hydrogen, and nitrogen contents of the activated hydrochars. Samples were analyzed in duplicate and the averages are reported here. The surface areas (SA) of the samples were measured by N2 sorptometry on a Quantachrome Autosorb I at 77 K and using the Brunauer-Emmett-Teller (BET) method in the 0.01 to 0.3 relative pressure range of the N2 adsorption isotherm. Pore volumes (PV) were calculated from the desorption branch N2 isotherms using Barrett-Joyner-Halenda (BJH) theory. Samples were de-gassed under vacuum at least 24 h at 180 °C prior to analysis.
2.3. Batch sorption experiments
Batch aqueous contaminant sorption experiments were carried out in triplicate. All chemicals used were analytical grade and obtained from Fisher Scientific Co. and solutions were prepared using DI water (Nanopure water, Barnstead). Mixtures of 30 mL of each solution and 0.1 g of AHC in 68 mL digestion vessels were agitated on a mechanical shaker at 40 rpm. For sorption kinetics experiments, the AHCs were tested at 500 ppm for lead and 700 ppm for methylene blue, and samples were withdrawn at time intervals ranging from 0.5 to 24 h. For sorption isotherm experiments, the AHCs were mixed with solutions ranging from 10 to 1000 ppm for methylene blue and 10–700 ppm for lead and then shaken for 24 h. The solutions were then immediately filtered through 0.45 um filter paper (Whatman). Aqueous lead concentrations were analyzed using inductively coupled plasma spectroscopy (ICP-AES, Perkin Elmer Optima 2100 DV), and methylene blue was measured with a UV spectrometer (Thermo Scientific EVO 60) at a wavelength of 665 nm. The sorption rates of the AHCs were calculated as the difference between starting and final sorbate solution concentrations relative to the amount of sorbent (mg g−1). All the experiments were conducted in duplicate and the mean values are reported. Additional analyses were conducted whenever two measurements showed a difference larger than 5%.
2.4. Mathematical models
Pseudo-first-order, pseudo-second-order, and Elovich models were used to simulate the sorption kinetics data. Governing equations for these models can be written as [Citation1]:
First-order: ,
Second-order: ,
Elovich: ,
where qt and qe are the amount of sorbate removed at time t and at equilibrium, respectively (mg g−1); k1 and k2 are the first-order and second-order sorption rate constants (h−1), respectively; α is the initial sorption rate (mg g−1); and β is the desorption constant (g mg−1). The first- and second-order models are semi-empirical equations, while the Elovich model is an empirical fitting equation.
The Langmuir and Freundlich models were used to simulate the sorption isotherms. Their governing equations can be written as [Citation1]:
Langmuir: ,
Freundlich: ,
where K and Kf are the Langmuir bonding term related to interaction energies (L mg−1) and the Freundlich affinity coefficient (mg(1−n) Ln g−1), respectively; Smax is the Langmuir maximum capacity (mg kg−1); Ce is the equilibrium solution concentration (mg L−1) of the sorbate; and n is the Freundlich linearity constant. The Langmuir model assumes monolayer adsorption onto a homogeneous surface with no interactions between the adsorbed molecules. The Freundlich model is an empirical equation commonly used for heterogeneous surfaces.
3. Results and discussion
3.1. Physicochemical characteristics
Chemical activation greatly increased the surface area of the hydrochars, especially with H3PO4. Hickory and peanut hull hydrochars activated with H3PO4 had surface areas of 1436 and 1091 m2 g−1, respectively. While hickory had a surface area almost 50% greater than that of peanut hull after undergoing H3PO4 activation, peanut hull had a surface area that was twice as large as hickory when KOH activation was used (571 vs. 222 m2 g−1). This suggested that hickory hydrochar might be a better feedstock for the production of ACs with relatively high surface area. In the hickory AHCs, BJH-PV decreased after activation despite the increase in surface area, although the BJH-PV of peanut hull AHCs increased along with an increase in surface area (Table ). The BJH-PVs of all of the chemically AHCs are lower than those of the physically AHCs (Table ). Previous studies have shown that chemical activated AC may have small pore volume due to the lower burn-off rate compared to physical activation [Citation26].
Table 1. Bulk properties of activated hickory and peanut hull hydrochars.
Compared to physically AHCs, the chemically AHCs have a lower C content. In addition, the chemically AHCs also had slightly lower C content than the hydrochar they were synthesized from (Table ). The chemical activation process produces oxygenated functional groups on the surface of the hydrochar as a result of the chemicals that are added, whereas in physical activation, less functional groups are formed and the proportion of C in the AHC increases [Citation19]. H3PO4 activation produced hickory hydrochar with a higher surface area than did physical activation, and although it increased surface area of the peanut hull hydrochar, it did not increase as much as it did with physical activation. This indicated that feedstock type was also an important factor in the differences between physicochemical characteristics of the AHCs.
Final yields of chemically AHCs ranged from 40 to 54% of the original weight of hydrochar used. These yields are comparable to those obtained with physical activation at 600–800 °C, but were higher than physically AHCs obtained at 900 °C. However, for both hickory and peanut hull, the surface areas of the H3PO4 AHCs were more similar to those of the 900 °C physically AHCs. KOH AHC from peanut hulls had a surface area that was in between those that were physically activated at 600–800 and 900 °C, while KOH AHC from hickory had a surface area than those at all physical activation temperatures. The comparability in morphology between the chemically AHCs in this work and the 900 °C physically AHCs in a previous study indicates that activation of hydrochar might be more efficient with chemicals [Citation22]. This is because the addition of chemicals inhibits the formation of tars during activation. During physical activation, tars are formed and volatilized, increasing the amount of char that is burned off [Citation27].
3.2. Batch sorption experiments
An initial assessment of the chemically AHCs were conducted that showed the chemically activated hydrochars were better able to sorb lead than raw and physically activated hydrochar, with sorption capacities ranging from 95 to 162.3 mg Pb g−1, compared to 9.2–46.7 mg Pb g−1 (Figure ). The hickory AHCs had higher sorption rates than the peanut hull AHCs, and for both feedstocks, KOH created hydrochars with higher sorption rates than H3PO4. For methylene blue, chemically activated hydrochars had higher sorption rates than their raw counterparts, but were lower than that of their physically activated counterparts. The only exception to this is H3PO4 activated hickory hydrochar, which has a sorption capacity of 208.6 mg g−1 compared to 141.3 mg Pb g−1 for the physically activated hickory hydrochar. This is probably due to the much higher surface area of the H3PO4 hickory than those of the other AHCs.
Figure 1. Comparison of chemically AHC with physically AHC and raw HC for the sorption of contaminants. (A) methylene blue and (B) lead.
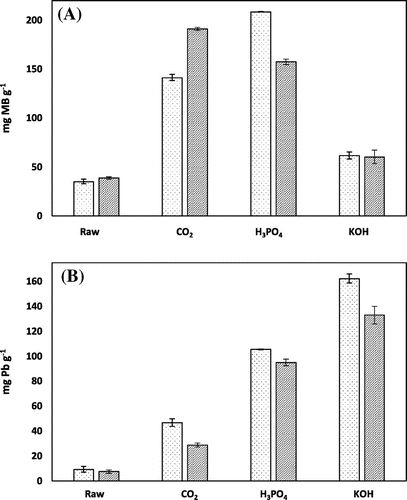
Kinetics experiments showed that all of the chemically AHCs were able to adsorb MB within 10 h and lead within 5 h (Figures and ). These kinetic rates are comparable to that of MB and lead sorption onto pristine and engineered biochars as reported in previous studies [Citation28–30]. As shown in Tables and , the Elovich model fits the data for lead sorption by H3PO4 peanut hull AHC and MB sorption by all of the AHCs (except H3PO4 hickory AHC), indicating that sorption in these instances involved heterogeneous surface adsorption processes [Citation31]. The second-order model fit the remaining data the best, suggesting sorption of MB and lead onto these AHCs followed the binuclear surface adsorption mechanism [Citation19].
Figure 2. Methylene blue adsorption kinetics: (A) H3PO4 hickory, (B) KOH hickory, (C) H3PO4 peanut, and (D) KOH peanut.
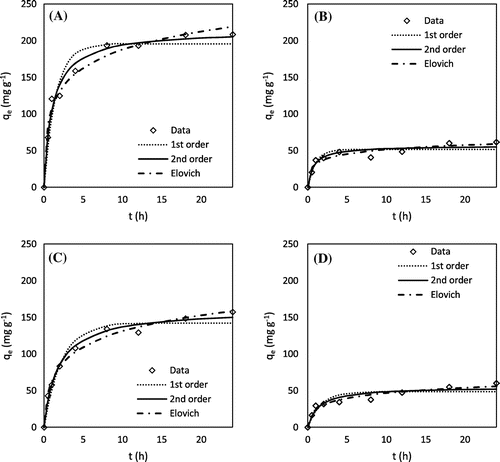
Figure 3. Lead adsorption kinetics: (A) H3PO4 hickory, (B) KOH hickory, (C) H3PO4 peanut, and (D) KOH peanut.
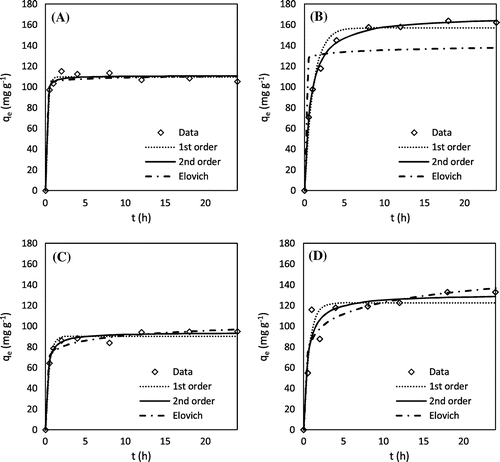
Table 2. Best-fit model parameters of lead sorption on AHCs.
Table 3. Best-fit model parameters of methylene blue sorption on AHCs.
Isotherm experiments showed that all of the chemically AHCs showed strong sorption of MB and lead from aqueous solutions (Figures and ). For MB adsorption isotherms, the Langmuir model fit the H3PO4 AHCs better than the Freundlich model, while Freundlich was a better fit for the KOH AHCs than Langmuir (Table ). For the adsorption of lead, Langmuir was a better fit than Freundlich for all of the AHCs, as indicated by their higher R2 values (Table ). For the adsorption isotherms followed the Langmuir model (i.e. lead on all AHCs and MB on the H3PO4 AHCs), the sorption processes should be mainly controlled by monolayer adsorption on a homogeneous surface with no interaction between the adsorbed molecules; while the ones followed the Freundlich model (i.e. MB on the KOH AHCs), the process might be controlled by chemisorption onto heterogeneous surfaces [Citation32].
3.3. Sorption mechanisms
Different mechanisms of sorption were likely at play with lead and MB, explaining why their rates of sorption are different according to the type of chemical used in activation. MB is a large organic molecule, while lead is smaller. KOH activation is known to produce highly microporous ACs, while H3PO4 has a higher proportion of meso- and macro- pores [Citation33]. The MB is therefore unable to access many of the sorption sites on the KOH AHCs [Citation33,34]. Likewise, H3PO4 also produces more micropores than physical activation; as a result, physically AHCs have a higher capacity for MB than the chemically AHCs [Citation26]. The exception to this is H3PO4 hickory, since the higher surface area compensates for the lower proportion of micropores, and more meso- and macro- porous sorption sites are provided. Although the KOH AHCs had surface areas that were five times lower than that of the H3PO4 AHCs, they were able to adsorb more lead. This indicates that in addition to the number of available sorption sites, surface oxygenated functional groups play a large role in the removal of lead from solution, as KOH tends to produce more functional groups than H3PO4. KOH activation of maize stalks have been found to produce ACs that are highly effective at removing Pb2+ from solution, and this was attributed to the micropores and functional groups that developed on the maize stalks after activation [Citation35]. The surface functional groups remove lead through surface complexation [Citation36]. Figure shows the relationship between surface area and the amount of contaminant sorbed by raw and activated hydrochars. There is a higher correlation between sorption and surface area with MB (R2 = 0.91) than there is with Pb (R2 = 0.06), confirming that surface area predominantly governs MB sorption and surface chemistry of the sorbent more important in Pb sorption.
4. Conclusions
Chemical activation of hydrochar resulted in an increase in surface area, which led to greater sorption of methylene blue and lead compared to the pristine hydrochar. In general, the higher the temperature and the longer the activation time, the greater the AHC ability to adsorb the two contaminants from aqueous solution. This can be linked to increases in surface area with activation time and temperature and suggests that surface adsorption was the governing mechanism. The AHCs produced at 900 °C sorbed methylene blue and lead at rates comparable to those of commercial activated carbons. Although these sorption rates are at the lower end of the range, further exploration of other hydrochar feedstock materials and activation conditions would likely yield AHCs with even higher sorption efficiencies. Because hydrothermal carbonization is more energy efficient than dry pyrolysis, the use of AHCs may prove to be a more cost-effective and environmentally friendly alternative to traditional activated carbons for contaminant remediation and water treatment applications. For instance, AHCs may be used in soil remediation to immobilize toxic chemicals including heavy metals and organics to reduce their mobility and bioavailability.
Funding
This research was partially supported by the NSF (Division of Chemical, Bioengineering, Environmental, and Transport Systems) [grant number CBET-1054405].
Disclosure statement
No potential conflict of interest was reported by the authors.
References
- Inyang M, Gao B, Yao Y, et al. Removal of heavy metals from aqueous solution by biochars derived from anaerobically digested biomass. Bioresour Technol. 2012;110:50–56.10.1016/j.biortech.2012.01.072
- Girgis BS, Yunis SS, Soliman AM. Characteristics of activated carbon from peanut hulls in relation to conditions of preparation. Mater Lett. 2002;57:164–172.10.1016/S0167-577X(02)00724-3
- Gottipati R, Mishra S. Preparation of microporous activated carbon from Aegle Marmelos fruit shell and its application in removal of chromium(VI) from aqueous phase. J Ind Eng Chem. 2016;36:355–363.10.1016/j.jiec.2016.03.005
- Habila MA, ALOthman ZA, Al-Tamrah SA, et al. Activated carbon from waste as an efficient adsorbent for malathion for detection and removal purposes. J Ind Eng Chem. 2015;32:336–344.10.1016/j.jiec.2015.09.009
- Hesas RH, Arami-Niya A, Daud WMAW, et al. Microwave-assisted production of activated carbons from oil palm shell in the presence of CO2 or N-2 for CO2 adsorption. J Ind Eng Chem. 2015;24:196–205.10.1016/j.jiec.2014.09.029
- Heo YJ, Park SJ. Synthesis of activated carbon derived from rice husks for improving hydrogen storage capacity. J Ind Eng Chem. 2015;31:330–334.10.1016/j.jiec.2015.07.006
- Betancur M, Martínez JD, Murillo R. Production of activated carbon by waste tire thermochemical degradation with CO2. J Hazard Mater. 2009;168:882–887.10.1016/j.jhazmat.2009.02.167
- Baçaoui A, Yaacoubi A, Dahbi A, et al. Optimization of conditions for the preparation of activated carbons from olive-waste cakes. Carbon. 2001;39:425–432.10.1016/S0008-6223(00)00135-4
- Chang CF, Chang CY, Tsai WT. Effects of burn-off and activation temperature on preparation of activated carbon from corn cob agrowaste by CO2 and steam. J Colloid Interface Sci. 2000;232:45–49.10.1006/jcis.2000.7171
- Kobya M, Demirbas E, Senturk E, et al. Adsorption of heavy metal ions from aqueous solutions by activated carbon prepared from apricot stone. Biores Technol. 2005;96:1518–1521.10.1016/j.biortech.2004.12.005
- Namasivayam C, Kavitha D. Removal of Congo Red from water by adsorption onto activated carbon prepared from coir pith, an agricultural solid waste. Dyes Pigm. 2002;54:47–58.10.1016/S0143-7208(02)00025-6
- Valix M, Cheung WH, McKay G. Preparation of activated carbon using low temperature carbonisation and physical activation of high ash raw bagasse for acid dye adsorption. Chemosphere. 2004;56:493–501.10.1016/j.chemosphere.2004.04.004
- Ahmadpour A, Do DD. The preparation of active carbons from coal by chemical and physical activation. Carbon. 1996;34:471–479.10.1016/0008-6223(95)00204-9
- Lillo-Ródenas MA, Juan-Juan J, Cazorla-Amorós D, et al. About reactions occurring during chemical activation with hydroxides. Carbon. 2004;42:1371–1375.10.1016/j.carbon.2004.01.008
- Jia X, Wang QH, Cen KF, et al. Sulfur transformation during the pyrolysis of coal mixed with coal ash in a fixed bed reactor. Fuel. 2016;177:260–267.10.1016/j.fuel.2016.03.013
- Sun YN, Gao B, Yao Y, et al. Effects of feedstock type, production method, and pyrolysis temperature on biochar and hydrochar properties. Chem Eng J. 2014;240:574–578.10.1016/j.cej.2013.10.081
- Fang J, Gao B, Chen J, et al. Hydrochars derived from plant biomass under various conditions: Characterization and potential applications and impacts. Chem Eng J. 2015;267:253–259.10.1016/j.cej.2015.01.026
- Fang J, Zhan L, Ok YS, et al. Minireview of potential applications of hydrochar derived from hydrothermal carbonization of biomass. J Ind Eng Chem. 2017. doi:10.1016/j.jiec.2017.08.026.
- Xue Y, Gao B, Yao Y, et al. Hydrogen peroxide modification enhances the ability of biochar (hydrochar) produced from hydrothermal carbonization of peanut hull to remove aqueous heavy metals: Batch and column tests. Chem Eng J. 2012;200-202:673–680.10.1016/j.cej.2012.06.116
- Fernandez ME, Ledesma B, Román S, et al. Development and characterization of activated hydrochars from orange peels as potential adsorbents for emerging organic contaminants. Biores Technol. 2015;183:221–228.10.1016/j.biortech.2015.02.035
- Zhang M, Gao B, Fang J, et al. Self-assembly of needle-like layered double hydroxide (LDH) nanocrystals on hydrochar: characterization and phosphate removal ability. RSC Adv. 2014;4:28171–28175.10.1039/c4ra02332c
- Fang J, Gao B, Zimmerman AR, et al. Physically (CO2) activated hydrochars from hickory and peanut hull: preparation, characterization, and sorption of methylene blue, lead, copper, and cadmium. RSC Adv. 2016;6:24906–24911.10.1039/C6RA01644H
- Jain A, Balasubramanian R, Srinivasan MP. Hydrothermal conversion of biomass waste to activated carbon with high porosity: A review. Chem Eng J. 2016;283:789–805.10.1016/j.cej.2015.08.014
- Nowicki P, Kuszynska I, Przepiorski J, et al. Effect of chemical activation method on the properties of activated carbons made of lignite. Przem Chem. 2012;91:1846–1850.
- Pasadakis N, Romanos G, Perdikatsis V, et al. The production of activated carbons using greek lignites by physical and chemical activation methods: a comparative study. Energ Source Part A. 2011;33:713–723.10.1080/15567030903261808
- Salas-Enríquez BG, Torres-Huerta AM, Conde-Barajas E, et al. Activated carbon production from the Guadua amplexifolia using a combination of physical and chemical activation. J Therm Anal Calorim. 2016;124:1383–1398.10.1007/s10973-016-5238-8
- Kandiyoti R, Lazaridis JI, Dyrvold B, et al. Pyrolysis of a ZnCl2-impregnated coal in an inert atmosphere. Fuel. 1984;63:1583–1587.10.1016/0016-2361(84)90231-X
- Inyang MI, Gao B, Yao Y, et al. A review of biochar as a low-cost adsorbent for aqueous heavy metal removal. Critical Reviews in Environmental Science and Technology. 2016;46:406–433.10.1080/10643389.2015.1096880
- Wang SS, Gao B, Li YC, et al. Manganese oxide-modified biochars: Preparation, characterization, and sorption of arsenate and lead. Biores Technol. 2015;181:13–17.10.1016/j.biortech.2015.01.044
- Yao Y, Gao B, Fang J, et al. Characterization and environmental applications of clay-biochar composites. Chem Eng J. 2014;242:136–143.10.1016/j.cej.2013.12.062
- Zhang M, Gao B. Removal of arsenic, methylene blue, and phosphate by biochar/AlOOH nanocomposite. Chem Eng J. 2013;226:286–292.10.1016/j.cej.2013.04.077
- Yao Y, Gao B, Inyang M, et al. Removal of phosphate from aqueous solution by biochar derived from anaerobically digested sugar beet tailings. J Hazard Mater. 2011;190:501–507.10.1016/j.jhazmat.2011.03.083
- Hui TS, Zaini MAA. Potassium hydroxide activation of activated carbon: a commentary. Carbon Lett. 2015;16:275–280.10.5714/CL.2015.16.4.275
- Altenor S, Carene B, Emmanuel E, et al. Adsorption studies of methylene blue and phenol onto vetiver roots activated carbon prepared by chemical activation. J Hazard Mater. 2009;165:1029–1039.10.1016/j.jhazmat.2008.10.133
- El-Hendawy ANA. An insight into the KOH activation mechanism through the production of microporous activated carbon for the removal of Pb2+ cations. Appl Surf Sci. 2009;255:3723–3730.10.1016/j.apsusc.2008.10.034
- Ding W, Dong X, Ime IM, et al. Pyrolytic temperatures impact lead sorption mechanisms by bagasse biochars. Chemosphere. 2014;105:68–74.10.1016/j.chemosphere.2013.12.042