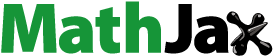
ABSTRACT
A combined technology between Iron-carbon micro-electrolysis (ICME) and H2O2 using scrap iron and granular active carbon was extensively investigated and optimized (three main independent parameters chosen) for chemical oxygen demand (COD) removal of landfill leachate reverse osmosis (RO) concentration by batch experiments. A regression quadratic equation was developed to model the outcome using central composite design (CCD) coupled with response surface methodology. A global optimization method–the convex optimization was applied to determine the optimal parameters. Under optimal parameters determined as C/Fe (Surface area) of 717143.8, H2O2 concentration of 1687.6 mg/L and pH of 3.8, the corresponding COD removal efficiency was up to 86.9%. Consequently, the COD in the effluent was less than 300 mg/L. The treated water can meet the level B standards of Wastewater Quality Standards for Discharge to Municipal Sewers (CJ 343—2010).
1. Introduction
Landfill leachates are referred as highly contaminated wastewaters principally generated by rainwater percolating through the covering and inner layers in municipal landfill during the decomposition process of the solid wastes [Citation1]. Leachate is typically characterized by high ammonium concentration, low biodegradability, heavy metals, high fraction of recalcitrant and large organic molecules, such as chlorinated organic compounds, benzene, toluene, and xylenes etc [Citation2]. Various treatment options for landfill leachate, including physicochemical process [Citation3–Citation5], and biological treatment have been widely reported in recent decades. Biological processes are commonly used to treat landfill leachate due to its low cost. However, in many cases, a single biological process has not proven efficient to eliminate the large amount of non-biodegradable organic substances to the required discharge standard. Since the leachate discharge standards were improved in China after the publication of <Standards for pollution control on the landfill site of municipal solid waste> (GB16889–2008) in 2008. Reverse osmosis (RO) membrane have been more widely applied because RO retain both organic and inorganic contaminants at a high rejection rate [Citation3,Citation4]. In general, an integrated membrane filtration technology, including membrane bioreactor (MBR), nanofiltration (NF), and reverse osmosis (RO), as a main post treatment step in leachate treatment has been more widely employed. However, the generation of large volumes of RO concentrates, which is toxic and bio-refractory, is a major problem with these processes [Citation6]. Up to 1/4–1/8 of the volume of the concentrated wastewater is rejected by RO containing high fraction of refractory organics with high molecular weight [Citation4]. Though the application of MBR unit and NF unit as pretreatment can minimize the volumes of RO concentrates, it is difficult to completely solve the problem of RO concentrate generated. Therefore, in order to minimize the potential environmental risk, proper treatment of landfill leachate RO concentrate is helpful to avoid the secondary pollution of the above processes.
Practically, in order to facilitate the degradation of various initially refractory (low BOD5/COD ratio) and/or toxic organic compounds to common microorganisms to readily biodegradable and less toxic intermediates, Advanced Oxidation Processes (AOPs) were proved to be an attractive and efficient option in the last couple of decades [Citation7]. Even though these processes presented excellent pollutants removal capabilities,the drawbacks of existing AOPs system, such as Fenton, ozone, ultraviolent irradiation, photocatalytical processes and etc, are high level of energy consumption and extra reagents cost, which unavoidably limit these potential engineering application. Iron-carbon micro-electrolysis (ICME) technology provides the advantages of no extra energy, the low cost, easy operation, wide applicability and long service life. Recently, ICME has been successfully used in treatment of various industrial wastewater, such as pharmaceutical wastewater [Citation8], dye-stuff [Citation9], chemical wastewater [Citation10] and etc. In the Fe-Cmicro-electrolysis system, iron scrap and globular activated carbon are commonly immerged into the wastewater and acted as the electrode materials. Numerous microscopic galvanic cells in ICME system were spontaneously formed between the particles of iron (anode) and carbon (cathode), which could generate a large number of hydroxyl (OH−), atomic hydrogen ([H]) and Fe (II) with high reactivity. It is worth noting that this process is simultaneously accompanied by the release of substantial electrons, thereby generating a series of physicochemical reaction such as electro-flocculation, electrostatic reaction and oxidation-reduction reaction for decomposing organic compounds [Citation11]. Thus, ICME can exhibit the capability of high degradation efficiency of refractory compounds and improvement of wastewater biodegradability. Additionally, most studies reported that an external addition of hydrogen peroxide in ICME could improve the removal efficiency of organic matters because Fe2+ dissolved in the solution from iron anode will further react with hydrogen peroxide [Citation12]. Therefore, deep study in detail of the degradation property of RO concentrate by ICME coupled with hydrogen peroxide was great interest.
The much-aimed this study was to evaluate the performance of ICME coupled with hydrogen peroxide process in treatment of RO concentrate in terms of COD abatement efficiency and treatment optimization. Firstly, these three independent variables: C/Fe ratio (Surface area) of ICME granule chosen, the concentration of H2O2 (mg/L) and pH were investigated for COD reduction of RO concentrate in a series of batch experiments based on a central composite design (CCD). Secondly, the obtained results were further modeled to a second-order polynomial model by response surface methodology (RSM). RSM is a powerful statistical tool for designing experiments, building and analyzing models, evaluating the simultaneous effects of several factors, and thus searching optimum conditions for desirable responses [Citation13]. Finally, concave optimization is widely applied to achieve to search the optimal conditions in the polynomial equation obtained because of guarantee of global optimization, which has been successfully applied in such areas as automatic control systems, estimation and signal processing, and so on [Citation14].
2. Materials and methods
2.1. Tested wastewater characteristics
The RO concentrate used in this work was collected from Wuhan Landfill treatment Engineering project, which was located in Wuhan city, China. The whole treatment process consisted of biological treatment (including anaerobic unit and A/O unit) followed by MBR unit, nanofiltration unit and RO unit. Prior to the experiment, the RO concentrate samples were stored at 4°C in order to keep the raw wastewater characteristics unchanged. The wastewater characteristics were summarized in . The extremely low BOD5/COD ratio of 0.049 indicated the poor biodegradability of RO concentrate.
Table 1. Characteristics of the landfill leachate RO concentrate quality.
2.2. Apparatus and procedure
The experimental apparatus was composed of air pump, flow meters, rubber tube, air-bubble sparger and a plexiglax cylinder (V = 600 mL), as shown in . The plexiglax cylinder filled with granular active carbon and zero valent iron was used to investigate the degradation of RO concentrate. Granular active carbon used had a diameter of 0.2–1.5 mm, a height of 4.0 mm and specific surface area of around 925.2 m2/g. Prior to experiment, granular active carbon was washed with distilled water in turn and then dried at 105°C for 24 h. Scrap iron chosen used as the anode of ICME was obtained from a machine shop and were degreased in a 10% hot alkaline solution. Prior to experiment, scrap iron was firstly soaked in a 2% sulfuric acid solution to remove surface rusts, and finally cleaned by deionized water [Citation15]. The different level of surface area of scrap iron was chosen in accordance with the CCD design ( and ) . The RO concentrate with a total volume of 250 mL was poured into the reactor. The solution was distributed by the air pump and gas was supplied at the bottom of the reactor through a pottery aerator. The pH of solution was adjusted using 3.0 mol/L H2SO4 or NaOH solution at certain time (five minutes) intervals. ICME coupled with hydrogen peroxide process lasted about 45 min to ensure equilibrium. After the reaction period, the supernatant was taken for water quality measurements after 30 min solids sedimentation and then was filtered immediately through a 0.22 µm membrane filter. The filtrate was analyzed for COD measurement by potassium dichromate oxidant method (Hach Corporation, USA). Each experiment was duplicated under identical conditions at room temperature.
Table 2. Experiment ranges and levels of the independent variables.
Table 3. Corresponding results, experimental design and levels of three independent variables based on Central composite design (CCD) for COD removal efficiency after treatment.
2.3. Experimental design and data analysis
In this study, three known critical variables which affect COD removal efficiency: the C/Fe (Surface area) (X1), H2O2 concentration (X2) and the pH (X3) were selected as the three independent variables based on the preliminary experiments. A Central composite designs (CCD) with 20 experiments was carried out for the optimization of the process variables. The range and levels of the variables (high and low) investigated in the work were summarized in . Each of the variables was coded at five levels: −α (−1.68), −1, 0, +1, and +α (+1.68). The variable xi was coded as Xi according to the following relationship:
Where Xi is the coded value of an independent variable, xi is corresponding natural value, x0 is the natural value of an independent variable at the center point and δxi is the step change value. The behavior of COD removal can be modeled by the following second order polynomial equation:
Where Y is the response variable, β0 is the intercept term, β1, β2 and β3 are linear coefficients, β12, β22 and β33 are quadratic coefficients, and X1, X2 and X3 are the coded values of the three independent variables.
Minitab 15 statistical software (USA) was applied to arrange the 20 experiments in CCD and further analyze the experimental data obtained in this study. The optimization of this model was performed using the convex optimization method.
3. Results and discussion
3.1. Optimization for COD removal efficiency using ICME coupled with H2O2 and ANOVA analysis
The equation of ICME coupled with H2O2 process was carried out in CCD to illustrate the effect of three independent variables on COD removal efficiency and the optimal condition. A total 20 experiments’ result of COD removal efficiency in CCD was showed in . Fourteen experiments were augmented with six replications carried out at the centre values (zero level) to evaluate the pure error. The values of all regression coefficients with the significance levels were exhibited in . According to the experimental design and result, the regression model obtained by the application of RSM is given by following equation:
Where Y is the value of COD after ICME coupled with H2O2 process, x1(C/Fe ratio (Surface area) of ICME granule chosen), x2 (concentration of H2O2) and x3 (pH of the solution) are the coded values of the test variables.
Statistical testing of the equation was carried out by analysis of variance (ANOVA) and the results for the coded variable levels are shown in . It will be noted that P < 0.05 indicates that the model terms are significant at 95% confidence level or more. The predicted value of R2 (0.9754) was close to the adjusted R2 (0.953), indicating a good agreement between the model-predicted and experimental values. The adequacy of models was further tested through lack-of-fit F-tests. The lack of fit F-statistic was not statistically significant because of the P-value (0.065) greater than 0.05, which suggests that it can be valid to predict the response.
Table 4. Analysis of variance (ANOVA) for the response surface quadratic model for COD removal.
To find optimal variables condition in a quadratic equation, the traditional method is firstly to solve the partial derivative of the target function and then force it to zero. However, the traditional method can’t ensure global optimality when the objective function investigated is non-convex (or non-concave) owing to the appearance of many extreme points. Differently, in the present study, convex optimization method was chosen to pursue the optimal conditions for maximum COD removal efficiency. Therefore, the work was to initially prove that the objective function is concave through convex optimization, and then proceed to achieve the solution of the objective function using the Lagrange multiplier method. In order to complete the first step to demonstrate that Z is a concave function, the following was performed:
By introducing ,Equation (3) can be alternatively solved by:
Where A and B are coefficient matrices, its form is as follows:
The Hessian matrix of Equation (4) is described by .
Eigen-decomposition of ∇2Y yields eigenvalues of −7.1151, −6.5545 and −3.6976. Since this Hessian matrix is negative, the original objective function of the optimization problem is a concave function. According to the convex optimization method to find the global maximum Y, we can discover a maximum point and the corresponding
.
A maximum response of 86.1% of COD removal efficiency can be predicted by the model at the following optimal conditions: C/Fe (Surface area) of 717,143.8, H2O2 of 1687.6 mg/L and pH of 3.8. The result of the actual experiment under these optimal conditions was found as 86.9%. The remain COD in the effluent was 267.2 mg/L, which was less than 300 mg/L. Consequently, the effluent discharged can meet the level B standards of Wastewater Quality Standards for Discharge to Municipal Sewers (CJ 343—2010).
3.2. Effect of various variables on optimization process and 3D response surface plots
represented the 3D response surface plots of the COD removal efficiency as functions of C/Fe (Surface area),H2O2 concentration and pH, respectively, with all of the other factors fixed. The 3D response surface plots are helpful in understanding both the main independent variables and the interaction effects. As observed in , three independent variables had significant impact on COD removal. Increasing the H2O2 concentration from 659.1 mg/L to around 1687.6 mg/L can significantly enhance COD removal efficiency from 48% to 82% ()). In the Fe-C micro-electrolysis system (ICME), Fe° acts as anode and loses two electrons to form Fe2+. The cell reactions of the Fe-C micro-electrolysis system can be represented as follows:
Figure 2. 3D plots showing (a) the effect of concentration of H2O2 and pH, (b) the effect of C/Fe and pH, (c) the effect of C/Fe and concentration of H2O2 on the COD removal.
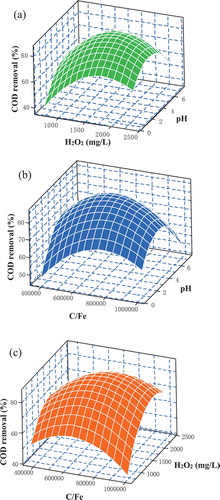
Anodic oxidation:
Cathode reduction (Acidic with oxygen):
In ICME coupled with hydrogen peroxide process, organic contaminants can be firstly reduced by [H] during ICME process. Furthermore, the majority of COD abatement can be accounted for the following Fenton reaction:
The Fenton reaction is a homogeneous catalytic oxidation process using a mixture of H2O2 and Fe2+ (and Fe3+) to produces a hydroxyl radical and a superoxide radical [Citation16]. The fate of organic compounds and their degradation by products is primarily dependant on their reaction with hydroxyl radicals [Citation17]. Hydroxyl radicals attack the organic pollutants and lead to the complete destruction of contaminants to CO2, water and inorganic salts as end products [Citation18].
However, it should be emphasized that ICME coupled with H2O2 activity will become less if more H2O2 is provided. As can be seen from , at a concentration of more than 1687.6 mg/L, enhancement in COD removal efficiency was observed, even slightly decrease when concentration of H2O2 was increased to 2000 mg/L. This finding may be explained by the fact that excessive H2O2 will react with hydroxyl radicals, which will lead to the abatement of hydroxyl radicals [Citation19].
Another important operating parameter in the ICME coupled with H2O2 process is the concentration of Fe0 required as catalyst. In the Fe-C micro-electrolysis system, iron and activated carbon will act as anode and cathode, respectively. When iron and activated carbon particles are fully mixed and contacted with each other in wastewater, massive microscopic galvanic cells will be formed spontaneously between the anode and cathode [Citation20]. Organic pollutant can be reduced by Fe2+ release from this system by a series of physicochemical reaction such as electro-flocculation, electrostatic reaction and oxidation-reduction reaction [Citation11]. Lower C/Fe ratio (Surface area) of ICME granule chosen means more Fe which can release from anode to form Fe2+. In the classic Fenton oxidation process, high concentration of Fe2+ can cause an enhancement of degradation efficiency since Fe2+ determines the rate of the conversion of H2O2 to free radical. It was apparent from that when C/Fe ratio reduced from 1,002,836–717,143.8, COD removal efficiency can reach the range from 65–83% under the condition of concentration of 1500 mg/L in the acid environment. On the other hand, the existence of optimal C/Fe ratio can be explained by reaction (6) – (12):
Obviously, excess of iron ions will react with hydroxyls radical and inevitably contribute to a scavenging effect on hydroxyl radicals.
pH is one of the major factors that limits the performance of both ICME and Fenton related processes. It was obvious from that in the case of highly acidic pH (between 2.5 and 4.0), the highest ICME coupled with H2O2 process activity was attained. No obvious difference in degradation efficiency can be found when pH was in the range from 2.5–4.0. In ICME process, more Fe2+ can release from anode in acidic solution. In following Fenton process, the production of hydroxyl radicals in the pH range 2–4 is higher because of either H2O2 regeneration or increase in reaction rates [Citation21]. A similar conclusion was demonstrated in a previous study that stable hydroxyl radicals were produced at pH values of 2–4 and that high oxidizing potential was exhibited in this pH range [Citation22]. Above pH 5.0, the iron ions especially the Fe3+ will precipitate, which will inhibit the regeneration of ferrous ions. Unavoidably, the catalyst dosage in Fenton’s reaction decreases. Further, under alkaline condition, hydrogen peroxide is unstable and doesn’t produce any hydroxyls radicals [Citation23].
4. Conclusion
From the experimental data, it was concluded that a combined technology between Iron-carbon micro-electrolysis (ICME) and H2O2 can exhibit the huge potential in significantly reducing COD in treating landfill leachate RO concentration. A quadratic equation proposed by response surface methodology (RSM) was proved to accurately predict the effect of three independent variables on COD removal efficiency. By the Convex optimization method, C/Fe (Surface area) of 717,143.8, H2O2 concentration of 1687.6 mg/L and pH of 3.8 were found to be optimum for maximum COD removal efficiency. Under the optimal condition, a maximum COD removal efficiency of 86.9% can be achieved. After treatment, the effluent COD was less than 300 mg/L, which was often applied for cooling hot slag.
Acknowledgments
We wish to thank the anonymous reviews and editors for their valuable suggestions on revising and improving the work.
Disclosure statement
No potential conflict of interest was reported by the authors.
Additional information
Funding
References
- Oulego P, Collado S, Laca A, et al. Tertiary treatment of biologically pre-treated landfill leachates by non-catalytic wet oxidation. Chem Eng J. 2015;273:647–655.
- Cortez S, Teixeira P, Oliveira R, et al. Evaluation of Fenton and ozone-based advanced oxidation processes as mature landfill leachate pre-treatments. J Environ Manage. 2011;92(3):749–755.
- Qi XX, Zhang CJ, Zhang Y. Treatment of landfill leachate RO concentrate by VMD. International Conference on Circuits and Systems (CAS). Paris, France. 2015;13–17.
- Wu YY, Zhou SQ, Zheng K, et al. Mathematical model analysis of Fenton oxidation of landfill leachate. Waste Manage. 2011;31:468–474.
- Zhang J, Yang T, Wang HY, et al. Study on treating old landfill leachate by Ultrasound–fenton oxidation combined with MAP chemical precipitation. Chem Speciation Bioavailability. 2016;27(4):175–182.
- Ji FZ, Zhang H, Li J, et al. Treatment of reverse osmosis (RO) concentrate from an old landfill site by Fe0/PS/O3 process. J Chem Technol Biotechnol. 2017;92:2616–2625.
- Klavariot M, Mantzavinos D, Kassinos D. Removal of residual pharmaceuticals from aqueous systems by advanced oxidation processes. Environ Int. 2009;35:402–417.
- Wang KL, Liu SQ, Zhang Q, et al. Pharmaceutical wastewater treatment by internal micro-electrolysis-coagulation, biological treatment and activated carbon adsorption. Environ Technol. 2009;30:1469–1474.
- Wang MX, Zhu SQ, He XW. Study on micro-electrolysis treatment for decolorizing dyed water. J China Univ Min Tech (China). 2001;11:212–216.
- Sun L, Wang C, Ji M, et al. Treatment of mixed chemical wastewater and the agglomeration mechanism via an internal electrolysis filter. Chem Eng J. 2013;215:50–56.
- Yang Z, Ma Y, Liu Y, et al. Degradation of organic pollutants in near-neutral pH solution by Fe-C micro-electrolysis system. Chem Eng J. 2017;315:403–414.
- Deng Y, Englehardt JD. Treatment of landfill leachate by the Fenton process. Water Res. 2006;40:3683–3694.
- Bezerraa MA, Santelli RE, Oliverira EP, et al. Response surface methodology (RSM) as a tool for optimization in analytical chemistry. Talanta. 2008;76:965–977.
- Boyd S, Vandenberghe L. Convex optimization. New York (NY): Cambridge University Press; 2004.
- Cheng H, Xu W, Liu J, et al. Pretreatment of wastewater from triazine manufacturing by coagulation, electrolysis, and internal micro-electrolysis. J Hazard Mater. 2007;146:385–392.
- Fan XQ, Hao HY, Wang YC, et al. Fenton-like degradation of nalidixic acid with Fe3+/H2O2. Environ Sci Pollut Res. 2013;20:3649–3656.
- Pignatello JJ, Oliveros E, MacKay A. Advanced oxidation processes fororganic contaminant destruction based on the Fenton reaction and related chemistry. Crit Rev Environ Sci Technol. 2006;36:1–84.
- Muhammad U, Hamidi AA, Mohd SY. Review: trends in the use of Fenton, electro-Fenton and photo-Fenton for the treatment of landfill leachate. Waste Manage. 2010;30:2113–2121.
- Ghaly MY, Härtel G, Mayer R, et al. Photochemical oxidation of p-chlorophenol by UV/H2O2 and photo-Fenton process. A comparative study. Waste Manage. 2001;21:41–47.
- Luo JH, Song GY, Liu JY, et al. Mechanism of enhanced nitrate reduction via micro-electrolysis at the powdered zero-valent iron/activated carbon interface. J Colloid Interface Sci. 2014;435:21–25.
- Sedlak DL, Andren AW. Oxidation of chlorobenzene with Fenton’s reagent. Environ Sci Technol. 1991;25:777–782.
- Deng Y. Physical and oxidative removal of organics during Fenton treatment of mature municipal landfill leachate. J Hazard Mater. 2007;146:334–340.
- Rivas FJ, Beltrán FJ, Gimeno O, et al. Optimisation of Fenton’s reagent usage as a pre-treatment for fermentation brines. J Hazard Mater. 2003;96:277–290.