Abstract
Purpose: The purpose of this study is to systematically study the cell-cycle alterations of glioblastoma stem-like cells (GSLCs) after irradiation, possibly enriching the mechanisms of radioresistance of GSLCs.
Materials and methods: GSLCs were enriched and identified, and then the radioresistance of GSLCs was validated by analyzing cell survival, cell proliferation, and radiation-induced apoptosis. The discrepancy of the cell-cycle distribution and expression of cell-cycle-related proteins between GSLCs and glioblastoma differentiated cells (GDCs) after irradiation was completely analyzed.
Results: The survival fractions and the cell viabilities of GSLCs were significantly higher than those of GDCs after irradiation. Radiation-induced apoptosis was less prominent in GSLCs than in GDCs. After irradiation with high-dose X-rays, the percentages of GDCs in G2/M phase was evidently increased. However, radiation-induced G2/M arrest occurred less frequently in GSLCs, but S-phase arrest occurred in GSLCs after irradiation with 8 Gy. Further mechanistic studies showed that the expressions levels of Cdc25c, Cdc2, and CyclinB1 in GSLCs were not apparently changed after irradiation, while those of p-ATM and p-Chk1 were sharply increased after irradiation in GSLCs. The basal level of Cdc25c expression in GSLCs was much higher than that in GDCs.
Conclusions: We explored the cell-cycle alterations and cell-cycle-related proteins expression levels in GSLCs after irradiation, providing a novel mechanism of radioresistance of GSLCs.
Introduction
Glioblastoma multiforme (GBM) is one of the most malignant tumors in the central nervous system (Wen and Kesari Citation2008; Stupp et al. Citation2009). Even with multimodal therapy strategies, including surgical resection, radiotherapy and chemotherapy, the prognosis remains extremely poor with a median survival of only 14.6 months (Stupp et al. Citation2009; Binello and Germano Citation2011). Numerous studies have demonstrated the intra-tumoral heterogeneity of GBMs by the presence of a small cell clone harboring stem-like properties called glioblastoma stem-like cells (GSLCs) (Singh et al. Citation2004; Magee et al. Citation2012; Patel et al. Citation2014). GSLCs generate glioblastoma differentiated cells (GDCs) with the small stem-like cell subpopulation and their properties maintained (Bassoy et al. Citation2016). It is considered that GSLCs are more aggressive and radio- and chemo-resistant than GDCs; consequently, GSLCs are the source of the recurrence of GBMs and contribute to treatment failure (Bao et al. Citation2006; Hermann et al. Citation2010; Rycaj and Tang Citation2014).
Accumulating evidence exists concerning the radioresistance of GSLCs (Bao et al. Citation2006; Lomonaco et al. Citation2009; Wang J et al. Citation2010). Bao et al. (Citation2006) have found that the proportion of survived CD133-positive GSLCs after radiation was increased relative to that of CD133-negative GDCs, and this finding was attributed to greater activation of checkpoints, such as ATM, Rad17, Chk1, and Chk2, in response to irradiation. Additionally, recent studies have shown that Chk1, but not Chk2, exerts dominant functions in the DNA damage repair of GSLCs after irradiation (Uto et al. Citation2004; Wu et al. Citation2012; Bo et al. Citation2014).
It is commonly accepted that radiation could induce G2/M arrest through activation of the ATM/Chk1 pathway in p53-deficient cancer cells (Sancar et al. Citation2004; Lossaint et al. Citation2011; Nahar et al. Citation2014). Radiation-induced G2/M arrest is regulated by many factors, among which the ATM/Chk1/Cdc25c/Cdc2 pathway plays the most essential role in cancer cells (Bucher and Britten Citation2008; Dai and Grant Citation2010).
However, interestingly, we have found that radiation-induced G2/M arrest rarely occurred in GSLCs in our study. This finding is likely correlated with the radioresistance of GSLCs. To shed light on the mechanisms of this finding, we first compared the expression of cell-cycle-related proteins in GSLCs with that in GDCs after irradiation, the results of which are expected to offer a novel perspective on the theory of the radioresistance of GSLCs.
Materials and methods
Cell culture
Human glioblastoma cell lines U87 and U251 were purchased from American Type Culture Collection (ATCC). They were cultured in Dulbecco’s modified Eagle’s medium (DMEM) (HyClone, Logan, UT) containing 10% fetal bovine serum (FBS) (HyClone, Logan, UT) under standard incubator conditions (95% humidified air, 5% CO2, 37 °C). Additionally, U87 and U251 were cultured as spheres (U87-sph and U251-sph) in serum-free DMEM/F12 medium (HyClone, Logan, UT) in the presence of epidermal growth factor (EGF, 20 ng/ml) (PeproTech, Rocky Hill, NJ), basic fibroblast growth factor (bFGF, 20 ng/ml) (PeproTech, Rocky Hill, NJ), recombinant human leukemia inhibitor factor (rhLIF, 10 ng/ml) (Millipore, Billerica, MA) and 1 × B27 supplement (Gibco, Grand Island, NY). After 8 passages, U87-sph and U251-sph were harvested and analyzed for the expression of stem cell markers.
Reagents and antibodies
PE-conjugated human CD133/1 antibody for FACS analysis was obtained from Miltenyi Biotec (Bergisch Gladbach, Germany). The following antibodies were used in the immunofluorescence assay: CD133 (1:100; Biorbyt Ltd, Cambridge, UK), nestin (1:250; Boster, Wuhan, China), and Sox-2 (1:250; ABGENT, San Diego, CA). The antibodies used in western blot analysis were as follows: anti-p-ATM (1:1000), anti-p-Chk1 (1:1000), anti-p-Cdc25c (1:1000), anti-Cdc25c (1:1000), anti-p-Cdc2 (1:1000), anti-Cdc2 (1:1000), and anti-CyclinB1 (1:1000) (Cell Signaling Technology, Danvers, MA) and anti-GAPDH (1:500; Boster, Wuhan, China).
Immunofluorescence assay
Cells were washed with PBS and then were fixed for 30 min in 4% paraformaldehyde at room temperature. Permeabilization was conducted using 0.25% (v/v) Triton X-100 reagent (only for Sox-2 staining). Subsequently, cells were blocked in 10% (v/v) normal goat serum in PBS for 1 h at 37 °C and were incubated with primary antibodies overnight at 4 °C. After washing with PBS, cells were incubated with Dylight 488- or Dylight 594-conjugated secondary antibodies (1:250, Abbkine, Redlands, CA) for 1 h at room temperature, and cell nuclei were counterstained with DAPI. Images were captured using an Olympus fluorescence microscope (Olympus, Tokyo, Japan).
Flow cytometry analysis
Cells were harvested and washed twice in PBS solution containing 0.5% bovine serum albumin (BSA) and then were incubated with PE-conjugated CD133/1 antibody at 4 °C for 10 min in the dark. After washing twice with 0.5% BSA/PBS, the cells were subjected to FACS analysis.
Irradiation
The cells were irradiated with X-rays which were generated from a biological X-ray irradiator (RS2000, Rad Source Technologies, Boca Raton, FL). All irradiations were performed at room temperature and at a dose rate of 100 cGy/min.
Clonogenic assay
Cells were seeded in triplicate into six-well plates at different densities based on the doses of X-rays. The plates were coated with poly-l-lysine (PLL) and incubated 12 h prior to irradiation. Next, the plates were exposed to 0–8 Gy of X-ray radiation. Fourteen days after irradiation, the cells were stained with crystal violet and colonies comprising 50 cells or more were counted. The survival fraction (SF) was calculated by the following formula: SF = (mean colonies number)/(initial number of plating cells × plating efficiency). Cell survival curves were determined using a multi-target single-hit model and GraphPad Prism software (San Diego, CA).
Proliferation assay
Cell proliferation was analyzed using the cell counting kit-8 (CCK-8) assay (Dojindo, Kumamoto, Japan). Cells were seeded in 8 replicates at 5000 cells/wells into poly-l-lysine (PLL)-coated 96-well plates. The cells were then irradiated with 4 Gy or 8 Gy of X-rays. At different time points after irradiation, CCK-8 solution was added to each well, followed by incubation at 37 °C for 3 h. The absorbance was examined at a wavelength of 450 nm by a microplate reader with the subtraction of baseline reading. Cell viability was defined as follows: cell viability (%) = Absorbance of irradiated well/Absorbance of unirradiated control well ×100%.
Cell-cycle analysis
At different time points after irradiation, the cells (in triplicate) were harvested and fixed in 70% ethanol at 4 °C for 24 h and then were stained with propidium iodide (PI) using the Cell Cycle Detection Kit (Beyotime, Shanghai, China) according to the instructions from the manufacturer. Finally, PI-stained cells were analyzed using the FACSCalibur flow cytometer from BD Biosciences (Franklin Lakes, NJ).
Annexin V-FITC/PI staining assay
Forty-eight hours after irradiation, the apoptotic rates of the cells were assessed using the Annexin V-FITC/PI Apoptosis Detection Kit (KeyGEN Biotech, Nanjing, China) according to the protocols from the manufacturer. Briefly, the harvested cells were resuspended in binding buffer containing 5 μl of Annexin V-FITC and 5 μl of PI and were incubated in the dark at room temperature for 15 min. The cells were then analyzed by flow cytometry immediately.
Caspase-3 activity assay
At 24 h and 48 h after irradiation, the caspase-3 activity of the cells was evaluated using the Caspase 3 Activity Assay Kit (Beyotime, Shanghai, China). Briefly, cells were harvested and lysed in lysis buffer on ice for 15 min, and the supernatants were collected and measured for the protein concentration. The lysates were then incubated with Ac-DEVD-pNA (2mM) at 37 °C for 60 min. Caspase-3 activity was determined based on the absorbance at a wavelength of 405 nm by a microplate reader.
Western blot analysis
At different time points after irradiation, the cells were collected and lysed in lysis buffer containing protease inhibitors. The protein concentrations were measured using the BCA protein assay kit (Beyotime, Shanghai, China). Equal amounts of total protein were separated by SDS-PAGE and were transferred onto nitrocellulose membranes. After blocking with 5% non-fat milk in TBST (Tris-buffered saline with 0.1% Tween-20) at room temperature for 1 h, the membranes were incubated with primary antibodies at 4 °C overnight, subsequently washed with TBST and incubated with horseradish peroxidase-conjugated secondary antibodies (1:2000) (Proteintech, Chicago, IL) for 1 h at room temperature. The immunoreactive bands were visualized using an enhanced chemiluminescent (ECL) detection kit (Menlo Park, CA). The membranes were stripped with Restore Western Blot Stripping Buffer (Thermo Scientific, Rockford, IL) at 37 °C for 30 min. The band intensities were quantified by Quantity One software (Bio-Rad, Hercules, CA).
Statistical analysis
The experiments were performed three times. The data are expressed as the means ± standard error of the mean (SEM). Statistical analyses were performed by unpaired two-tailed Student’s t-test or analysis of variance (ANOVA). A p value < .05 was considered statistically significant. Statistical analysis was performed using statistical software (GraphPad Software, San Diego, CA).
Results
Spheroid culture for the enrichment of GSLCs from glioblastoma cell lines
To isolate and propagate GSLCs from glioblastoma cell lines in vitro, U87 and U251 cells were cultured in serum-free medium containing EGF, bFGF, B27 and LIF (GSLC medium), and then they formed sphere-like clones immediately. The tumor spheres grew slower than their parental cells, and they could maintain their sphere-forming ability over long-term culture in the GSLC medium (Supplementary Figures 1(A) and 2(A)).
To test whether these U87 and U251 spheres are enriched for GSLCs, after eight passages, the stem cell markers of cell spheres and their parental cell lines were detected. Flow cytometry analysis showed that the percentage of CD133-positive cells was much higher in U87-sph cells and U251-sph cells than in their parental glioblastoma cell lines (p < .01) (Supplementary Figures 1(B,C) and 2(C,D)). Immunofluorescence results showed that most U87-sph cells express CD133, nestin and Sox-2, whereas few U87 cells express these stem cell markers (Supplementary Figure 1(D)). The expression of CD133 in U251 and U251-sph demonstrated similar results (Supplementary Figure 2(B)). These results indicated that GSLCs could be enriched from glioblastoma cell lines cultured in serum-free medium.
GSLCs are more resistant to irradiation
To verify the theory that GSLCs are more resistant to irradiation, we performed the clonogenic survival assay and proliferation assay to study radiation-induced cell death in GSLCs. The results of the clonogenic assay showed that the survival fractions of both U87-sph and U251-sph were much higher than those of their parental glioblastoma cell lines after irradiation with different doses of X-ray (p < .01) (). Further study using the CCK-8 assay showed that the cell viabilities of cell spheres were significantly higher than those of their parental cell lines after 4 Gy or 8Gy of X-rays ().
Figure 1. Glioblastoma stem-like cells are resistant to irradiation. (A, B) Survival curves derived from clonogenic assay of U87 cells and U87-sph cells (A) and U251 cells and U251-sph cells (B) irradiated with 0-8 Gy of X-rays. Data from three independent experiments performed in triplicate are presented as the means ± SEM. (**p < .01, n = 3). (C, D) The cell viabilities of U87, U87-sph, (C) U251 and U251-sph (D) were analyzed by CCK-8 assay at different time points after treatment with 4 Gy and 8 Gy of X-rays. The relative cell viability (%) is quantified as a percentage compared to the control group (untreated group). Data from three independent experiments with eight replicates in each experiment are presented as the means ± SEM. (**p < .01, n = 3).
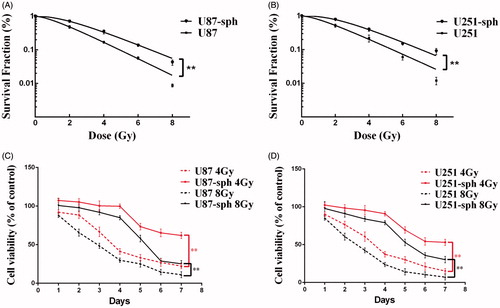
Radiation-induced apoptosis is less prominent in GSLCs
Because apoptosis is the primary manner of radiation-induced cell death, we compared radiation-induced apoptosis in GSLCs with that in their parental cells using the Annexin V-FITC/PI staining assay and Caspase-3 activity assay. The results showed that the apoptotic rates of U87-sph 48 h after irradiation were apparently lower than those of U87 cells when irradiated with 4 Gy and 8 Gy (p < .01) (). Further study showed that, when treated with 4 Gy or 8 Gy of X-rays, the caspase-3 activity levels of U87 cells and U251 cells were significantly higher than those of U87-sph and U251-sph, respectively, at both 24 h and 48 h after irradiation (p < .01). Nevertheless, when irradiated with 2 Gy of X-rays, there was no significant difference between GSLCs and their parental cells in caspase-3 activity (p > .05) ().
Figure 2. Radiation-induced apoptosis is less prominent in glioblatoma stem-like cells. (A) U87 and U87-sph were irradiated with X-ray with a dose of 4 Gy or 8 Gy, and harvested 48h after treatment. Then the cell apoptosis was assessed by Annexin V-FITC/PI assay. Representative images from three independent experiments are shown. (B) The bar charts show the mean cell apoptosis of three independent experiments performed in triplicate. The vertical axis represents apoptotic rates/baseline apoptotic rates (%/%). Unirradiated cells served as a control. (**p < .01, versus U87, n = 3). U87 and U87-sph (C) and U251 and U251-sph (D) were irradiated with X-ray with a dose of 2 Gy, 4 Gy, and 8 Gy. After cells were cultured for 24 h and 48 h, caspase-3 activity was measured. Untreated cells served as a control. (**p < .01, versus U87 or U251, n = 3).
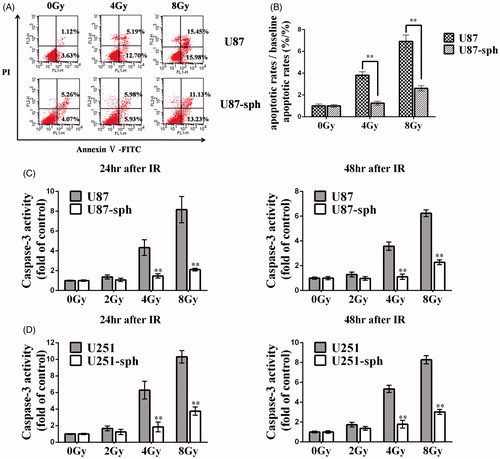
Radiation-induced G2/M arrest occurs less frequently in GSLCs
Because radiation-induced apoptosis is closely related to the cell cycle, we analyzed the discrepancy of the cell-cycle distribution after irradiation between GSLCs and GDCs. The percentage of G2/M phase U87 cells after irradiation with 8 Gy or 4 Gy was evidently higher than that of unirradiated U87 cells, especially at 12 h and 24 h after irradiation (p < .01) (). However, the percentage of G2/M phase U87-sph cells did not change notably after irradiation (); however, when treated with 8 Gy of X-rays, the percentage of S phase was significantly higher in U87-sph cells than in unirradiated U87-sph cells at 24 h after irradiation (p < .01) (). The results indicated that radiation-induced G2/M arrest occurred less frequently in U87-sph cells, but S-phase arrest occurred in U87-sph cells after irradiated with 8 Gy. We verified the results on another cell pair, U251 and U251-sph cells, and found that G2/M phase was apparently arrested in U251 cells at 24 h after irradiation with 8 Gy (p < .01) (), while S phase was arrested in U251-sph cells at 12 h, 24 h and 48 h, especially at 12 h and 24 h, after irradiation with 8 Gy (p < .05) ().
Figure 3. Radiation-induced G2/M arrest occurs less frequently in glioblatoma stem-like cells. (A) Cell-cycle analysis of U87 and U87-sph at different time points after irradiation with different doses. Representative images from three independent experiments are shown. (B) Cell-cycle analysis of U251 and U251-sph at different time points after irradiation with 8 Gy. Representative images from three independent experiments are shown. (C, D) The bar charts show the percentages of G2/M phase U87 and U87-sph (C) and U251 and U251-sph (D) at different time points after irradiation with 8 Gy (**p < .01, *p < .05, versus control, n = 3). (E, F) The bar charts show the percentages of S phase U87 and U87-sph (E) and U251 and U251-sph (F) at different time points after irradiation with 8 Gy (**p < .01, *p < .05, NS: non-significant, versus control, n = 3).
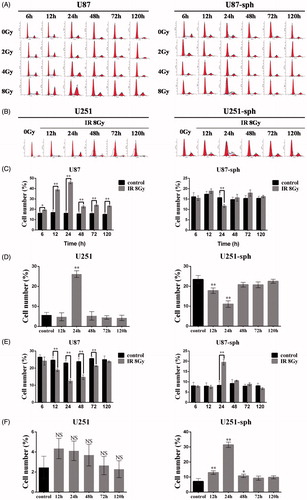
Differential expression of cell-cycle-related proteins after irradiation between GSLCs and GDCs
To explore the mechanisms underlying the different cell-cycle distributions after irradiation between GSLCs and GDCs, we analyzed the expression of cell-cycle-related proteins by western blotting. The expressions levels of p-ATM and p-Chk1 were increased time dependently in both U87 and U87-sph cells after irradiation, but the trend was more apparent in U87-sph cells (). Relative intensity analysis also showed that the expression of p-Chk1 in U87-sph cells was sharply increased after irradiation (p < .01), whereas only a slight increase was found in U87 cells (). The expression of Cdc25c in U87 cells was down-regulated, especially at 12 h, 24 h, and 36 h after irradiation compared with untreated U87 cells (p < .01). Correspondingly, the expression of p-Cdc25c in U87 cells was up-regulated, especially at 12 h, 24 h, and 36 h after irradiation. However, for U87-sph cells, the expression of Cdc25c and p-Cdc25c did not change apparently after irradiation, but Cdc25c was higher expressed in U87-sph cells than in U87 cells (). Western blot analysis of Cdc25c expression in U251 and U251-sph cells showed similar results (). The expression of Cdc2 in U87 cells was also down-regulated, especially at 12 h, 24 h, and 36 h after irradiation as Cdc25c expression (p < .01). Similarly, the trend of p-Cdc2 expression was the same as p-Cdc25c. For U87-sph cells, the expression of Cdc2 and p-Cdc2 was also unchanged, and the expression of p-Cdc2 was very low, similar to p-Cdc25c. CyclinB1 expression in both U87 cells and U251 cells after irradiation was similar to Cdc25c and Cdc2 expression, and CyclinB1 expression was slightly decreased at 24 h and 36 h after irradiation in U87-sph cells but was not changed in U251-sph cells ().
Figure 4. The expression of cell-cycle-related proteins in glioblatoma stem-like cells and their parental glioma cells after irradiation. (A) Cell lysates of U87 and U87-sph were extracted at different time points after treatment with 8 Gy of X-ray, and were analyzed by western blot assay for the expression of p-ATM, p-Chk1, p-Cdc25c, Cdc25c, p-Cdc2, Cdc2, and CyclinB1. GAPDH served as an internal loading control. (B) The bar charts show quantification of the bands intensity of p-Chk1, Cdc25c, Cdc2, and CyclinB1 from (A). Data from three independent experiments are presented as the means ± SEM (**p < .01, *p < .05, versus control, n = 3). (C) Cell lysates of U251 and U251-sph were extracted at different time points after treatment with 8 Gy of X-ray, and were analyzed by western blot assay for the expression of Cdc25c, Cdc2, and CyclinB1. GAPDH served as an internal loading control. (D) The bar charts show quantification of the bands intensity of Cdc25c and CyclinB1 from (C). Data from three independent experiments are presented as the means ± SEM. (**p < .01, *p < .05, versus control, n = 3).
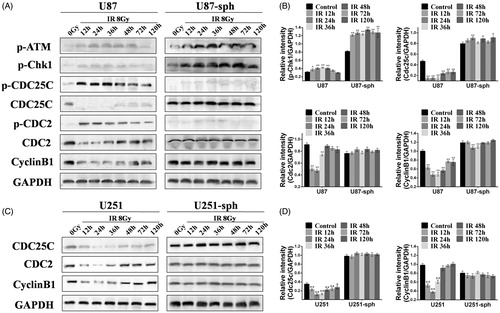
Discussion
Many studies have demonstrated that glioma stem cells are resistant to radiotherapy (Bao et al. Citation2006; Wang et al. Citation2010), but few have studied the cell-cycle changes of GSLCs after irradiation. It is commonly considered that radiation could induce cell-cycle arrest in tumor cells (Krueger et al. Citation2010; Nahar et al. Citation2014). However, in this paper, we first proposed a view that cell-cycle arrest rarely occurs in GSLCs after irradiation.
The strategies for the enrichment of glioma stem-like cells include serum-free medium culture (Iacopino et al. Citation2014), side population cell analysis (Tabu et al. Citation2016) and sorting by cell surface markers (Bao et al. Citation2006). We applied serum-free medium culture to enrich GSLCs as described in several previous studies (Iacopino et al. Citation2014; Balvers et al. Citation2015).
Our results showed that the apoptotic rates and caspase-3 activities of irradiated GSLCs were lower than those of glioma cell lines, indicating that GSLCs were not sensitive to radiation-induced apoptosis. The results validate the theory that GSLCs are more resistant to radiation than glioma differentiated cells (Bao et al. Citation2006; Ahmed et al. Citation2015).
Recently, several researchers have focused on the mechanisms of the radioresistance of GSLCs. The main viewpoints include DNA damage repair (Ahmed et al. Citation2015), pro-survival pathway (Berger et al. Citation2011), and GSLC niches (Fidoamore et al. Citation2016), but none could completely exclusively shed light on the mechanisms. The radioresistance of GSLCs is a complicated phenomenon involving multiple factors (Rycaj and Tang Citation2014; Osswald et al. Citation2015). Bao et al. (Citation2006) have found that the expression of p-ATM and p-Chk1 was significantly up-regulated in CD133-positive GSCs after radiation compared with CD133-negative GDCs, indicating CD133-positive GSCs show greater checkpoint activation in response to DNA damage. In our study, we have also found a sharp increase in p-ATM and p-Chk1 expression in GSLCs after IR, and their expression in unirradiated GSLCs was higher than that in unirradiated GDCs.
Activation of the ATM/Chk1 pathway could phosphorylate Cdc25c at Serine-216 (Ser-216) (Peng et al. Citation1997; Zhao et al. Citation2012; Xue et al. Citation2016). The protein phosphatase Cdc25c regulates entry into mitosis by activating the Cdc2/CyclinB1 kinase complex. At the onset of mitosis, Cdc2 is dephosphorylated by Cdc25c at Threonine-14 (Thr-14) and Tyrosine-15 (Tyr-15), the Cdc2/CyclinB complex translocates into the nucleus, and the cell-cycle progresses into M phase (Hutchins and Clarke Citation2004; Perdiguero and Nebreda Citation2004; Boutros et al. Citation2007). Cdc25c and Cdc2 are both critical regulators in G2/M transition and are modulated by many factors. Previous studies have suggested that activation of the ATM/Chk1 pathway could inhibit Cdc25c activation, thereby preventing dephosphorylation of Cdc2 and entry into mitosis, as well as cell-cycle arrest at the G2/M phase (De Souza et al. Citation2000; Sancar et al. Citation2004; Boutros et al. Citation2007). However, in our study, we found that the percentage of G2/M phase GSLC cells was unchanged after irradiation, although there was high expression of p-ATM and p-Chk1. For GDCs, G2/M arrest occurred at 12 h and 24 h after irradiation with 4 Gy or 8 Gy, and both ATM and Chk1 were activated after irradiation.
Thus, G2/M arrest was not subsequently induced by ATM/Chk1 pathway activation in GSLCs as their parental GDCs. To clarify the mechanisms of this difference between GSLCs and GDCs, we analyzed the expression of cell-cycle-related proteins using western blotting. The expression of Cdc25c was apparently higher in GSLCs than in GDCs and was almost unchanged after irradiation. Considering that Cdc25c is considered to be related to radioresistance (Zhao et al. Citation2012; Li et al. Citation2013), high expression of Cdc25c in GSLCs also indicates that GSLCs are more resistant to irradiation. Because the quantity of total Cdc25c protein in GSLCs is large, phosphorylated Cdc25c only accounts for a very small proportion of total protein: the remaining phosphatase Cdc25c remains sufficient to drive the cell cycle. This is one likely explanation for the inconsistency between activated ATM/Chk1 and G2/M arrest. Second, the expression of Cdc25c and Cdc2 was influenced by many other factors, including 14-3-3, Gadd45, p21, and Wee1 (Wang XW et al. Citation1999; Abbas and Dutta Citation2009). G2/M arrest was not only controlled by the ATM/Chk1 pathway after irradiation but was also regulated by these proteins, whereas the detailed mechanisms remain to be elucidated.
Additionally, low-dose irradiation could not induce G2/M arrest in U87 cells, indicating U87 cells possess inherent radioresistance. Considering the time-dependence and dose-dependence of G2/M arrest in U87 cells, we conclude that cell-cycle arrest likely occurred in GSLCs after irradiation with a dose above 8 Gy. Our results showed the percentage of S phase was a little increased in GSLCs after irradiation with 8 Gy; hence, ultra-high-dose irradiation is likely to lead to S phase arrest in GSLCs. However, further study should be performed to validate this conclusion.
Conclusions
Overall, we explored the radioresistance of GSLCs through cell-cycle changes and cell-cycle-related protein expression after irradiation, uncovering that radiation-induced G2/M arrest rarely occurred in GSLCs, and the expression levels of Cdc25c, Cdc2, and CyclinB1 were not apparently changed after irradiation, although ATM and Chk1 were mostly activated in GSLCs after irradiation. The basal level of Cdc25c expression in GSLCs was much higher than that of GDCs. These findings might provide a novel mechanism of the radioresistance of GSLCs.
Supplementary_file.docx
Download MS Word (7.1 MB)Disclosure statement
The authors report no conflicts of interest. The authors alone are responsible for the content and writing of the paper.
Additional information
Funding
Notes on contributors
Junfeng Liu
Junfeng Liu, his research focuses on the mechanisms of radioresistance of glioma stem-like cells and the roles of microenvironment in the radioresistance of tumors.
Yu Liu
Yu Liu, Tao Xie, Longjun Luo, and Cheng Xu are postgraduate students. Their research interests are enrichment of glioma stem-like cells, radiation-induced apoptosis and radioresistance of glioma stem-like cells.
Tao Xie
Yu Liu, Tao Xie, Longjun Luo, and Cheng Xu are postgraduate students. Their research interests are enrichment of glioma stem-like cells, radiation-induced apoptosis and radioresistance of glioma stem-like cells.
Longjun Luo
Yu Liu, Tao Xie, Longjun Luo, and Cheng Xu are postgraduate students. Their research interests are enrichment of glioma stem-like cells, radiation-induced apoptosis and radioresistance of glioma stem-like cells.
Cheng Xu
Yu Liu, Tao Xie, Longjun Luo, and Cheng Xu are postgraduate students. Their research interests are enrichment of glioma stem-like cells, radiation-induced apoptosis and radioresistance of glioma stem-like cells.
Qinglei Gao
Qinglei Gao, her research focuses on the mechanisms of metastasis and treatment resistance of malignant tumors, especially ovarian cancer.
Lu Shen
Lu Shen, her research focuses on cell senescence, ovarian aging and animal experiments.
Feng Wan
Feng Wan, his current research interests center on the differentiation of glioma stem-like cells and the effects of hypoxia on brain tumor cells.
Ting Lei
Ting Lei, his research focuses on tumorigenesis and development of brain tumors, especially pituitary tumors.
Fei Ye
Fei Ye, his research focuses on molecular mechanisms of radioresistance of glioma stem-like cells and molecular targeted therapy for gliomas.
References
- Abbas T, Dutta A. 2009. p21 in cancer: intricate networks and multiple activities. Nat Rev Cancer. 9:400–414.
- Ahmed SU, Carruthers R, Gilmour L, Yildirim S, Watts C, Chalmers AJ. 2015. Selective inhibition of parallel DNA damage response pathways optimizes radiosensitization of glioblastoma stem-like cells. Cancer Res. 75:4416–4428.
- Balvers RK, Lamfers MLM, Kloezeman JJ, Kleijn A, Pont LMEB, Dirven CMF, Leenstra S. 2015. ABT-888 enhances cytotoxic effects of temozolomide independent of MGMT status in serum free cultured glioma cells. J Transl Med. 13:74.
- Bao S, Wu Q, McLendon RE, Hao Y, Shi Q, Hjelmeland AB, Dewhirst MW, Bigner DD, Rich JN. 2006. Glioma stem cells promote radioresistance by preferential activation of the DNA damage response. Nature. 444:756–760.
- Bassoy EY, Chiusolo V, Jacquemin G, Riccadonna C, Walker PR, Martinvalet D. 2016. Glioma stemlike cells enhance the killing of glioma differentiated cells by cytotoxic lymphocytes. PLoS One. 11:e0153433.
- Berger R, Jennewein C, Marschall V, Karl S, Cristofanon S, Wagner L, Vellanki SH, Hehlgans S, Rodel F, Debatin KM, et al. 2011. NF-κB is required for Smac mimetic-mediated sensitization of glioblastoma cells for γ-irradiation-induced apoptosis. Mol Cancer Ther. 10:1867–1875.
- Binello E, Germano IM. 2011. Targeting glioma stem cells: a novel framework for brain tumors. Cancer Sci. 102:1958–1966.
- Bo S, Hui H, Li W, Hui L, Hong X, Lin D, Dai WX, Wu YH, Ai XH, Hao J, et al. 2014. Chk1, but not Chk2, is responsible for G2/M phase arrest induced by diallyl disulfide in human gastric cancer BGC823 cells. Food Chem Toxicol. 68:61–70.
- Boutros R, Lobjois V, Ducommun B. 2007. CDC25 phosphatases in cancer cells: key players? Good targets? Nat Rev Cancer. 7:495–507.
- Bucher N, Britten CD. 2008. G2 checkpoint abrogation and checkpoint kinase-1 targeting in the treatment of cancer. Br J Cancer. 98:523–528. *eng.
- Dai Y, Grant S. 2010. New insights into checkpoint kinase 1 in the DNA damage response signaling network. Clin Cancer Res. 16:376–383.
- De Souza CP, Ellem KA, Gabrielli BG. 2000. Centrosomal and cytoplasmic Cdc2/cyclin B1 activation precedes nuclear mitotic events. Exp Cell Res. 257:11–21.
- Fidoamore A, Cristiano L, Antonosante A, d'Angelo M, Di Giacomo E, Astarita C, Giordano A, Ippoliti R, Benedetti E, Cimini A. 2016. Glioblastoma stem cells microenvironment: the paracrine roles of the Niche in drug and radioresistance. Stem Cells Int. 2016:6809105.
- Hermann PC, Bhaskar S, Cioffi M, Heeschen C. 2010. Cancer stem cells in solid tumors. Semin Cancer Biol. 20:77–84.
- Hutchins JR, Clarke PR. 2004. Many fingers on the mitotic trigger: post-translational regulation of the Cdc25C phosphatase. Cell Cycle. 3:41–45.
- Iacopino F, Angelucci C, Piacentini R, Biamonte F, Mangiola A, Maira G, Grassi C, Sica G. 2014. Isolation of cancer stem cells from three human glioblastoma cell lines: characterization of two selected clones. PLoS One. 9:e105166.
- Krueger SA, Wilson GD, Piasentin E, Joiner MC, Marples B. 2010. The effects of G2-phase enrichment and checkpoint abrogation on low-dose hyper-radiosensitivity. Int J Radiat Oncol Biol Phys. 77:1509–1517.
- Li J, Yang CX, Mei ZJ, Chen J, Zhang SM, Sun SX, Zhou FX, Zhou YF, Xie CH. 2013. Involvement of cdc25c in cell cycle alteration of a radioresistant lung cancer cell line established with fractionated ionizing radiation. Asian Pac J Cancer Prev. 14:5725–5730.
- Lomonaco SL, Finniss S, Xiang C, Decarvalho A, Umansky F, Kalkanis SN, Mikkelsen T, Brodie C. 2009. The induction of autophagy by gamma-radiation contributes to the radioresistance of glioma stem cells. Int J Cancer. 125:717–722.
- Lossaint G, Besnard E, Fisher D, Piette J, Dulic V. 2011. Chk1 is dispensable for G2 arrest in response to sustained DNA damage when the ATM/p53/p21 pathway is functional. Oncogene. 30:4261–4274.
- Magee JA, Piskounova E, Morrison SJ. 2012. Cancer stem cells: impact, heterogeneity, and uncertainty. Cancer Cell. 21:283–296.
- Nahar K, Goto T, Kaida A, Deguchi S, Miura M. 2014. Effects of Chk1 inhibition on the temporal duration of radiation-induced G2 arrest in HeLa cells. J Radiat Res. 55:1021–1027.
- Osswald M, Jung E, Sahm F, Solecki G, Venkataramani V, Blaes J, Weil S, Horstmann H, Wiestler B, Syed M, et al. 2015. Brain tumour cells interconnect to a functional and resistant network. Nature. 528:93–98.
- Patel AP, Tirosh I, Trombetta JJ, Shalek AK, Gillespie SM, Wakimoto H, Cahill DP, Nahed BV, Curry WT, Martuza RL, et al. 2014. Single-cell RNA-seq highlights intratumoral heterogeneity in primary glioblastoma. Science. 344:1396–1401.
- Peng CY, Graves PR, Thoma RS, Wu Z, Shaw AS, Piwnica-Worms H. 1997. Mitotic and G2 checkpoint control: regulation of 14-3-3 protein binding by phosphorylation of Cdc25C on serine-216. Science. 277:1501–1505.
- Perdiguero E, Nebreda AR. 2004. Regulation of Cdc25C activity during the meiotic G2/M transition. Cell Cycle. 3:733–737.
- Rycaj K, Tang DG. 2014. Cancer stem cells and radioresistance. Int J Radiat Biol. 90:615–621.
- Sancar A, Lindsey-Boltz LA, Unsal-Kacmaz K, Linn S. 2004. Molecular mechanisms of mammalian DNA repair and the DNA damage checkpoints. Annu Rev Biochem. 73:39–85.
- Singh SK, Hawkins C, Clarke ID, Squire JA, Bayani J, Hide T, Henkelman RM, Cusimano MD, Dirks PB. 2004. Identification of human brain tumour initiating cells. Nature. 432:396–401.
- Stupp R, Hegi ME, Mason WP, van den Bent MJ, Taphoorn MJB, Janzer RC, Ludwin SK, Allgeier A, Fisher B, Belanger K, et al. 2009. Effects of radiotherapy with concomitant and adjuvant temozolomide versus radiotherapy alone on survival in glioblastoma in a randomised phase III study: 5-year analysis of the EORTC-NCIC trial. Lancet Oncol. 10:459–466.
- Tabu K, Muramatsu N, Mangani C, Wu M, Zhang R, Kimura T, Terashima K, Bizen N, Kimura R, Wang W, et al. 2016. A synthetic polymer scaffold reveals the self-maintenance strategies of rat glioma stem cells by organization of the advantageous Niche. Stem Cells. 34:1151–1162.
- Uto K, Inoue D, Shimuta K, Nakajo N, Sagata N. 2004. Chk1, but not Chk2, inhibits Cdc25 phosphatases by a novel common mechanism. EBBO J. 23:3386–3396.
- Wang J, Wakeman TP, Lathia JD, Hjelmeland AB, Wang XF, White RR, Rich JN, Sullenger BA. 2010. Notch promotes radioresistance of glioma stem cells. Stem Cells. 28:17–28.
- Wang XW, Zhan Q, Coursen JD, Khan MA, Kontny HU, Yu L, Hollander MC, O'Connor PM, Fornace AJ Jr, Harris CC. 1999. GADD45 induction of a G2/M cell cycle checkpoint. Proc Natl Acad Sci USA. 96:3706–3711.
- Wen PY, Kesari S. 2008. Malignant gliomas in adults. N Engl J Med. 359:492–507.
- Wu J, Lai GZ, Wan F, Xiao ZZ, Zeng LC, Wang XW, Ye F, Lei T. 2012. Knockdown of checkpoint kinase 1 is associated with the increased radiosensitivity of glioblastoma stem-like cells. Tohoku J Exp Med. 226:267–274.
- Xue J, Zong Y, Li PD, Wang LX, Li YQ, Niu YF. 2016. Low-dose hyper-radiosensitivity in human hepatocellular HepG2 cells is associated with Cdc25C-mediated G2/M cell cycle checkpoint control. Int J Radiat Biol. 92:543–547.
- Zhao YX, Cui YS, Han J, Ren JH, Wu G, Cheng J. 2012. Cell division cycle 25 homolog c effects on low-dose hyper-radiosensitivity and induced radioresistance at elevated dosage in A549 cells. J Radiat Res. 53:686–694.