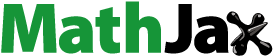
Abstract
Purpose
Uncertainties regarding the magnitude of health effects following exposure to low doses of ionizing radiation remain a matter of concern both for professionals and for the public. There is consensus within the international radiation research community that more research is required on biological effects of radiation doses below 100 mGy applied at low dose rates. Moreover, there is a demand for increasing education and training of future radiation researchers and regulators. Research, education and training is primarily carried out at universities but university-based radiation research is often hampered by limited access to radiation sources. The aim of the present report is to describe small and cost-effective low activity gamma and alpha sources that can easily be installed and used in university laboratories.
Methods and results
A gamma radiation source was made from an euxenite-(Y) rock (Y,Ca,Ce,U,Th)(Nb,Ta,Ti)2O6) that was found in an abandoned mine in Sweden. It allows exposing cells grown in culture dishes to radiation at a dose rate of 50 µGy/h and lower. Three alpha sources were custom-made and yield a dose rate of 1 mGy/h each. The construction, dosimetry and cellular effects of the sources are described.
Conclusions
We hope that the report will stimulate research and training activities in the low dose field by facilitating access to radiation sources.
Introduction
Since the 1990s, the focus of radiation protection research has been directed toward biological effects of low doses and low doses rates in an attempt to better understand the health risks associated with chronic exposure to low doses (Cho et al. Citation2019; Wojcik and Harms-Ringdahl Citation2019). Given the fact that cellular effects induced by low dose exposures are likely to be modest, it was argued that large, systems biology approaches are necessary to unravel the mechanisms and health effects of low dose exposures. The first large funding programme for low dose research was initiated by the United States Department of Energy (DOE) in 1998 and lasted for 10 years (Brooks Citation2018). In 2009, a High Level Expert Group (HLEG), consisting of experts from several European Union countries, supported by EURATOM, published a report on European low dose risk research (HLEG Citation2009). The aim of the report was to summarize the state of science and challenges in low dose research and to propose a European research strategy (Belli et al. Citation2015). The basic line of thinking was that uncertainties and confounding factors that are associated with epidemiological studies on low dose effects can only be overcome by large concerted research actions. Large projects require access to large radiation sources where many cells can be irradiated in a high throughput set up.
The recommendations of the HLEG were translated into large EU actions, starting from with the DOREMI Network of Excellence (http://www.melodi-online.eu/DoReMi/home.html), through the OPERRA project (http://www.melodi-online.eu/operra.html) and the CONCERT European Joint Programme (http://www.concert-h2020.eu). Although there is no doubt that large concerted projects including mechanistic investigations have advantages with respect to the knowledge gained, they preferentially attract nonacademic, national research entities which can contribute with their statutory funding and disfavor universities and nonacademic small-scale laboratories. This trend was augmented by the requirement, which was introduced in CONCERT, to contribute with own funding coming from a governmental source.
Indeed, from among the 36 CONCERT basic beneficiaries (termed POMs – programme owners and managers), only 4 were universities (11%) receiving 10% of the budget. The budget to the CONCERT coordinator is excluded from this calculation because it included funds for research projects which CONCERT financially supported. From among the 96 partners funded via the 9 supported projects, only 28 were universities (29%). This poses a certain problem because universities are responsible for educating the public, experts and researchers. Without financial support, the university-based research and education in the field of radiation protection will decline. Although national funding for university-based research is available in many countries, the problem should be approached at the international level. A major goal of the recently published Osaka Call for Action for co-operation in biological research to address low dose radiation risk is to enhance education about radiation and its effects among the public, students and the radiation community as a whole (Weiss and Yonekura Citation2019).
University-, and small-scale laboratory-based radiation protection research can be hampered by the limited access to appropriate radiation sources. University-based radiation sources for low dose research exist, but are rare (Manesh et al. Citation2014; Lind et al. Citation2019). Radiation sources with good dosimetry can be found in university hospitals but they are used for diagnostics and therapy. Many radiation protection authorities have radiation sources for calibrating dosimetric equipment, but access to them is limited, often not free of charge and their use requires transporting biological material outside the laboratory. Moreover, they are often not suitable for research on biological effects of low doses and low dose rates where cells need to be exposed over prolonged periods of time. What is needed to facilitate low dose radiation research at universities and small-scale laboratories are small and low-cost radiation sources that can be easily installed and maintained so that they are accessible around the clock for long-term exposure experiments.
In his book ‘small is beautiful – a study of economics as if people mattered’, E.F. Schumacher (Schumacher Citation1974) writes that ‘with industries and firms, just as with nations, there is an irresistible trend, dictated by modern technology, for units to become even bigger. We are generally told that gigantic organizations are inescapably necessary; but when we look closely we can notice that as soon as great size has been created there is often a strenuous attempt to attain smallness within bigness’. In this sense, transferred into the area of radiation research, the aim of the present report is to describe small and cost-effective low activity gamma and alpha sources that were constructed/acquired in our university laboratories. They are used to study the effects of low doses and low dose rates of ionizing radiation and we hope that the report will stimulate the acquisition and use of similar sources by other radiation researchers.
Materials and methods
Radiation sources
The sources described below can be placed inside cell incubators with the aim of studying effects of chronic irradiation. While cells exposed to gamma radiation can be grown on ordinary plastic culture dishes, cells exposed to alpha particles must be grown on Mylar foil. The gamma source is a small, natural euxenite-(Y) ore that was found in an abandoned mine in Sweden. The alpha source is 241Am which was acquired from Eckert and Ziegler, Berlin, Germany. Thanks to their low activities, neither source requires permission from the respective radiation protection authority. Details of the sources, dosimetric measurement and calculations as well as the culture dishes for alpha irradiation are described in the results section below.
Cell experiments and statistical analysis
In order to demonstrate the action of both sources, human osteosarcoma cells, U2OS, expressing a green fluorescent protein (GFP)-tagged repair protein p53-binding protein 1 (53BP1), were exposed to increasing doses of radiation and the micronucleus test was used to estimate the frequency of micronuclei as marker of DNA damage and the binucleation index as marker of cell proliferation. These cells are being used in both laboratories and are described in more detail elsewhere (Sollazzo et al. Citation2017; Sollazzo et al. Citation2018; Olofsson et al. Citation2020) . In short, the cells were cultured in Dulbecco modified Eagles medium (Sigma-Aldrich, Stockholm, Sweden and Warszawa, Poland) supplemented with 10% bovine calf serum and 1% penicillin streptomycin (Sigma-Aldrich), in a 5% CO2 humidified 37 °C incubator.
For experiments with the low dose gamma source at the Jan Kochanowski University in Kielce cells were seeded out at low density on 90 mm-diameter plastic dishes containing 10 ml of medium and stacked on the source inside an incubator for 192 hours (8 days) resulting in an averaged dose of 10.5 mGy to cells in stack position 1 and 5.9 mGy to cells in stack position 2 (see Section ‘Dosimetry’ below and for more details). Control cells were grown in the same incubator but outside the range of gamma radiation. Cells were passaged every 72 h. Cytochalasin B (Sigma-Aldrich) was added at a final concentration of 5.6 µg/ml for the final 24 h of incubation time. After 192 h cells were trypsinised, transferred to centrifuge tubes, spun down and resuspended in warm (37 °C) 0.14 M KCl (Sigma-Aldrich). After a 5 min incubation time at room temperature, the cells were centrifuged and fixed in fixative I (methanol: 0.9% NaCl: acetic acid (Sigma-Aldrich); 12:13:3) and subsequently in fixative II (methanol: acetic acid; 4:1). Following 2–3 washes with fixative II cells were dropped onto clean, dry microscopic slides (Menzel-Glaser, Germany), dried and stained with 5% Giemsa (Merck, Germany) for 10 min. Three independent experiments were performed. Blinded slides were analyzed for MN by two independent scorers and their results were pooled. The total number of scored binucleated cells was 4852 for controls, 4444 for 5.9 Gy and 3897 for 10.5 Gy. A total of 1500 nuclei per treatment group were scored for estimating the percentage of binucleated cells.
Table 2. Gamma radiation dose rates in µGy/h in cells grown in Petri dishes of varying diameter stacked on the euxenite-(Y) source.
For experiments with the low dose alpha source at the Stockholm University, U2OS cells expressing a GFP-tagged 53BP1protein were used. One day before exposure started cells were seeded on Mylar foil of alpha exposure dishes that are described below and grown for 100 h (4 days and 4 h) standing on the alpha source 1 (see description below) resulting in a dose of 110 mGy. Control cells were grown in the same incubator but outside the range of alpha radiation. Cytochalasin B (Sigma-Aldrich) was added at a final concentration of 5.6 µg/ml for the final 24 h of incubation time. Cells were harvested for MN as described above. Three independent experiments were performed. One thousand binucleated cells per treatment and experiment were scored for micronuclei on blinded slides by one scorer. Five hundred nuclei per treatment group and experiment were scored for estimating the percentage of binucleated cells.
The difference in MN frequencies and the binucleation index between the treatment groups was analyzed for significance by measuring the effect size as described in (Cohen Citation1988). The effect size thresholds were assumed as small (d value < 0.2), medium (d value 0.2–0.5), large (d value 0.5–0.8) and very large (d value > 1.3).
Results
Low dose gamma source at the Jan Kochanowski University in Kielce
The source of gamma radiation is an euxenite-(Y) mineral with a mass of 294 g that was collected in Småland, Sweden. The mineral had approximate dimensions of 10 × 4 × 4 cm. It was transported to the Jan Kochanowski University in Kielce, crushed to small pieces, pressed into fine powder and encapsulated in a round, sealed plastic capsule of 11 cm inner diameter and 1 cm height. The wall thickness of the plastic capsule is 0.2 cm. The dish was positioned inside a custom-made lead tray to shield the radiation from the bottom and sides (). The thickness of the tray walls is ca 2.5 cm and of the bottom ca 5.5 cm. The diameter of the tray is 16 cm and the height is 6.5 cm. The weight is 4.7 kg.
Figure 1. Pictures of the euxenite-(Y) source embedded in the lead tray. a: View from top. b: source positioned inside an incubator with two stacked cell culture dishes.
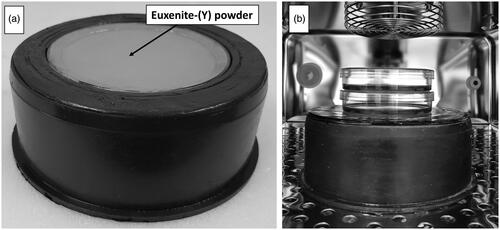
The tray with the source can be placed inside a cell incubator. Cells in culture dishes can be stacked directly on the source and exposed to gamma radiation at different dose rates.
Nuclide composition and gamma radiation spectrum
The nuclide composition and activity of the source was measured using a nitrogen cooled HPGe GX3020 – b12075 detector placed in a shielded container and connected to a Genie™ 2000 Spectroscopy Software, Canberra Industries, Inc, USA. The energy spectrum is shown in with characteristic radionuclide peaks. The largest fraction of the gamma dose comes from photons with an energy below 600 keV.
Figure 2. Energy spectrum of the euxenite-(Y) source with characteristic isotope peaks measured with a High Purity Germanium detector. The spectrum was truncated at 1750 keV. Peaks from overlapping radionuclides are labeled by lists of radionuclides.
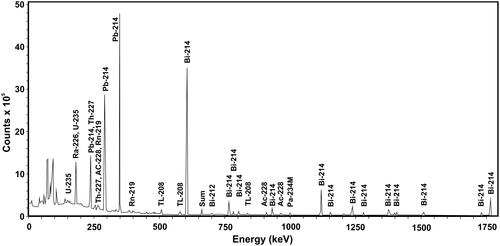
As a result of spectrum analysis, 14 radionuclides were identified with the highest contribution to the gamma emission. The radionuclides belong mainly to the uranium-radium series and showed a maintained radioactive equilibrium. Their activities are listed in . The relatively large uncertainties result from low activities of the nuclides and from interference between weak and strong peaks. Due to the problem of interference, the activity of 226Ra was estimated from the radioactive equilibrium of 214 Bi and 214Pb.
Table 1. Activities of the major radionuclides detected in the euxenite-(Y) mineral.
Dosimetry
The dosimetry of the euxenite-(Y) source was carried out in two ways. Firstly, based on the activity measurements, the dose to cells in dishes positioned on the source was calculated according to the EquationEquation (1)(1)
(1)
(1)
(1)
where D = absorbed dose; Kc = collision kerma; ΦE is the fluence of registered gamma rays with different energies; E is gamma ray energy and (µen/ρ) is the mass energy absorption coefficient (Nilsson Citation2015). The thus obtained value of D represents the dose averaged over the entire source area with a diameter of 11 cm. The uncertainty of the dose results mainly from the uncertainty of activity measurements of the four major radionuclides 234mPa, 226Ra, 214Pb and 214 Bi (). The highest uncertainty (9.1%) was observed for 214Pb. In order to take into consideration the higher uncertainties of the radionuclides with lower activity, an uncertainty level of 10% on the dose rate was assumed. Average dose values to cells growing in Petri dishes stacked on the source on top of each other were estimated based on the calculation of the changing solid angle subtended by a circular source. The results are given in marked by a hashtag, together with 10% uncertainty.
Secondly, LiF:Mg,Cu,P (MCP-N) thermoluminescence detectors (Bilski et al. Citation1994) were used to validate the calculation results and to estimate the distribution of doses across the source area. 22 MCP-N pellets were placed on the source, arranged in rings with radii of 5, 20, 30 40, 50 and 55 mm () and exposed for 37 days. The absorbed doses were determined relative to the calibration of the detectors performed on a 137Cs source and are shown for each detector in and averaged for each radius in . Due to the long-range penetrating power of gamma radiation the dose rate declines with increasing radius. The consequence of this is that cells exposed on a Petri dish of a given diameter positioned in the center of the source will receive a gradient of doses (). The doses, averaged over the dish diameter, absorbed by cells growing on Petri dishes with the most commonly used diameters of 60, 90 and 110 mm were calculated (). To this end doses from each radius were weighted by the corresponding fraction of the dish area covered by a ring described by two adjacent radii. The dose at a radius of 45 mm (corresponding to a dish of 90 mm) was not measured and was calculated as the mean from radii of 40 and 50 mm. Three dose values per ring area were derived: based on the dose measured at the inner radius, the outer radius and the mean dose. The average dose to a dish with a given diameter was calculated by weighing the inner, outer and mean doses per ring. The results are shown in , stack position 1, marked by asterisks. The values in brackets are based on the mean doses from measurements at inner and outer radii of a given ring and represent the dose uncertainty. The doses to cells in stack positions 2–6 were calculated by proportionality from the respective stack position values given in marked with a hashtag.
Figure 3. Results of TLD dosimetry of the euxenite-(Y) source. Panel a: distribution and readings (in µGy/h) of TLD dosimeters on the source. Rings symbolize radii of 5, 20, 30, 40, 50 and 55 mm. Numbers in italics above the arrow represent the calculated average dose rate for an area between two radii. Panel b: black circles represent the average dose rate measured at each radius. Error bars represent standard deviations from the individual measurements.
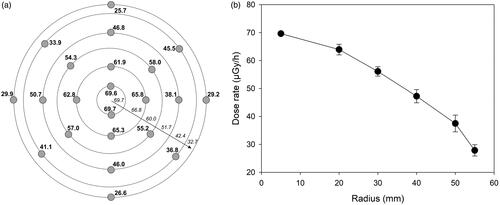
Micronuclei in U2OS cells exposed to gamma radiation
MN frequencies and percent of binucleated cells in U2OS cells that were grown on the euxenite-(Y) source for 192 h are shown in . A dose-dependent increase in MN frequency was observed. Cohen’s effect size for the difference between 0 Gy and 5.9 mGy was large (d = 0.96) and also large for the difference between 0 Gy and 10.5 (d = 0.97). The effect size between both radiation doses was small (d = 0.35). The binucleation index did not show a dose-dependent relationship.
Figure 4. MN frequencies (grey bars) and percent of binucleated cells (black dots) in U2OS cells exposed to gamma radiation from the euxenite-(Y) source for 192 h. Cells receiving 10.5 mGy were exposed in stack position 1 at 55 µGy/h and cells receiving 5.9 mGy were exposed in stack position 2 at 31 µGy/h. Asterisks mark large differences (d = 0.8–1.3, Cohen’s effect size). Error bars represent standard deviations from 3 independent experiments. BNC: binucleated cell.
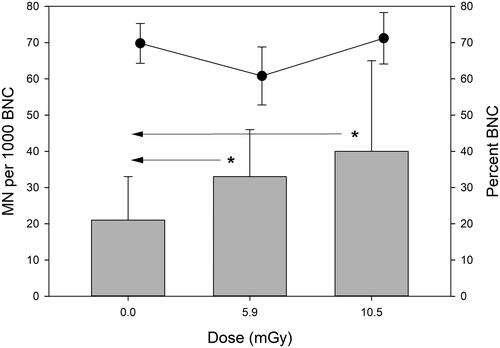
Low dose alpha sources at Stockholm University
Three identical alpha sources were purchased from Eckert & Ziegler Isotope Products GmbH, Braunschweig, Germany, product number AM1AP10005N. The sources contain 241Am, on AP1 foil (specification of the manufacturer) glued to a plexiglass circular dish with a diameter of 70 mm and protected by a gold layer. The active size of a source is 6 cm in diameter. The source activity as given by the manufacturer is 200 Bq ± 30%.
Cell culture dishes were custom-made by the STAWO company, Poland, to match the diameter of the sources. Bottomless dishes were milled from polyethylene rods and are composed of an inner ring (chamber) with an inside diameter of 60 mm and an outside ring (sleeve). The sleeve is slid over the chamber and a 2.5 µm Mylar foil so that a flat and wrinkle-free Mylar bottom is formed ().
Alpha particle energy and fluence
Alpha spectroscopy was performed in vacuum with a silicon charged particle radiation detector (Ortec, USA). Each 241Am source was placed directly on the circle shaped detector with a diameter of 20 mm. The measured energy spectra with Gaussian distributions fitted are shown in .
Since the time of measurement and consequently, the accumulated number of counts, differed between the sources, the energy spectra were rescaled as for a 1000s long measurement. Mean energy values obtained from Gaussian fits and fluence calculated for the three sources are given in . The propagation of error was used to properly determine the uncertainty of fluence. Alpha particle fluence was measured with a detector of 1 cm diameter, placed 1.7 cm from the source. The diameter of the detector was 1 cm and corrections taking into account solid angles were applied.
Table 3. Fitted mean energy values with standard deviations and fluence for the three investigated 241Am alpha sources (#1, #2 and #3).
Additionally, a series of measurements were performed using the detector with a 3 mm diameter collimator to study alpha particles emitted perpendicularly to the surface of the source. The collimator was placed in three different randomly chosen locations on the source to investigate its uniformity. Alpha particle energy and number of counts from a given location were measured and are shown in .
Table 4. Fitted mean energy values with standard deviations for the three investigated alpha sources (#1, #2 and #3) measured in three different collimator locations on the source surface.
The observed differences in alpha energy result most probably from inhomogeneity of self-absorbance of the source and thickness of the gold foil. Analysis of variance (ANOVA) using the Fisher test was used to compare the energy measurements for each source and between sources. The values were found to be in good agreement (p ≤ .05). The differences in counts are not larger than 30%, which is the uncertainty of the source activity given by the manufacturer.
Dosimetry
On their way to reach the cells, alpha particles emitted from the 241Am source lose part of their energy in the gold layer and the 2.5 µm Mylar foil. The energy loss for a given absorber was calculated with the publicly available software SRIM (Ziegler et al. Citation2010). Based on energy measurements the thickness of gold layers for each source was estimated as (1.46 ± 0.39) µm (#1), (1.62 ± 0.48) µm (#2) and (1.30 ± 0.37) µm (#3). Taking into account the Mylar foil on which the cells are grown and irradiated, the energy calculated for each source was used to estimate the dose rate. To this end, the cell layer was assumed to be 1.85 µm thick and have the density equivalent to water. The following dose rates were calculated: 1.1 mGy/h (#1), 1.4 mGy/h (#2) and 1.2 mGy/h (#3).
At the same time, it was estimated that for these sources the dose from characteristic x-ray radiation is negligibly small and equal to about 0.8 nGy/h.
Monte Carlo simulation
In order to check the homogeneity of dose across the 241Am source, the Geant4 framework (version 10.5.p01) (Agostinelli et al. Citation2003) was used to simulate the dose delivered to cells by alpha particles. The simulated experimental setup corresponded with the experimental setup as shown in . The thickness of the 241Am source assumed to be 0.4 µm with an activity of 200 Bq. The thickness of the gold layer covering the 241Am was 1.4 µm. 9 × 106 particles were simulated with energies in the range shown in . The dose rate was calculated to a water column of 2 µm above the Mylar foil. The result is shown in . The calculated dose rate was homogeneous across the source and had an average value of 3.8 mGy/h.
Figure 5. The alpha exposure setup. Panel A: polyethylene dish with Mylar foil strapped to the bottom. Panel B: A polyethylene lid with a drilled hole to allow air exchange is placed on top of the dish.
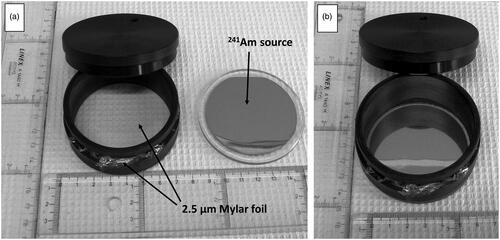
Figure 6. Characteristics of the 241Am sources. Panel a: energy spectra for the three 241Am sources. Data points represent measurements, lines represent Gaussian fits. Panel b: Graphical presentation of dose rate homogeneity of a source to cells growing on 2.5 µm Mylar calculated by Monte Carlo simulation using the Geant4 framework. Panel c: Dose rate across the source with 95% confidence intervals.
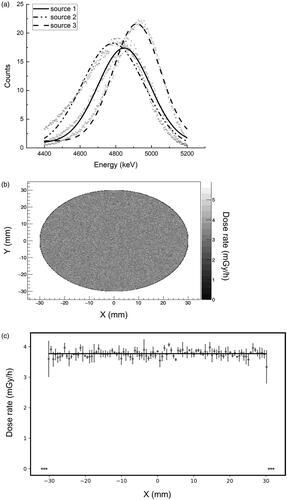
Micronuclei in U2OS cells exposed to alpha radiation
MN frequencies and percent of binucleated cells in U2OS cells that were grown on the 241Am source #1 for 100 h are shown in . The effect of a dose of 110 mGy was very large (Cohen’s effect size > 1.3). Also very large was the alpha radiation effect on cell proliferation as estimated by the binucleation index.
Figure 7. MN frequencies (grey bars) and percent of binucleated cells (black dots) in U2OS cells exposed to alpha radiation from a 241Am source for 100 h. Asterisks mark very large differences (d > 1.3, Cohen’s effect size). Error bars represent standard deviations from 3 independent experiments. BNC: binucleated cell.
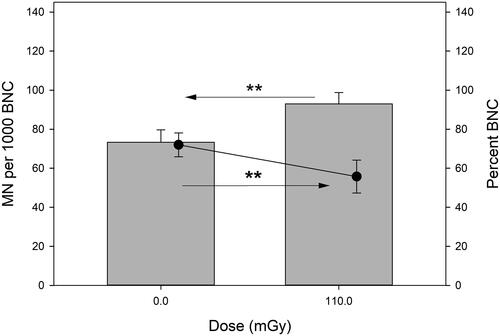
Discussion
The aim of this paper was to describe low activity gamma and alpha sources that can be used in small-scale experiments to study cellular effects of low doses and low dose rates. The sources were set up as a collaborative initiative between the universities of Stockholm (Sweden), Kielce (Poland) and Warszawa (Poland). They can be placed inside cell incubators with cells in culture dishes positioned on top of them, allowing chronic exposures. The sources have different dose rates because they were constructed to serve independent projects, rather than be used for joint investigations such as assessment of biological effectiveness.
The alpha sources are used at Stockholm University and complement a battery of exposure installations that have been constructed previously. These include a radon source (Bajinskis et al. Citation2012), a facility to expose cells to changing dose rates of X-rays (Brehwens et al. Citation2012), to mixed beams of alpha and X-rays (Staaf et al. Citation2012) and a low activity gamma radiation 137Cs source (Bajinskis et al. Citation2011; Yentrapalli et al. Citation2013). The latter 137Cs source is positioned below a cell culture incubator and the gamma dose rate at which cells are exposed can be varied by using lead shielding and by placing cell dishes on shelves mounted at different heights above the source. The low activity alpha sources can be positioned on any shelf inside the incubator allowing cells to be simultaneously exposed to a mixed beam of low dose rate alpha (dose rate 1.1–1.4 mGy/h) and gamma radiation (variable dose rates, currently 1 mGy to 25 mGy/h). The low-density plastic dishes to which the 241Am sources are glued only weakly absorb gamma radiation so do not pose a shielding problem. Although numerous high and medium activity alpha sources for external irradiation of cells have been constructed and described before (Edwards et al. Citation1980; Roos and Kellerer Citation1989; Goodhead et al. Citation1991; Neti et al. Citation2004; Esposito et al. Citation2009; Tisnek et al. Citation2009; Thompson et al. Citation2019), none of them is designed for chronic exposure of cells at dose rates in the range of mGy/h. Low dose rate alpha particle experiments with cell cultures have been described before, but with alpha-emitting radioisotopes added directly to the cell medium (Tisnek et al. Citation2009; Shi et al. Citation2016). In this respect, the sources described here may be the first of their kind to be used for chronic, external exposure of cell cultures to alpha particles.
The euxenite-(Y) source is installed at the Jan Kochanowski University in Kielce. Recently, a low energy X-ray source was constructed there for high dose rate cell exposure experiments (Czub et al. Citation2020), but a low dose rate source was missing. Thanks to its low activity and the lead shielding, the euxenite-(Y) source can be placed inside a cell incubator that is simultaneously used for culturing control, non-exposed cells or for running non-radiation experiments. It is currently used to measure cellular effects of chronic, very low dose rate exposures and for studies aiming at understanding the mechanisms of adaptive responses (Wojcik and Shadley Citation2000; Tapio and Jacob Citation2007).
Both sources were characterized for activity and energy spectrum of the emitted radiation, the dose rate and dose homogeneity across the source diameter. The dose rates of the alpha sources were calculated based on measurements of the flux and the energy of alpha particles. Monte Carlo simulation was primarily used to check the dose homogeneity across the source dimeter and was found to be constant. The obtained dose rate was somewhat higher than that based on measurements but can be explained by the assumed ideal source geometry and not considered source self-absorption of alpha particles. Ideal source characteristics are seldom met in reality which is demonstrated by the 30% uncertainty in the source activity given by the producer. The dose rate of the euxenite-(Y) source was calculated based on activity measurements and derived directly from TLD detector measurements. Both approaches gave coherent results. The TLD measurement allowed estimating the dose rate homogeneity across the source dimeter and revealed a gradual decline of the dose rate with distance from the source center. This can be explained by the loss of photons with decreasing distance from the source center, the consequence being that cells in a Petri dish positioned on the source for a given period of time absorb different doses. The dose inhomogeneity decreases with decreasing diameter of the Petri dish and is ca 20% for a dish of 60 mm and ca 40% for a dish of 90 mm. Resolving this problem would require increasing the source diameter or increasing the source thickness with increasing distance from the source center. The former solution will lead to difficulties in placing the source inside an incubator as it will take up more space and become increasingly heavy. The latter solution would require time-consuming experimentation. Although both solutions are achievable we did not think that they were necessary. The source is used to chronically expose cells to radiation in an attempt to study how cells are influenced by long-term irradiation as encountered in the environment or following incorporation of radionuclides, where the doses absorbed by cells are never homogeneous. Hence, the dosimetric uncertainty, when well defined, does not pose a problem in gaining knowledge on the biological effects of low dose and low dose rate exposure.
Apart from the dose rate inhomogeneity across the euxenite-(Y) diameter, a further factor contributing to dosimetric uncertainty is the short distance between the sources and the cell-containing Petri dishes positioned on them. Due to the inverse-square law, slight increase of the distance between the source and cells will result in a sharp dose decline. This is demonstrated by the reduction of the dose rate with the stack position. For the alpha source the distance problem is more complicated due to the Bragg peak of charged particles (Brown and Suit Citation2004). Here it is important to note that the linear energy transfer (LET) of the alpha particles is not homogeneous. We do not use collimators to eliminate alpha particles entering the cell layer at angles lower than 90° because their use would require constructing demanding, helium-filled cabinets to prevent the stopping of alpha particles by air (Roos and Kellerer Citation1989). So selecting an optimal distance between the source and cells to achieve maximum LET inside cell nuclei is not possible. However, as with the dose rate uncertainty, we do not feel that the LET inhomogeneity is a problem in view of the fact that in vivo exposure to alpha particles due to incorporation of alpha emitting radionuclides also involves mixed LET values.
The efficiency of the sources to induce biological effects was demonstrated by analyzing the frequency of micronuclei in cells exposed to two doses of gamma radiation and one dose of alpha particles. In the gamma-exposure experiments, a single exposure time was applied to cells growing on stacked Petri dishes. The difference in the absorbed dose resulted from difference in the dose rate, not time of exposure. Although small, the difference in micronucleus frequency between exposed cells could be due to both the difference in the dose and the dose rate and the interesting question is which of the contributing factors are dominant. Bringing clarity into this question requires further experiments that are easily carried out with the source at hand. The fact that doses in the range of a few mGy were able to induce a large effect is worth mentioning because, in the human peripheral blood lymphocyte micronucleus test which is regarded as sensitive, the lowest dose of acute gamma radiation that can be detected is in the range of a few hundred mGy (Wuttke et al. Citation1993; Vral et al. Citation1997). The exposure time of 192 h means that the cells must have underwent several cells divisions so the observed micronuclei may result from derived aberrations, bystander effects and genomic instability which are unlikely to play a role after acute exposure (UNSCEAR Citation2012; Burtt et al. Citation2016). Discussing the possible mechanisms is beyond the scope of this paper but the results suggest the direction of further interesting experiments that can be carried out with this type of source.
The alpha dose of 110 mGy given over a time of 100 h had a strong effect on the MN frequency in U2OS cells. In contrast to gamma radiation it also inhibited cell proliferation. We are currently using two cell lines to study the impact of chronic exposure to alpha particles, gamma radiation and mixed beams on expression of p53-controlled genes. The results will be published elsewhere.
The sources described are particularly well suited for university-based radiation research groups which are often faced with financial limitations and difficulties in accessing radiation sources. At the same time, many physics departments at universities have the know-how and instrumentation needed for characterizing the sources – a sine qua non for their meaningful application in radiation biology experiments. The sources are not very expensive: the cost of a single 241Am source was ca 4000 €. The cost of the plastic ring – ca 20€. What is also required is the 2.5 µm Mylar foil which we acquire from Goodfellow Cambridge Ltd, UK. The alpha source activity was chosen to yield a dose rate of ca 1 mGy/h. The euxenite-(Y) gamma source was found in an open-access, abandoned mineral mine in Småland, Sweden, so its acquisition was cost-free. Naturally radioactive minerals can be found on many sites worldwide (see https://en.wikipedia.org/wiki/Euxenite or https://en.wikipedia.org/wiki/Uraninite). We had no requirement for a specific dose rate of the source, so used the whole mineral and estimated the doses rate once it was ready. However, it is possible to use minerals of varying activities and masses to construct sources of varying strength, depending on the demand. At the same time it is important to note that the activities of both sources are below the values for which licensing by the radiation protection authorities in Poland and Sweden is required and it was only necessary to register them. Also, their use does not require the designation of a supervised or controlled area for radiation use.
There is an agreement among radiation protection researchers worldwide that a better understanding of radiation health effects requires more research on the biological effects of low doses and low dose rates (Salomaa et al. Citation2017; Weiss and Yonekura Citation2019). In addition, the last decades have witnessed a gradual decline in expertise both in the areas of radiation research and in academic teaching throughout Europe, and internationally. This is of particular concern given the increasing application of ionizing radiation in medicine and a possible renaissance of nuclear energy (HLEG Citation2009). Here, academic institutions have a pivotal role to play. Research at universities is often carried out by Master and Doctoral students who, if involved in radiation experiments related to the problems of low dose risk, can contribute to the process of regaining expertise in the area of radiation research and radiation risk assessment. Master projects are often of short duration and are difficult to embed in large research projects. Also, PhD projects can be composed of several small-scale projects, the results of which form the complete thesis. We believe that the small radiation sources described in the study will be particularly useful for such investigations. We hope that the ease of their acquisition will contribute to the increased attractiveness of radiation research studies at universities.
Disclosure statement
The authors report no conflicts of interest. The authors alone are responsible for the content and writing of the paper.
Additional information
Funding
Notes on contributors
Magdalena Płódowska
Magdalena Płódowska is PhD student in the field of radiation biology at the Jan Kochanowski University in Kielce. Płódowska focuses on studying cytogenetic and cellular effects in cells chronically exposed to low doses of gamma radiation.
Milagrosa Lopez-Riego
Milagrosa Lopez-Riego is PhD student in the field of radiation biology at the Stockholm University. Lopez-Riego focuses on studying genetic and cellular effects in cells chronically exposed to a combination of radiations of different qualities.
Pamela Akuwudike
Pamela Akuwudike is PhD student in the field of radiation biology at the Stockholm University. Akuwudike focuses on studying genetic and cellular effects in cells repeatedly exposed to gamma radiation.
Daniel Sobota
Daniel Sobota has a MSc in physics and is employed at the Department of Medical Physics, Jan Kochanowski University in Kielce. Sobota is an expert in dose measurements and radiological protection.
Mateusz Filipek
Mateusz Filipek is PhD Student in the field of medical physics at the Biomedical Physics Division at Faculty of Physics, University of Warsaw. Filipek focuses on studying and modeling survival curves for cells exposed to different types of ionizing radiation.
Mariusz Kłosowski
Mariusz Kłosowski is a researcher at the Institute of Nuclear Physics, Polish Academy of Sciences, Kraków. He focuses on developing and validating thermoluminescent dosimeters for radiotherapy.
Urszula Kaźmierczak
Urszula Kaźmierczak is a researcher at the Heavy Ion Laboratory, University of Warsaw. Kaźmierczak and her research interests focus on biophysical and biological effects of high LET radiation.
Beata Brzozowska
Beata Brzozowska is a researcher at the Biomedical Physics Division at Faculty of Physics, University of Warsaw and her scientific interests concern medical physics and radiobiology with special focus on modeling DNA damage and repair in cells exposed to ionizing radiation.
Agnieszka Baliga
Agnieszka Baliga has a MSc in biotechnology. Her project work focused on analysis of cellular effects of gamma radiation at very low dose rates.
Halina Lisowska
Halina Lisowska is professor of radiation biology at the Jan Kochanowski University in Kielce. Lisowska focuses on factors influencing the in vitro sensitivity of cells to ionizing radiation.
Janusz Braziewicz
Janusz Braziewicz is professor of physics at Department of Medical Physics, Jan Kochanowski University in Kielce, Braziewicz is an expert in atomic physics and X-ray fluorescence.
Paweł Olko
Paweł Olko leads the Division of Applications of Physics at the Institute of Nuclear Physics, Polish Academy of Sciences, Kraków. He is an international expert on radiation dosimetry.
Lovisa Lundholm
Lovisa Lundholm is a researcher in the field of radiation biology at the Stockholm University. Lundholm focuses on the impact of chromatin condensation on the response of cells to radiations of different qualities.
Andrzej Wojcik
Andrzej Wojcik, PhD, is professor of radiation biology at the Stockholm University and Jan Kochanowski University in Kielce. Wojcik focuses on studying the cellular effects of radiation, with special focus on combined exposure to radiations of different qualities.
References
- Agostinelli S, Allison J, Amako K, Apostolakis J, Araujo H, Arce P, Asai M, Axen D, Banerjee S, Barrand G, et al. 2003. Geant4—a simulation toolkit. Nucl Instrum Methods Phys Res Sect A. 506:250–303.
- Bajinskis A, Lindegren H, Johansson L, Harms-Ringdahl M, Forsby A. 2011. Low-dose/dose-rate γ radiation depresses neural differentiation and alters protein expression profiles in neuroblastoma SH-SY5Y cells and C17.2 neural stem cells. Radiat Res. 175:185–192.
- Bajinskis A, Olsson G, Harms-Ringdahl M. 2012. The indirect effect of radiation reduces the repair fidelity of NHEJ as verified in repair deficient CHO cell lines exposed to different radiation qualities and potassium bromate. Mutat Res. 731:125–132.
- Belli M, Tabocchini MA, Jourdain JR, Salomaa S, Repussard J. 2015. The European initiative on low-dose risk research: from the HLEG to MELODI. Radiat Prot Dosimetry. 166:178–181.
- Bilski P, Olko P, Burgkhardt B, Piesch E, Waligórski MPR. 1994. Thermoluminescence Efficiency of LiF:Mg,Cu,P (MCP-N) Detectors to Photons, Beta-Electrons, Alpha Particles and Thermal Neutrons. Radiation Protection Dosimetry. 55:31–38.
- Brehwens K, Bajinskis A, Staaf E, Haghdoost S, Cederwall B, Wojcik A. 2012. A new device to expose cells to changing dose rates of ionising radiation. Radiat Prot Dosimetry. 148:366–371.
- Brooks AL. 2018. Low dose radiation: the history of the U.S. Depeartment of Energy research program. Washington (DC): Washington State University Press.
- Brown A, Suit H. 2004. The centenary of the discovery of the Bragg peak. Radiother Oncol. 73:265–268.
- Burtt JJ, Thompson PA, Lafrenie RM. 2016. Non-targeted effects and radiation-induced carcinogenesis: a review. J Radiol Prot. 36:R23–R35.
- Cho K, Imaoka T, Klokov D, Paunesku T, Salomaa S, Birschwilks M, Bouffler S, Brooks AL, Hei TK, Iwasaki T, et al. 2019. Funding for radiation research: past, present and future. Int J Radiati Biol. 95:816–840.
- Cohen J. 1988. Statistical power analysis for the behavioral sciences. 2nd ed. Hillsdale (NJ): Lawrence Erlbaum Associates, Publishers.
- Czub J, Braziewicz J, Wasilewski A, Wysocka-Rabin A, Wolowiec P, Wojcik A. 2020. Monte Carlo dosimetry using Fluka code and experimental dosimetry with Gafchromic EBT2, XR-RV3 of self-built experimental setup for radiobiological studies with low-energy X-rays. Int J Radiat Biol. 96:718–733.
- Edwards AA, Purrott RJ, Prosser JS, Lloyd DC. 1980. The induction of chromosome aberrations in human lymphocytes by alpha-radiation. Int J Radiat Biol Relat Stud Phys Chem Med. 38:83–91.
- Esposito G, Antonelli F, Belli M, Campa A, Simone G, Sorrentino E, Tabocchini MA. 2009. An alpha-particle irradiator for radiobiological research and its implementation for bystander effect studies. Radiat Res. 172:632–642.
- Goodhead DT, Bance DA, Stretch A, Wilkinson RE. 1991. A versatile plutonium-238 irradiator for radiobiological studies with alpha-particles. Int J Radiat Biol. 59:195–210.
- HLEG. 2009. European Low Dose Risk Research. High Level and Expert Group Report. European Commission, Directorate-General for Research.
- Lind OC, Helen OD, Salbu B. 2019. The NMBU FIGARO low dose irradiation facility. Int J Radiat Biol. 95:76–81.
- Manesh SS, Deperas-Kaminska M, Fotouhi A, Sangsuwan T, Harms-Ringdahl M, Wojcik A, Haghdoost S. 2014. Mutations and chromosomal aberrations in hMTH1-transfected and non-transfected TK6 cells after exposure to low dose rates of gamma radiation. Radiat Environ Biophys. 53:417–425.
- Neti PV, de Toledo SM, Perumal V, Azzam EI, Howell RW. 2004. A multi-port low-fluence alpha-particle irradiator: fabrication, testing and benchmark radiobiological studies. Radiat Res. 161:732–738.
- Nilsson BN. 2015. Exercises with solutions in radiation physics. Berlin (Germany): Walter de Gruyter GmbH & Co KG.
- Olofsson D, Cheng L, Fernández RB, Płódowska M, Riego ML, Akuwudike P, Lisowska H, Lundholm L, Wojcik A. 2020. Biological effectiveness of very high gamma dose rate and its implication for radiological protection. Radiat Environ Biophys. 59:451–460.
- Roos H, Kellerer AM. 1989. Design criteria and performance of an alpha irradiation device for cell studies. Phys Med Biol. 34:1823–1832.
- Salomaa S, Jourdain JR, Kreuzer M, Jung T, Repussard J. 2017. Multidisciplinary European low dose initiative: an update of the MELODI program. Int J Radiat Biol. 93:1035–1039.
- Schumacher EF. 1974. Small is beautiful, a study of economics as if people mattered. London (UK): Abacus Sphere Books.
- Shi X, Seymour C, Mothersill C. 2016. The effects of chronic, low doses of Ra-226 on cultured fish and human cells. Environ Res. 148:303–309.
- Sollazzo A, Brzozowska B, Cheng L, Lundholm L, Haghdoost S, Scherthan H, Wojcik A. 2017. Alpha particles and X rays interact in inducing DNA damage in U2OS cells. Radiat Res. 188:400–411.
- Sollazzo A, Brzozowska B, Cheng L, Lundholm L, Scherthan H, Wojcik A. 2018. Live dynamics of 53BP1 foci following simultaneous induction of clustered and dispersed DNA damage in U2OS cells. Int J Mol Sci. 19:519.
- Staaf E, Brehwens K, Haghdoost S, Pachnerova-Brabcova K, Czub J, Braziewicz J, Nievaart S, Wojcik A. 2012. Characterisation of a setup for mixed beam exposures of cells to 241Am alpha particles and X-rays. Radiat Prot Dosimetry. 151:570–579.
- Tapio S, Jacob V. 2007. Radioadaptive response revisited. Radiat Environ Biophys. 46:1–12.
- Thompson JM, Elliott A, D'Abrantes S, Sawakuchi GO, Hill MA. 2019. Tracking down alpha-particles: the design, characterisation and testing of a shallow-angled alpha-particle irradiator. Radiat Prot Dosimetry. 183:264–269.
- Tisnek N, Kalanxhi E, Serkland CW, Iversen J, Belyakov OV, Dahle J. 2009. A (238)Pu irradiator for exposure of cultured cells with alpha-radiation: construction, calibration and dosimetry. Appl Radiat Isot. 67:1998–2002.
- UNSCEAR. 2012. Biological mechanisms of radiation actions at low doses. A white paper to guide the Scientific Committee’s future programme of work. Report V 12-57831. New York (NY): United Nations.
- Vral A, Thierens H, Ridder de L. 1997. In vitro micronucleus-centromere assay to detect radiation-damage induced by low doses in human lymphocytes. Int J Radiat Biol. 71:61–68.
- Weiss W, Yonekura Y. 2019. International co-operation in biological research to address low dose radiation risk: Osaka Call-for-Action. Int J Radiat Biol. 95:1351–1353.
- Wojcik A, Harms-Ringdahl M. 2019. Radiation protection biology then and now. Int J Radiat Biol. 95:841–850.
- Wojcik A, Shadley JD. 2000. The current status of the adaptive response to ionizing radiation in mammalian cells. Hum Ecol Risk Assess. 6:281–300.
- Wuttke K, Streffer C, Muller WU. 1993. Radiation induced micronuclei in subpopulations of human lymphocytes. Mutat Res. 286:181–188.
- Yentrapalli R, Azimzadeh O, Barjaktarovic Z, Sarioglu H, Wojcik A, Harms-Ringdahl M, Atkinson MJ, Haghdoost S, Tapio S. 2013. Quantitative proteomic analysis reveals induction of premature senescence in human umbilical vein endothelial cells exposed to chronic low-dose rate gamma radiation. Proteomics. 13:1096–1107.
- Ziegler JF, Biersack JP, Ziegler MD. 2010. SRIM – the stopping and range of ions in matter. Nucl Instr Meth Phys Res Sec B. 268:1818–1823.