ABSTRACT
New pest management solutions are needed to control soil invertebrates (insects, nematodes, mollusks) in order to implement the goals of the European Green Deal. Natural volatile organic compounds (VOCs) such as 1-octen-3-ol and 3-octanone, emitted by the entomopathogenic fungus Metarhizium brunneum could be part of the solution. Three major crop pests, Agriotes lineatus (wireworm), Diabrotica virgifera virgifera (corn rootworm) and Phyllopertha horticola (garden chafer), were susceptible to fumigation with 1-octen-3-ol and 3-octanone. The toxicity of the VOCs was tested in soil-free arenas and in soils which differed in moisture content and porosity. The mortality rates were dependent on the interaction between the VOC treatment, dose and pest species. The insects differed in their sensitivity to these VOCs. A dose of 1.25 µl of 1-octen-3-ol applied in a closed glass tube was sufficient to kill D. v. virgifera and P. horticola in soil trials whereas 5 µl was needed to kill A. lineatus. The highest dose (20 µl) was highly toxic to all insects. Soil moisture content slightly influenced mortality rates whereas porosity had no obvious impact. The mode of action of the VOCs is unknown but the compounds are likely to cause tissue damage and loss of body fluids. This may explain the shrivelled appearance of corn rootworm and garden chafer and melanisation in wireworm. Both 1-octen-3-ol and 3-octanone show promise as biofumigants.
Key message
Soil pests were susceptible to the biofumigants 1-octen-3-ol and 3-octanone.
Low dose fumigants could be used for soil pest management.
The soil porosity did not influence pest mortality.
Introduction
Larval stages of many major crop pests, such as wireworm, chafers, root weevils, leatherjackets and corn rootworm, cause substantial crop losses by feeding on the subterranean parts of plants and predisposing them to infection by opportunistic pathogens (Bažok et al., Citation2021; Hann et al., Citation2015; Parker, Citation2005). The combined global annual losses caused by these pests are several hundred billion dollars (Dhaliwal et al., Citation2015; Oliveira et al., Citation2014; Wan & Yang, Citation2016). The western corn rootworm (Diabrotica virgifera virgifera le Conte) alone causes losses in excess of U.S.$1 billion in the U.S.A. (Anderson et al., Citation2020; Benjamin et al., Citation2018; Gassmann, Citation2021). Currently, the control of subterranean pests is heavily dependent on the use of chemical insecticides. However, many insecticides have been withdrawn due to the risks they pose to humans, pollution of the environment, and the development of resistance in pest populations (Ntalli & Caboni, Citation2017; Van Herk & Vernon, Citation2007). Control of subterranean pests is further complicated by the fact that they are difficult to monitor; with feeding damage not evident until the above parts of the plant show stunted growth, wilting or death. Therefore, control measures must be preventive to prevent feeding damage and reduce yield (Antwi et al., Citation2017; Dedryver et al., Citation2009; Kabaluk & Ericsson, Citation2007).
Control of soil-dwelling pests can be achieved by soil fumigation as fumigants can easily diffuse in the soil and will kill a wide range of pest species and are less persistent than conventional chemical pesticides (Ajwa et al., Citation2002; Duniway, Citation2002). However, many fumigants such as methyl bromide, have been withdrawn or are restricted in their use due to their ozone-depleting properties, non-target activity, persistence and contamination of ground water (Ajwa et al., Citation2002; Oki & Giambelluca, Citation1987; Ruzo, Citation2006; Taylor, Citation1994; Weisskopf et al., Citation2021; Zasada et al., Citation2010). Currently, there are very few environmentally acceptable fumigants available for farmers. Much attention has been given to biofumigation using plants from the Brassicaceae family or Marigold (Tagetes, family Asteraceae). Brassicas produce glucosinolates that are converted into isothiocyanates, which are toxic to nematodes as well as other macro and micro soil organisms (Dutta et al., Citation2019). However, biofumigation can be costly, difficult to implement and can give variable results (Brennan et al., Citation2020; Dutta et al., Citation2019; Morris et al., Citation2020).
Recently, much attention has focused on microbial volatile organic compounds (VOCs) due to their pesticidal and semiochemical properties (Davis et al., Citation2013; Kline et al., Citation2007). Microbes produce a vast array of VOCs with different degrees of specificity and potency. Promising candidates include 1-octen-3-ol and 3-octanone which are produced by a wide range of fungi including entomopathogenic fungi such as Metarhizium anisopliae Metch. Sorokin (Bennett & Inamdar, Citation2015; Bojke et al., Citation2018). Unlike traditional fumigants which persist for many weeks, 1-octen-3-ol and 3-octanone have a significantly shorter half-life, reducing soil and atmospheric pollution. These compounds kill via fumigation a wide range of invertebrates including insects, plant-parasitic nematodes and molluscs (Cui et al., Citation2021; Herrera et al., Citation2015; Khoja et al., Citation2019, Citation2021). Besides the fumigant and killing properties of 1-octen-3-ol, these compounds also have semiochemicals properties (Xu et al., Citation2015). Kline et al. (Citation2007) showed that mosquitoes were attracted to R-1-octen-3-ol but not S-1-octen-3-ol. In contrast, both enantiomers were attractive to the grain beetles Oryzaephilus surinamensis L, Oryzaephilus mercator Fauvel (Pierce et al., Citation1989), Ahasverus advena Waltl (Pierce et al., Citation1989, Citation1991) and the tsetse flies Glossina morsitans Westwood and Glossina pallidipes Austen (Hall et al., Citation1984).
The aim of this study was to evaluate 3-octanone and 1-octen-3-ol isomers as soil fumigants, against three soil pests of global importance, namely larvae of Agriotes lineatus L., D. v. virgifera and Phyllopertha horticola L. The efficacy of the compounds was tested in different soil conditions (dry, wet, different porosity), to assess the potential integration of VOCs in integrated pest management strategies.
Materials and methods
Source and maintenance of insect pests
Field collected wireworm (A. lineatus) were kept in 1L pots filled with medium loam and provided slices of potato as food. The larvae used in this study were 14 ± 2.8 mm long and weighed 16.8 ± 8.0 grams. Wireworm were kept at 23°C until required.
Corn rootworm (Diabrotica v. virgifera) eggs, obtained from the Austrian Agency for Health and Food Safety Ltd, were incubated in a soil-sand mixture. The eggs hatched in about 17 days at 25°C but hatching could be delayed by two weeks if kept at 20°C. Larvae were provided young Maize plants as food. A mixture of first and second instar larvae being used in trials. These were handled using a round brush to avoid damaging them.
Garden chafer (Phyllopertha horticola) larvae were collected from untreated field sites in Tyrol (Austria) in late August and early September 2020. The larvae were placed in individual polypropylene containers (∅ 5 cm, height 5–10 cm) filled with soil and after a week in quarantine, healthy, disease-free individuals were selected for trials. Garden chafer werekept at 20°C until use.
Fumigation assay in absence of soil
Fumigation assays were conducted in 50 ml glass tubes (LLG, 9.400 280) against the test insects using 3-octanone, 1-octen-3-ol (racemic mixture), S-1octen-3-ol (Sigma Aldrich France) and R-1-octen-3-ol (Bedoukian Research, U.S.A.). A 1 cm2 lens cleaning tissue (VWR, France) was placed under the lid of each tube and treated with a defined dose of the VOC. The three insect species were exposed to 1.25, 2.5, 5, 10 and 20 μl corresponding to 25, 50, 100, 200 and 400 ppm, respectively of each VOC. The mortality was checked after 3, 6 and 24 h without opening the tubes. At the end of the experiment, A. lineatus were placed on clean, moist loam for five days, to see if they recovered. However, D. v. virgifera and P. horticola larvae were transferred to a Petri dish lined with moist paper and some food (maize root for D. v. virgifera and slice of carrot for P. horticola). There were ten replicates per treatment and insect. All assays were conducted in the dark at 20°C.
Fumigation assay in presence of soil
The lethality of the four VOCs wastested using three different set ups.
Soil trials at 50% humidity
Three doses (5, 10 or 20 µl) of each VOC was tested but lower doses (1.25 and 2.5 µl) of 1-octen-3-ol and its isomers were included as these were highly toxic. The VOCs were injected into 7 mm Sharrow cellulose tips (Wilsons & Co Ltd) which were placed at the bottom of 50 ml glass tubes before being covered with 10 g of loam at 50% humidity. For the control, 20 µl of water was injected into the cellulose tips. A single larva was placed on top of the soil, before capping the tube. The tubes were kept in the dark at 20°C through the trials. The mortality of the larvae was assessed after 24-, 48-, 72- and 96-hours post treatment.
The assessment method differed for each species. For example, relatively large P. horticola larvae could be observed without opening the tubes but inactive larvae were gently poked to confirm mortality. For A. lineatus, three different tubes were opened at each time point to determine if the insects were alive or dead. Individuals were returned to the tubes in less than 30 s. Due to the small size and delicate nature, destructive assessment had to be performed on D. v. virgifera. Thus, three replicate tubes were emptied at each time point and the soil carefully sifted to recover the larvae. Unlike A. lineatus, the highly fragile D. v. virgifera larvae were not monitored for delayed mortality. In the final assessment, A. lineatus and P. horticola larvae were placed on fresh soil and incubated in the dark for 5 days to see if they recovered or confirm their death. There were twelve replicates per treatment for A. lineatus or D. v. virgifera and ten replicates per treatment for P. horticola.
Soil trials at reduced moisture content
Additional trials were done in loam with a reduced moisture content (≈ 38% humidity). These trials were only done with D. v. virgifera and P. horticola, due to insufficient A. lineatus larvae being available. For this trial, the cellulose tips were injected with 1.25, 2.5, 5 or 10μl of each VOC and mortality assessed as previously described after 3, 6, 24 and 48 h. Ten repetitions were done for P. horticola and twelve for D. v. virgifera.
Soil porosity trial
The effect of soil porosity was also tested using loam mixed with 5, 10 or 20% (wt:wt) silver sand. Assays were performed as outlined above with slight modifications. Briefly, for A. lineatus and P. horticola, two doses (2.5 and 5 µl) were used for 1-octen-3-ol and its isomers, while 5 and 10 µl were used for 3-octanone. For D. v. virgifera, a single 1.25 µl dose of each VOC was tested (). These doses were chosen because they resulted in ≥50% mortality in soil at 50% humidity trials. The mortality was assessed as previously described after 24-, 48-, 72- and 96-hours. There were twelve replicates per treatment for A. lineatus and D. v. virgifera but ten for P. horticola. All soil porosity trials were done in soil at 50% humidity.
Table 1. Different doses of the VOCs 1-octen-3-ol (racemic mixture), R and S isomers of 1 -octen-3-ol and 3-octanone evaluated in soil porosity assays.
Statistical analysis
Survival data were graphically presented as Kaplan-Meier plots, using the ‘survminer’ R package. Survival regression was performed to test hypotheses on the effects of VOC treatment and dose on each species, as well as the effect of soil porosity were included, using the ‘survival’ R package. Dose was fitted as a categorical variable to accommodate nonlinear relationships. Models were initially fitted with fully interacting explanatory variables: Treatment × Dose × Species, interacting with Porosity where included. For all species, individuals that survived beyond their last observation were classed as right censored. In the cases of A. lineatus and P. horticola, this was at 96 h post treatment. For D. v. virgifera, individuals were sampled without replacement at each time point, so all live insects were classed as right censored. In addition, live D. v. virgifera individuals that were not sampled at the first time point were classed as left censored, as it was not known how long prior to observation they died. The statistical significance of explanatory variables was assessed by comparing Akaike’s Information Criterion with small-sample correction (AICc) values of nested models, using the ‘AICcmodavg’ R package. Pairwise differences between treatments were assessed using 95% confidence intervals. All statistical analyses were performed using R version 4.0.5 (R Core Team, Citation2021).
Results
Fumigation assay in absence of soil
In the soil-less trials, all D. v. virgifera, and P. horticola larvae died in less than 3 h even when exposed to the lower doses of VOCs. Larvae of A. lineatus also died in less than 3 h, except those exposed to 1.25 µl (25 ppm) 3-octanone, which were moribund but recovered 48 h after the end of the trial. Higher doses of 3-octanone killed A. lineatus in less than 3 h. VOC-killed wireworms were often melanised, with melanisation starting near the intersegmental membranes and spiracles before spreading to the rest of the body. In contrast, D. v. virgifera larvae started to shrivel after 3 h exposure to the VOCs. By 24 h, both D. v. virgifera and P. horticola had lost body fluids. Since all treated individuals were moribund or dead within 3 h and no mortality was seen in control insects, statistical analysis was not undertaken.
Fumigation assay in presence of soil
Soil trials at 50% humidity
Treatment with all four VOCs resulted in high levels of mortality in all three species, with increasing mortality and decreasing time to mortality at higher doses (). The differences in AICc values between the model including the three-way interactions between VOC treatment, dose and species (T × D × S) and the three sub-models with only two-way interactions were such that >99.9% of AICc weight was attributed to the full model (). This indicates very strong support for differences within levels of each predictor variable being highly conditional on the levels of the other predictor variables.
Figure 1. Kaplan-Meier plots of insect survival following VOC treatment in soil at 50% humidity. Observed survival is shown as stepped changes in survival probability at each time point. ‘+’ symbols indicate where individuals were censored. Fitted lines show the best fitting survival regression model predictions. Color-coding shows increasing intensity with increasing VOC dose.
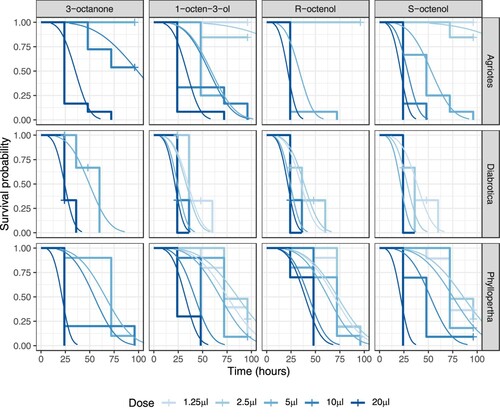
Table 2. Survival regression model comparison of nested models in trials at 50% soil humidity.
With a statistically significant three-way interaction between VOC treatment, dose, and species, findings were idiosyncratic for specific combinations. However, some general species effects can be identified, using 95% confidence intervals as the basis for discrimination between treatments (). D. v. virgifera showed higher mortality (lower survival probability) at the lowest doses than P. horticola, which in turn showed higher mortality at the lowest doses than A. lineatus. In fact, A. lineatus displayed some evidence of a threshold effect above 5 µl (100 ppm) for 1-octen-3-ol and its isomers, and 10 µl for 3-octanone, as doses below these thresholds resulted in less than 50% mortality even by the end of the trial (, top row). However, at the highest dose (20μl), all species showed 100% mortality within 72 h.
Figure 2. Mean insect survival time following VOC treatment in soil at 50% humidity. Dots represent mean predicted survival time on a natural logarithm scale, based on survival regression (). Error bars represent 95% confidence intervals. Color-coding shows increasing intensity with increasing VOC dose.
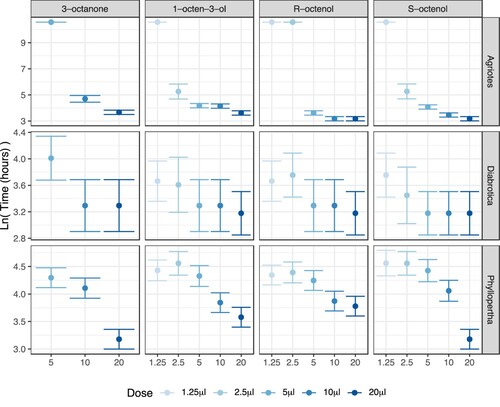
Soil trials at reduced moisture content
As with the trials at higher moisture content, treatment with all four VOCs resulted in high levels of mortality of D. v. virgifera and P. horticola, with increasing mortality and decreasing time to mortality at higher doses (). Overall, differences between VOC treatment, dose, and species were statistically significant (): as in the 50% humidity trials, >99.9% of AICc weight was attributed to the full model, indicating very strong support for the effects of each predictor variable being conditional on the other predictor variables.
Figure 3. Kaplan-Meier plots of insect survival following VOC treatment in soil at 38% humidity. Observed survival is shown as stepped changes in survival probability at each time point. ‘+’ symbols indicate where individuals were censored. Fitted lines show the best fitting survival regression model predictions. Color-coding shows increasing intensity with increasing VOC dose.
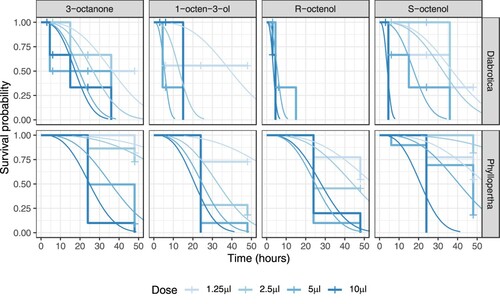
Table 3. Survival regression model comparison of nested models in trials at 38% soil humidity.
Although the 50% humidity trial and reduced moisture content trials were not directly compared statistically, having been performed separately, D. v. virgifera can be seen to have longer survival at reduced humidity, except in the case of R-octenol: in this species, the difference between VOCs is only evident at reduced humidity ( and ). In contrast, in P. horticola the interacting effects of VOC treatment and dose are very similar across both moisture content levels ( and ).
Figure 4. Mean insect survival time following VOC treatment in soil at 38% humidity. Dots represent mean predicted survival time on a natural logarithm scale, based on survival regression (). Error bars represent 95% confidence intervals. Color-coding shows increasing intensity with increasing VOC dose.
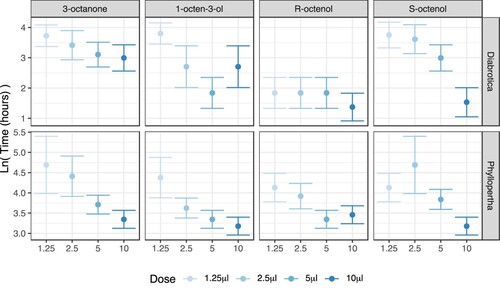
Soil porosity trial
The survival regression model that did not include porosity had substantially more statistical support than the model including porosity (, T × D × S vs. T × D × S × P), indicating that increasing the porosity of the soil had no significant impact on the efficacy of the treatments. Removing porosity as a predictor variable reduced the experimental design and analysis to the interaction between VOC treatment, dose, and species (T × D × S). Thus, porosity was not further analysed as this was assessed in the trial reported above.
Table 4. Survival regression model comparison of nested models, focusing on the effect of Porosity on survival.
In the current study, VOCs were applied in a liquid form and quickly volatilised. Although the dispersion profile of the volatile in soil was not possible to determine, it is clear that the compounds diffused freely through the soil profile for test insects to receive a lethal dose.
Discussion
The VOCs 3-octanone and 1-octen-3-ol, including its isomers, are highly toxic in a closed soil-free environment to subterranean crop pests, killing quickly even at the lowest doses. In the presence of soil, the VOCs are also highly potent, but the efficacy is dependent on the volatile compound, dose, soil moisture content and pest species but not soil porosity. It is well documented that the dispersion of fumigants in the soil is affected by a range of edaphic factors such as texture, pH, organic matter content, moisture content, cation exchange capacity, and microbial activities (Munneckel & Gundy, Citation1979; Ruzo, Citation2006). Soil porosity is known to influence the speed of dispersal and persistence (Wang et al., Citation2021), however, this was not observed in the case of 1-octen-3-ol or 3-octanone, presumably due to the system being closed. In open systems, these VOCS appeared to diffuse more quickly in porous sandy soils (unpublished observations).
Even though no significant differences were found between the fumigants activity of 1-octen-3-ol and 3-octanone, previous studies showed that garden snail (Cornu aspersum Müller), grey field slugs (Derocerus reticulatum Müller), root-knot nematode (Meloidogyne hapla Chitwood) (Khoja et al., Citation2019, Citation2021), and maize weevil (Sitophilus zeamais Motschulsky) (Herrera et al., Citation2015) were more susceptible to 1-octen-3-ol than 3-octanone. In contrast, Hummadi et al. (Citation2021) found that 3-octanone was more toxic to larvae of waxmoth (Galleria mellonella L.), chestnut weevil (Curcilio elephas Gyllenhaal), and chestnut tortrix (Cydia splendana Hübner), especially at the higher doses tested. Interestingly, at lower doses both VOCs anaesthetised these insects (Hummadi et al., Citation2021), similar observation were made with A. lineatus exposed to 1.25 µl (25 ppm) 3-octanone during the soil-less trial. Altogether these observations suggest differential sensitivity of pests exposed to VOCs. Differential sensitivity in pest species has also been reported for other fumigants such as ozone and ethanedinitrile (Işikber & Öztekin, Citation2009; Ramadan et al., Citation2020). The similar activity of racemic 1-octen-3-ol and R- or S-1-octen-3-ol will benefit further research or the industry as the cost of racemic 1-octen-3-ol is significantly lower compared to the cost of the purified enantiomers.
Very little is known about the mode of action of VOCs on subterranean insects. The exact mode of action of the VOCs was out of the scope of our study. However, the melanisation around the spiracles and intersegmental membranes of A. lineatus suggests these could be routes of entry. Melanisation is a major defense response of insects triggered by injury or stress (Butt et al., Citation2016). The fact that the other test insects appeared shrivelled suggest that some damage was done to tissues but why this did not trigger melanisation is unclear. Khoja et al. (Citation2019) noted that the VOCs contacting the fleshy parts of snails killed quickly but not if the compounds were applied to the shell. We postulate that hard cuticle and shell act as a stronger barrier which limit/block passive penetration of the VOCs, while these compounds diffuse easily through soft cuticle (intersegmental membranes) and via spiracles.
Even though fumigants diffuse 10,000–30,000 times better through the soil air space than through the water phase (Goring, Citation1962; Hartley & Audus, Citation1964; Lembright, Citation1990), soil moisture may be needed for the penetration and spreading of some classes of fumigants. In fact, nematodes absorb VOCs through water (Khoja et al., Citation2021), and in the case of fumigation with Brassicaceae, water is needed to hydrolyse the glucosinolates into isothiocyanates (Mohamed et al., Citation2020). Further studies should look at the mode of actions of the VOCs and how they are absorbed by the insects. To promote a deleterious effect on a pest, the VOCs need to penetrate the tissues to provoke damage (Khoja et al., Citation2019) and understanding how VOCs penetrate the tissues will help developing fumigation strategies.
The susceptibility of different insects, molluscs and nematodes in a closed environment to the VOCs indicate that they could be potent tools for pest management; however, further studies will be done to assess the efficacy of the VOCs in open containers to avoid saturation of the environment with VOCs. These more realistic tests will be needed to understand how the VOCs could be used in the field for pest management. It is possible that blend of 1-octen-3-ol and 3-octanone will be needed to be the most efficient against multiple species; however, the VOCs might also be toxic to beneficial arthropods and earthworms. Moreover, VOCs are also known to have attractant or repellent properties (Hummadi et al., Citation2021; Khoja et al., Citation2021; Kline et al., Citation2007; Xu et al., Citation2015). Further trials will be done in open containers and small plots to confirm their toxicity and assess potential attractant or repellent effects.
Declarations
Funding: The authors thank Certis Europe and Swansea University for funding this work.
Authors’ contributions: PAB, IB, HS, MZ, AM and TMB conceived and designed research. PAB, MZ and SK conducted experiments. JB and PAB analyzed data. KW provided Diabrotica eggs. PAB, MZ, HS, JB and TMB wrote the manuscript. All authors read and approved the manuscript.
Disclosure statement
No potential conflict of interest was reported by the author(s).
References
- Ajwa, H. A., Trout, T., Mueller, J., Wilhelm, S., Nelson, S. D., Soppe, R., & Shatley, D. (2002). Application of alternative fumigants through drip irrigation systems. Phytopathology®, 92(12), 1349–1355. https://doi.org/10.1094/PHYTO.2002.92.12.1349
- Anderson, J. A., Mickelson, J., Challender, M., Moellring, E., Sult, T., TeRonde, S., Walker, C., Wang, Y., & Maxwell, C. A. (2020). Agronomic and compositional assessment of genetically modified DP23211 maize for corn rootworm control. GM Crops & Food, 11(4), 206–214. https://doi.org/10.1080/21645698.2020.1770556
- Antwi, F. B., Shrestha, G., Reddy, G. V. P., & Jaronski, S. T. (2017). Entomopathogens in conjunction with imidacloprid could be used to manage wireworms (Coleoptera: Elateridae) on spring wheat. The Canadian Entomologist, 150(1), 124–139. https://doi.org/10.4039/tce.2017.58
- Bažok, R., Lemić, D., Chiarini, F., & Furlan, L. (2021). Western corn rootworm (Diabrotica virgifera virgifera LeConte) in Europe : Current status and sustainable pest management. Insects, 12(195), 195–126. https://doi.org/10.3390/insects12030195
- Benjamin, E. O., Grabenweger, G., Strasser, H., & Wesseler, J. H. (2018). The environmental and economic benefits of biological control of western corn rootworm Diabrotica virgifera virgifera and wireworms Agriotes spp. In maize and potatoes for selected European countries. Journal of Plant Diseases and Protection, 125, 273–285. https://doi.org/10.1007/s41348-018-0156-6
- Bennett, J. W., & Inamdar, A. A. (2015). Are some fungal volatile organic compounds (VOCs) mycotoxins? Toxins, 7(9), 3785–3804. https://doi.org/10.3390/toxins7093785
- Bojke, A., Tkaczuk, C., Stepnowski, P., & Gołębiowski, M. (2018). Comparison of volatile compounds released by entomopathogenic fungi. Microbiological Research, 214, 129–136. https://doi.org/10.1016/j.micres.2018.06.011
- Brennan, R. J. B., Glaze-Corcoran, S., Wick, R., & Hashemi, M. (2020). Biofumigation: An alternative strategy for the control of plant parasitic nematodes. Journal of Integrative Agriculture, 19(7), 1680–1690. https://doi.org/10.1016/S2095-3119(19)62817-0
- Butt, T. M., Coates, C. J., Dubovskiy, I. M., & Ratcliffe, N. A. (2016). Entomopathogenic fungi : New insights into host - pathogen interactions. In Advances in Genetics, 94, 1–58. https://doi.org/10.1016/bs.adgen.2016.01.006
- Cui, K., Zhang, L., He, L., Zhang, Z., Zhang, T., Mu, W., Lin, J., & Liu, F. (2021). Toxicological effects of the fungal volatile compound 1-octen-3-ol against the red flour beetle, Tribolium castaneum (Herbst). Ecotoxicology and Environmental Safety, 208, 111597–111599. https://doi.org/10.1016/j.ecoenv.2020.111597
- Davis, T. S., Crippen, T. L., Hofstetter, R. W., & Tomberlin, J. K. (2013). Microbial volatile emissions as insect semiochemicals. Journal of Chemical Ecology, 39(7), 840–859. https://doi.org/10.1007/s10886-013-0306-z
- Dedryver, C.-A., Robin, N., Taupin, P., & Thibord, J.-B. (2009). Lutte contre les taupins : Etat des recherches et des connaissances techniques en France et dans l’U.E.
- Dhaliwal, G. S., Jindal, V., & Mohindru, B. (2015). Crop losses due to insect pests: Global and Indian scenario. Indian Journal of Entomology, 77(2), 165. https://doi.org/10.5958/0974-8172.2015.00033.4
- Duniway, J. M. (2002). Status of chemical alternatives to methyl bromide for pre-plant fumigation of soil. Phytopathology®, 92(12), 1337–1343. https://doi.org/10.1094/PHYTO.2002.92.12.1337
- Dutta, T. K., Khan, M. R., & Phani, V. (2019). Plant-parasitic nematode management via biofumigation using brassica and non-brassica plants: Current status and future prospects. Current Plant Biology, 17, 17–32. https://doi.org/10.1016/j.cpb.2019.02.001
- Gassmann, A. J. (2021). Resistance to bt maize by western corn rootworm: Effects of pest biology, the pest–crop interaction and the agricultural landscape on resistance. Insects, 12(2), 136–116. https://doi.org/10.3390/insects12020136
- Goring, C. A. I. (1962). Theory and principles of soil fumigation. Adv. Pest Control Res, 5(1), 47–84.
- Hall, D. R., Beevor, P. S., Cork, A., Nesbitt, B. F., & Vale, G. A. (1984). 1-Octen-3-ol. A potent olfactory stimulant and attractant for tsetse isolated from cattle odours. International Journal of Tropical Insect Science, 5(05), 335–339. https://doi.org/10.1017/S1742758400008626
- Hann, P., Trska, C., Wechselberger, K. F., Eitzinger, J., & Kromp, B. (2015). Phyllopertha horticola (Coleoptera: Scarabaeidae) larvae in eastern Austrian mountainous grasslands and the associated damage risk related to soil, topography and management. SpringerPlus, 4(1), 1–15. https://doi.org/10.1186/s40064-015-0918-6
- Hartley, G. S., & Audus, L. J. (1964). Herbicide behavior in the soil. The Physiology and Biochemistry of Herbicides, 111–161.
- Herrera, J. M., Pizzolitto, R. P., Zunino, M. P., Dambolena, J. S., & Zygadlo, J. A. (2015). Effect of fungal volatile organic compounds on a fungus and an insect that damage stored maize. Journal of Stored Products Research, 62, 74–80. https://doi.org/10.1016/j.jspr.2015.04.006
- Hummadi, E. H., Dearden, A., Generalovic, T., Clunie, B., Harrott, A., Cetin, Y., Demirbek, M., Khoja, S., Eastwood, D., Dudley, E., Hazir, S., Touray, M., Ulug, D., Hazal Gulsen, S., Cimen, H., & Butt, T. (2021). Volatile organic compounds of Metarhizium brunneum influence the efficacy of entomopathogenic nematodes in insect control. Biological Control, 155, 104527–104512. https://doi.org/10.1016/j.biocontrol.2020.104527
- Işikber, A. A., & Öztekin, S. (2009). Comparison of susceptibility of two stored-product insects, Ephestia kuehniella Zeller and Tribolium confusum du Val to gaseous ozone. Journal of Stored Products Research, 45(3), 159–164. https://doi.org/10.1016/j.jspr.2008.12.003
- Kabaluk, J. T., & Ericsson, J. D. (2007). Metarhizium anisopliae seed treatment increases yield of field corn when applied for wireworm control. Agronomy Journal, 99(5), 1377–1381. https://doi.org/10.2134/agronj2007.0017N
- Khoja, S., Eltayef, K. M., Baxter, I., Bull, J. C., Loveridge, E. J., & Butt, T. (2019). Fungal volatile organic compounds show promise as potent molluscicides. Pest Management Science, 75(12), 3392–3404. https://doi.org/10.1002/ps.5578
- Khoja, S., Eltayef, K. M., Baxter, I., Myrta, A., Bull, J. C., & Butt, T. (2021). Volatiles of the entomopathogenic fungus, Metarhizium brunneum, attract and kill plant parasitic nematodes. Biological Control, 152, 104472. https://doi.org/10.1016/j.biocontrol.2020.104472
- Kline, D. L., Allan, S. A., Bernier, U. R., & Welch, C. H. (2007). Evaluation of the enantiomers of 1-octen-3-ol and 1-octyn-3-ol as attractants for mosquitoes associated with a freshwater swamp in Florida, USA. Medical and Veterinary Entomology, 21(4), 323–331. https://doi.org/10.1111/j.1365-2915.2007.00697.x
- Lembright, H. W. (1990). Soil fumigation: Principles and application technology. Journal of Nematology, 22(4S), 632–644. http://www.ncbi.nlm.nih.gov/pubmed/19287772%0Ahttp://www.pubmedcentral.nih.gov/articlerender.fcgi?artid = PMC2619113
- Mohamed, S., AbdEl-kareem, S., Massoud, S., & Abdallah, M. (2020). The role of soil moisture regime in enhancing biofumigation efficacy against Meloidogyne Incognita (Kofoid and White) Chitwood on tomato. Sinai Journal of Applied Sciences, 9(2), 143–156. https://doi.org/10.21608/sinjas.2020.88765
- Morris, E. K., Fletcher, R., & Veresoglou, S. D. (2020). Effective methods of biofumigation: A meta-analysis. Plant and Soil, 446(1), 379–392. https://doi.org/10.1007/s11104-019-04352-y
- Munneckel, D. E., & Gundy, S. D. V. (1979). Movement of fumigants in soil, dosage responses and differential effects. Annual Review of Phytopathology, 17(1), 405–429. https://doi.org/10.1146/annurev.py.17.090179.002201
- Ntalli, N., & Caboni, P. (2017). A review of isothiocyanates biofumigation activity on plant parasitic nematodes. Phytochemistry Reviews, 16(5), 827–834. https://doi.org/10.1007/s11101-017-9491-7
- Oki, D. S., & Giambelluca, T. W. (1987). DBCP, EDB, and TCP contamination of ground water in Hawaii. Ground Water, 25(6), 693–702. https://doi.org/10.1111/j.1745-6584.1987.tb02210.x
- Oliveira, C. M., Auad, A. M., Mendes, S. M., & Frizzas, M. R. (2014). Crop losses and the economic impact of insect pests on Brazilian agriculture. Crop Protection, 56, 50–54. https://doi.org/10.1016/j.cropro.2013.10.022
- Parker, W. E. (2005). Practical implementation of a wireworm management strategy – lessons from the UK potato industry. Insect Pathogens and Insect Parasitic Nematodes: IOBC Wprs Bulletin, 28(2), 87–90.
- Pierce, A. M., Pierce, H. D., Borden, J. H., & Oehlschlager, A. C. (1989). Production dynamics of Cucujolide pheromones and identification of 1-Octen-3-ol as a new aggregation pheromone for Oryzaephilus surinamensis and O. mercator (Coleoptera: Cucujidae). Environmental Entomology, 18(5), 747–755. https://doi.org/10.1093/ee/18.5.747
- Pierce, A. M., Pierce, H. D., Oehlschlager, A. C., & Borden, J. H. (1991). 1-Octen-3-ol, attractive semiochemical for foreign grain beetle, Ahasverus advena (Waltl) (Coleoptera: Cucujidae). Journal of Chemical Ecology, 17(3), 567–580. https://doi.org/10.1007/BF00982127
- Ramadan, G. R. M., Zhu, K. Y., Abdelgaleil, S. A. M., Shawir, M. S., El-Bakary, A. S., Edde, P. A., & Phillips, T. W. (2020). Ethanedinitrile as a fumigant for Lasioderma serricorne (Coleoptera: Anobiidae), and Rhyzopertha dominica (Coleoptera: Bostrichidae): toxicity and mode of action. Journal of Economic Entomology, 113(3), 1519–1527. https://doi.org/10.1093/jee/toz343
- Ruzo, L. O. (2006). Physical, chemical and environmental properties of selected chemical alternatives for the pre-plant use of methyl bromide as soil fumigant. Pest Management Science, 62(2), 99–113. https://doi.org/10.1002/ps.1135
- Taylor, R. W. D. (1994). Methyl bromide – is there any future for this noteworthy fumigant? Journal of Stored Products Research, 30(4), 253–260. https://doi.org/10.1016/S0022-474X(94)90317-4
- Team, R. C. (2021). R: A Language and environment for statistical computing. R Foundation for Statistical Computing. https://www.r-project.org/
- Van Herk, W. G., & Vernon, R. S. (2007). Soil bioassay for studying behavioral responses of wireworms (Coleoptera : Elateridae) to insecticide-treated wheat seed. Environmental Entomology, 36(6), 1441–1449. https://doi.org/10.1603/0046-225X(2007)36[1441:SBFSBR]2.0.CO;2
- Wan, F. H., & Yang, N. W. (2016). Invasion and management of agricultural alien insects in China. Annual Review of Entomology, 61(1), 77–98. https://doi.org/10.1146/annurev-ento-010715-023916
- Wang, X., Zhang, Y., Cao, A., Xu, J., Fang, W., Yan, D., Li, Y., & Wang, Q. (2021). Effects of soil type, moisture content and organic amendment rate on dimethyl disulfide distribution and persistency in soil. Environmental Pollution, 285, 117198. https://doi.org/10.1016/j.envpol.2021.117198
- Weisskopf, L., Schulz, S., & Garbeva, P. (2021). Microbial volatile organic compounds in intra-kingdom and inter-kingdom interactions. Nature Reviews Microbiology, 19(6), 391–404. https://doi.org/10.1038/s41579-020-00508-1
- Xu, P., Zhu, F., Buss, G. K., & Leal, W. S. (2015). 1-Octen-3-ol - the attractant that repels. F1000Research, 4, 156–110. https://doi.org/10.12688/f1000research.6646.1
- Zasada, I. A., Halbrendt, J. M., Kokalis-burelle, N., LaMondia, J., Mckenry, M. V., & Noling, J. W. (2010). Managing nematodes without methyl bromide. Annual Review of Phytopathology, 48(1), 311–328. https://doi.org/10.1146/annurev-phyto-073009-114425