ABSTRACT
Inorganic and organic sulphur compounds such as hydrogen sulphide (H2S) and thiols (RSH) are unwanted components in sour gas streams (e.g. biogas and refinery gases) because of their toxicity, corrosivity and bad smell. Biological treatment processes are often used to remove H2S at small and medium scales (<50 tons per day of H2S). Preliminarily research by our group focused on achieving maximum sulphur production from biological H2S oxidation in the presence of methanethiol. In this paper the underlying principles have been further studied by assessing the effect of methanethiol on the biological conversion of H2S under a wide range of redox conditions covering not only sulphur but also sulphate-producing conditions. Furthermore, our experiments were performed in an integrated system consisting of a gas absorber and a bioreactor in order to assess the effect of methanethiol on the overall gas treatment efficiency. This study shows that methanethiol inhibits the biological oxidation of H2S to sulphate by way of direct suppression of the cytochrome c oxidase activity in biomass, whereas the oxidation of H2S to sulphur was hardly affected. We estimated the kinetic parameters of biological H2S oxidation that can be used to develop a mathematical model to quantitatively describe the biodesulphurization process. Finally, it was found that methanethiol acts as a competitive inhibitor; therefore, its negative effect can be minimized by increasing the enzyme (biomass) concentration and the substrate (sulphide) concentration, which in practice means operating the biodesulphurization systems under low redox conditions.
1. Introduction
Inorganic and organic sulphur compounds such as hydrogen sulphide (H2S) and thiols (RSH) are abundant in sour gases such as landfill gas, biogas, natural gas and refinery gas.[Citation1] These compounds can be removed either by conventional physico-chemical processes or by employing (haloalkaliphilic) sulphur-oxidizing bacteria (SOB).[Citation2–5] A commonly applied process for the biological removal of H2S consists of a gas absorber, a sulphide-oxidizing bioreactor and a gravity settler for the removal of the formed sulphur particles ().[Citation6] The absorbed H2S, hereafter referred to as ‘sulphide’, is biologically oxidized to elemental sulphur (Equation (1)) and to sulphate (Equation (2)), but it can also be chemically oxidized to thiosulphate through intermediate polysulphide anions.[Citation7] Equation (3) is a simplified equation for this abiotic oxidation of sulphide.(1)
(2)
(3)
Figure 1. Simplified scheme of the process for biotechnological removal of H2S from gas streams. (A) Gas absorber; (B) bioreactor; (C) sulphur settler.
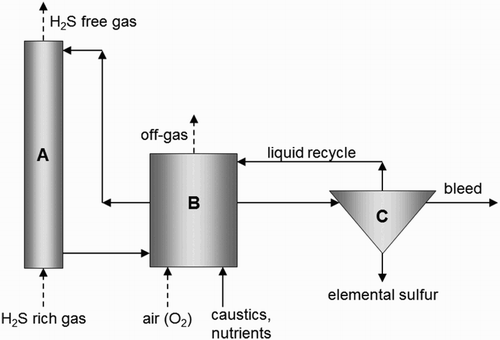
The selectivity for the biological reactions (Equations (1) and (2)) depends on the oxidation–reduction potential (ORP) of cytochromes in SOB,[Citation8] which can be controlled by the ORP of the reactor medium. The latter is primarily governed by the sulphide concentration,[Citation9] which can be regulated via the oxygen-to-sulphide (O2/H2S) supply ratio to the bioreactor. A previous study of biological sulphide removal under natron-alkaline conditions shows that, under optimal process conditions (O2/H2S = 0.6), about 83 mol% of the absorbed sulphide is biologically oxidized to sulphur.[Citation10] Less than 2 mol% is oxidized to sulphate and the remainder, about 15 mol%, is chemically converted to thiosulphate.[Citation10] Furthermore, it should be noted that at an O2/H2S supply ratio of 0.5 mol mol−1 no oxygen is available for sulphate production, while at a ratio of 2 mol mol−1 sulphate is the sole end product.[Citation11]
Sour gas streams also often contain organic sulphur compounds or thiols, which inhibit the SOB,[Citation12] leading to lower sulphide oxidation rates. Consequently toxic sulphide will accumulate which, in turn, will lead to the inhibition of reaction (1), thereby preventing the formation of hydroxyl ions that are needed to absorb H2S from the sour gas. Finally, the system will collapse. The predominant thiol in natural gas is methanethiol (CH3SH).[Citation13] Depending on the design of the absorber column, a fraction of methanethiol is absorbed in the gas washer, and then fed to the bioreactor where it is chemically oxidized to dimethyl disulphide (DMDS), according to the following reaction:(4)
Methanethiol can also chemically react with elemental biosulphur particles.[Citation14] The main reaction products are polysulphide anions and their associated methylated species, namely dimethyl polysulphanes (Equations (5) and (6)).
(5)
(6)
Promoted by nucleophilic catalysts such as HS− or CH3S−, the products of reaction 6 undergo inter-conversion reactions according to Equations (7) and (8).[Citation15](7)
(8)
Subsequently, the formed polysulphide anions are chemically oxidized to thiosulphate and sulphur according to Equation (9),[Citation16] or biologically oxidized to sulphur in a reaction analogous to Equation (1).[Citation17,Citation18](9)
Unfortunately, the number of publications describing the effect of thiols on biological desulphurization processes is limited. A preliminary study by our group focused on achieving maximum sulphur production from biological H2S oxidation in the presence of methanethiol.[Citation12] This was assured by maintaining a constant ORP value by allowing the system to decrease in O2/H2S supply ratio after methanethiol addition to the bioreactor.[Citation12] It was observed that a decrease in O2/H2S supply ratio resulted in O2-limiting conditions and hence in a significantly reduced sulphate formation rate. In order to be able to gain a better fundamental understanding of the effect of methanethiol on sulphur and sulphate formation rates, it is essential to perform experiments at a wide range of redox conditions as different enzyme systems are being used for sulphur and sulphate formation.[Citation19] Hence, in this study experiments were carried out at constant O2/H2S supply ratios within each experiment.[Citation11] Moreover, to explain our experimental results, we studied the inhibition of cytochrome c oxidase activity, the enzyme responsible for sulphate formation, by methanethiol in cell-free extracts obtained from different experiments. Finally, we also studied the inhibition mode for sulphide oxidation by estimating the unknown kinetic parameters in rate equations.
To mimic the various reactions that occur in large-scale field installations, H2S and methanethiol were added to an absorber located upstream of the bioreactor. To the best of our knowledge, such a configuration has not yet been used for experimental studies concerning the removal of H2S and thiols from gas streams.
2. Material and methods
2.1. Experimental set-up
The experimental set-up consisted of a falling-film gas absorber and a fed-batch bioreactor (). A falling-film absorber was chosen to avoid any sulphur plugging issues that did occur when we tested a packed column (unpublished results). Obviously the removal of H2S and methanethiol from the inlet gas can be simply attributed to acid–base reactions. However, the overall purpose of the process is that the consumed hydroxyl ions (OH−) in the absorber are regenerated in the bioreactor (Equations (1) and (2)). shows the dimensions and process conditions of the gas absorber. The liquid volume of the bioreactor was 2.2 L and the volume of the gas absorber was 0.2 L. The total liquid volume remained constant throughout each experimental run. Oxygen gas (99.995 vol%) was supplied to the bioreactor with the aid of a mass flow controller (type EL-FLOW, model F-201DV-AGD-33-K/E, 0–30 mL min−1, Bronkhorst, the Netherlands) to control the ORP (ORP-stat). The same type of mass flow controller was used to feed H2S (99.8 vol%, 0–17 mL min−1), CH3SH (1 vol% in N2, 0–8 mL min−1) and N2 gas (99.995 vol%, 0–350 mL min−1) to the gas absorber. pH was controlled by providing carbon dioxide (99.99 vol%) to the inlet of the gas absorber, with the use of a solenoid valve (125318, Burkert, Germany). The oxygen and carbon dioxide supply was controlled with a multiparameter transmitter (Liquiline CM442; Endress + Hauser, the Netherlands), based on real-time signals from the redox potential electrode (Ag/AgCl electrode, Orbisint CPS12D; Endress + Hauser) and a pH sensor (Orbisint CPS11D; Endress + Hauser), respectively. A gear pump (EW-74014-40, Metrohm Applikon, the Netherlands) was used to recycle the liquid between the bioreactor and the gas absorber. The gas phase was continuously recycled (34 L min−1) with a small gas compressor (N-840, KNF, Germany). The bioreactor and the gas absorber were kept at 35°C with a thermostat bath (DC10-P5/U, Haake, Germany). We collected gas samples from sampling points located at the inlet and outlet of the gas absorber and in the bioreactor (). Liquid samples came from a sampling point in the middle section of the bioreactor ().
Table 1. Dimensions and process conditions of gas absorber for H2S removal in our study.
2.1.1. Medium composition
The reactor medium included a carbonate/bicarbonate buffer including 0.051 M Na2CO3, 0.698 M NaHCO3 and 0.700 M KHCO3, hereafter referred to as ‘1.5 M [Na + + K+] buffer’. Furthermore, the medium contained 1.0 g K2HPO4, 6.0 g NaCl, 0.20 g MgCl2 × 6 H2O and 0.60 g urea, each per 1 L of Milli-Q water. A trace elements solution (1 mL L−1) was added as described elsewhere.[Citation20] The final pH of the medium was kept constant at a value of 8.5 ± 0.01 at 35°C.
2.1.2. Inoculum
The reactor was inoculated with concentrated biomass obtained by centrifugation (30 min at 15,970 × g) of a 1-L culture collected from a full-scale gas biodesulphurization installation at Industriewater Eerbeek B.V., the Netherlands.[Citation6] The dominant SOB species in this bioreactor is Thioalkalivibrio sulfidophilus.[Citation21]
2.2. Experimental design
2.2.1. Fed-batch experiments
Fed-batch experiments were performed in the set-up shown in . We conducted eleven experiments in duplicate under various ORP conditions by changing the H2S and O2 supply ratio (). However, during each experiment this ratio remained constant. Experiments 1–4 took place in the absence of CH3SH in the feed gas. In experiments 5–11, CH3SH was supplied at a constant loading rate of 0.24 mM d−1 to assess the reactor performance in the presence of methanethiol and any formed methylated sulphur species ().[Citation14] We always kept the H2S loading rate constant at a value of 36.8 mM d−1. Each experiment lasted 24 h during which we took four gas and liquid samples at regular time intervals to confirm that the reactor performance was stable.
Table 2. Overview of experimental runs at different operating conditions.
2.2.2. Cytochrome c oxidase activity measurements
The inhibitory effect of methanethiol on cytochrome c oxidase activity was assessed by measuring the oxidation rate of N,N,N′,N′-tetramethyl-p-phenylenediamine (TMPD) (Sigma-Aldrich, the Netherlands) spectrophotometrically at 610 nm (UV-1650PC, Shimadzu, Japan) as described by Sorokin et al.[Citation22] To verify the effect of biomass exposure to methanethiol, we used non-pre-exposed (a) and pre-exposed biomass (b) from a laboratory-scale bioreactor to assess cytochrome c oxidase activity. We also performed batch experiments with biomass exposed to methanethiol in the absence of any sulphide (c) to double-check the effect of methanethiol on cytochrome c oxidase.
Biomass collected from a bioreactor; not pre-exposed to methanethiol: The experimental set-up depicted in ran under conditions favourable for the expression of cytochrome c oxidase, that is, sulphate-forming conditions (ORP = −270 mV), and in the absence of methanethiol. Immediately before the start of the activity measurements, 10 µL of a methanethiol solution (sodium thiomethoxide, 95% pure, Sigma-Aldrich, the Netherlands), freshly prepared in oxygen-free Milli-Q water, was added to a sample of cell-free extract to obtain a final concentration of 0.05 mM.
Biomass collected from a bioreactor; pre-exposed to methanethiol: The reactor conditions were the same as for (a), except that the reactor was exposed to methanethiol for a period of three days (0.37 mM d−1) before the collection of biomass.
Biomass collected from a batch bottle; pre-exposed to methanethiol: Biomass was obtained from the reactor described in (a) and placed into a batch bottle, in which cells were exposed to 0.2 mM methanethiol for 18 hs at 35°C to double-check the effect of methanethiol on cytochrome c oxidase activity.
For each of these systems, the medium with biomass was centrifuged (30 min at 15,970 × g) and resuspended in 0.5 M [Na+ + K+] buffer at pH 8.5. This step was repeated two times to remove elemental sulphur particles. After that, cell-free extract was prepared by sonication of the biomass on ice. Unbroken cells and cell debris were removed by centrifugation (20,238g for 15 min). Total protein content was measured spectrophotometrically with the BCA™ Protein Assay Kit (Thermo Scientific, the Netherlands). We prepared control samples from biomass that was never exposed to methanethiol. All samples were analysed in triplicate.
2.2.3. Determination of the inhibition mode of SOB
Respiration tests were performed in order to investigate the inhibition mechanism of biological sulphide oxidation in the presence of methanethiol in an air-saturated medium ([Na+ + K+] = 1.5 M, pH = 8.5). We used a similar set-up as described elsewhere [Citation23]; it consisted of a glass mini reactor (60 mL) equipped with a magnetic stirrer. The reactor was closed with a Teflon piston to avoid any oxygen ingress. We added stock solutions containing sulphide and methanethiol to the reactor with a syringe passing through the piston. The sulphide oxidation rate was determined by measuring the oxygen consumption rate with a dissolved-oxygen sensor (Oxymax COS22D, Endress + Hauser). We calculated the biological sulphide oxidation rate as the difference between the total oxidation rate (including biological oxidation) and the abiotic oxidation rate (in the absence of biomass). We performed all experiments in triplicate at 35°C (DC10-P5/U thermostat bath, Haake, Germany).
The biomass used in these respiration tests was grown as described in Section 2.2.2(a). We centrifuged 700 mL (30 min at 15,970 × g) of biomass-containing solution and carried out a washing step after the resuspension of the pellet in 25 mL 1.5 M [Na+ + K+] buffer. The biomass concentration used in these respiration tests was always kept at 10 mg N L−1. We prepared separate solutions of sulphide and methanethiol by dissolving sodium sulphide nona-hydrate (98% pure, Sigma-Aldrich, the Netherlands) and sodium thiomethoxide (95% pure, Sigma-Aldrich, the Netherlands) in 1.5 M [Na+ + K+] buffer. Sulphide concentrations ranged from 0.05 to 0.4 mM. The biological sulphide oxidation rate was measured in the presence of 0 (absence), 0.02 and 0.05 mM methanethiol.
2.2.4. Adsorption of dimethyl polysulphanes onto the surface of biosulphur particles
We investigated the adsorption of dimethyl polysulphanes onto the surface of biosulphur particles by measuring dimethyl di-, tri- and tetrasulphide present in the headspace of the closed vials. Samples were prepared by adding a mixture of dimethyl polysulphanes (∼3 mM S) to a closed glass vial containing purified biosulphur (125 mM), suspended in 5 mL of 1.5 M [Na+ + K+] buffer. A mixture of dimethyl polysulphanes ranging from DMDS to dimethyl octasulphide was prepared as described by Rizkov et al.[Citation24] We applied the following procedure to purify the biosulphur suspension and remove any cell residue. First, the suspension was cooled to −20°C to cause cell lysis. Second, a centrifugation step (15,970g for 30 min) was applied and the supernatant was replaced with Milli-Q water to obtain a cell-free biosulphur suspension. We repeated this purification procedure four times to ensure high purity.
Each biosulphur sample was spiked with dimethyl polysulphanes and vigorously shaken for 5 min at room temperature, followed by a dimethyl polysulphanes headspace analysis. We verified that the mixing time was sufficient to reach equilibrium between the gas and the liquid phase (data not shown). After gas analysis, we concentrated the biosulphur particles by centrifugation (3260g for 10 min) and transferred them to a clean glass vial filled with 5 mL of 1.5 M [Na+ + K+] buffer. To allow desorption of any adsorbed dimethyl polysulphanes, the sample was vigorously shaken for 5 min at room temperature, followed by a second headspace analysis of the vial. We then calculated the percentage of desorbed dimethyl di-, tri- and tetrasulphide by comparing the peak areas of the compounds in the sample, that is, before and after extraction. Each sample was prepared and analysed in triplicate.
2.3. Analytical techniques
The biomass concentration was measured as the amount of organically bound nitrogen that was oxidized to nitrate by digestion with peroxodisulphate (LCK238, Hach Lange, the Netherlands). Before analysis, we centrifuged the cells twice at 20,238g for 10 min and washed the formed pellet with organic nitrogen-free medium. We did not attempt to remove any biosulphur particles, as their presence does not affect the measurements.[Citation10]
We calculated the elemental sulphur concentration by establishing the sulphur species mass balance on the basis of the sulphide, thiosulphate and sulphate analyses, assuming steady-state conditions as confirmed by the analysis of four consecutive liquid and gas samples.
We determined the sulphate and thiosulphate concentrations by ion chromatography (Metrohm Compact IC 761, Switzerland) with an anion column (Metrohm Metrosep A Supp 5, 150/4.0 mm, Switzerland) equipped with a pre-column (Metrohm Metrosep A Supp 4/5 Guard, Switzerland). The ion chromatography system included a chemical suppressor (Metrohm, Switzerland), CO2 suppressor (853, Metrohm, Switzerland) and conductivity detector (Metrohm, Switzerland). In addition, suppressors for eluent conductivity and carbon dioxide were used (Metrohm, Switzerland). The mobile phase flow rate was 0.7 mL min−1. The mobile phase consisted of 3.2 mM sodium carbonate and 1 mM sodium bicarbonate solution and 1% acetone. Before the analyses, we filtered the samples over a 0.22 μm syringe filter (Millex G5 filter unit, Merck, the Netherlands) and mixed them with 0.2 M zinc acetate in a 1:1 ratio to form ZnS, as its presence prevents abiotic sulphide oxidation. We stored the samples at 4°C.
DMDS, dimethyl trisulphide (DMTS) and polysulphides (dimethyl polysulphanes Me2S4 to Me2S8) were determined with an high-performance liquid chromatography equipped with a UV detector (Dionex UltiMate 3000RS, USA) at a wavelength of 210 nm. We separated the organic sulphur compounds with an Agilent column (Zorbax Extend-C18 1.8 µm, 2.1 × 50 mm, the Netherlands) at 20°C. The mobile phase consisted of methanol and water. The flow rate was 0.371 mL min−1 and the injection volume was 1.25 µL. The purities of the standards were above 98% for DMDS and DMTS (Sigma-Aldrich, the Netherlands).
Polysulphide anions were derivatized to dimethyl polysulphanes with methyl trifluoromethanesulphonate (≥98% pure, Sigma-Aldrich, the Netherlands), as follows:(10)
The sample preparation procedure and derivatization protocol are described elsewhere.[Citation25]
The gas phase (H2S, N2, CO2 and O2) was analysed with a gas chromatograph (Varian CP4900 Micro GC, Agilent, the Netherlands) equipped with two separate column modules, namely a 10-m-long Mol Sieve 5A PLOT (MS5) and a 10-m-long PoraPlot U (PPU). The limit of quantification was 0.1 vol% for all compounds. Both column modules were connected to a thermal conductivity detector for data acquisition. We used argon as a carrier gas with a flow rate of 1.47 ml min−1. The temperature was 80°C for the MS5 column, 65°C for the PPU column and 105°C at the injection port.
We measured the gaseous CH3SH and DMDS concentrations with a gas chromatograph (Varian CP3900 GC, Agilent, the Netherlands) equipped with an Agilent column (VF5-MS, 1 µm × 30 m × 0.25 mm). The limits of quantification were 3.6 ppm(v) and 0.2 ppm(v), respectively. The analysis was carried out with a flame ionization detector at 300°C. The initial oven temperature was 35°C. After 5 minutes, we applied a gradient of 4°C min−1 to obtain 45°C, followed by 100°C min−1 to reach 200°C, which was held for 1 min to clean the column. We used helium as the carrier gas, with a flow rate of 0.9 mL min−1. The injection volume was 1 mL.
To investigate the adsorption of dimethyl polysulphanes onto the surface of biosulphur particles, we analysed dimethyl di-, tri- and tetrasulphide concentrations in the headspace by using gas chromatography (6890N, Agilent, the Netherlands) coupled with a triple quadrupole mass spectrometer (5975, Agilent, the Netherlands), equipped with an Agilent column (HP-5MS, 30 m × 0.25 mm × 0.25 µm, Agilent, the Netherlands). Initially, the oven temperature was 50°C. After 2 min, a gradient of 12.5°C min−1 was applied to reach 200°C. We operated the mass spectrometer in SIM mode with a filament voltage of 70 eV and an electron multiplier voltage of 1200–2800 V. Helium was the carrier gas, with a flow rate of 1.3 mL min−1. The injection volume was 2.5 mL. The syringe temperature was 50°C. The equilibration time was 10 min at 40°C and the agitation speed was 250 rpm.
3. Results and discussion
3.1. Methanethiol removal from sour gas
The gas absorber column () was fed with a mixed-gas stream containing nitrogen gas as a carrier supplemented with H2S (0.9 vol%) and methanethiol (0.01 vol%). These sulphur compounds were continuously removed by dissolution into the alkaline washing solution. The removal efficiency of H2S and methanethiol in the gas absorber was around 99.8% and 70%, respectively.
Subsequently, the liquid stream loaded with HS− and CH3S− entered the base of the reactor where HS− was biologically oxidized to sulphur and sulphate (Equations (1) and (2)); also, some sulphide was chemically oxidized to thiosulphate (Equation (3)). Methanethiol was abiotically oxidized to dimethyl polysulphanes (Equations (4)–(6)). The bioreactor suspension was continuously recycled through the gas absorber where some DMDS was stripped off. The DMDS concentration in the outlet of the gas absorber was almost constant at 7 ± 1 ppm(v) at ORP values between −410 and −250 mV. For these ORP conditions, the methanethiol concentration was also almost constant at about 18.5 ± 2.2 ppm(v). However, at ORP values below −450 mV, the concentration of methanethiol at the absorber outlet doubled to 31.3 ± 3.8 ppm(v), whereas no more DMDS was detected in the off-gas. Apparently, under these conditions insufficient oxygen was available in the bioreactor for the chemical oxidation of methanethiol to DMDS (Equation (4)).
When we compared the methanethiol and DMDS concentration in gas samples from the inlet and outlet of the gas absorber, we found a gap of 40–60 mol% in the sulphur balance (absorber in–absorber out). There is no evidence that Tv. sulfidophilus, the dominant SOB species in the reactor,[Citation21] is able to oxidize methanethiol or DMDS. Based on earlier studies, we have strong indications that the dimethyl polysulphanes (resulting from Equations (5) and (6)) were adsorbing onto sulphur particles.[Citation12] To validate our hypothesis, we added dimethyl polysulphanes to a biosulphur suspension in a closed vial. Thereafter, we analysed the headspace for dimethyl di-, tri- and tetrasulphide. We then removed the biosulphur particles by centrifugation, mixed them with fresh 1.5 M [Na+ + K+] buffer in a new vial and carried out a second headspace analysis. We found dimethyl di-, tri- and tetrasulphide present at 56, 32 and 5 vol%, respectively. The percentages of dimethyl di-, tri- and tetrasulphide released during the washing process indicate that the strength of the adsorption of the dimethyl polysulphanes onto the biosulphur particles increased with increasing number of sulphur atoms in the dimethyl polysulphide chain. The fact that longer chain dimethyl polysulphanes are more hydrophobic may explain this higher affinity for the somewhat hydrophobic sulphur particles.[Citation26]
Dimethyl polysulphanes with more than two sulphur atoms form from the abiotic reaction between methanethiol and biosulphur particles (Equations (5) and (6)).[Citation14] As these compounds are far less volatile than methanethiol and DMDS, no stripping occurred in the upper section of the gas absorber. Instead, we detected accumulation in the bioreactor suspension up to a total concentration of ∼16 µM S at −450 mV ((a)). Van den Bosch et al. [Citation27] studied the inhibitory effect of DMDS and DMTS on the bacterial oxidation of sulphide and found that DMTS is more toxic to SOB than DMDS. Besides dimethyl polysulphanes, also inorganic polysulphide anions were produced from the reaction between methanethiol and elemental sulphur (Equation (6)), as reflected by the somewhat higher concentrations in the experiments with methanethiol ((b)). In addition, the
concentrations were higher at lower ORP values because of the increasing sulphide concentrations.[Citation10]
Figure 3. Reactor system operated at H2S = 36.8 mM d−1 and methanethiol = 0.24 mM d−1. (a) Concentration of dimethyl tetrasulphide (▪) and dimethyl pentasulphide (◊) in the reactor. (b) Sum of polysulphides at different ORPs for runs with (●) and without (○) methanethiol. (c) Concentration of methanethiol (▴) (CH3SH) at different ORPs in the headspace of the reactor. (d) Concentration of DMDS (▴) at different ORPs in the headspace of the reactor. The symbol □ indicates that the concentration was below the detection limit.
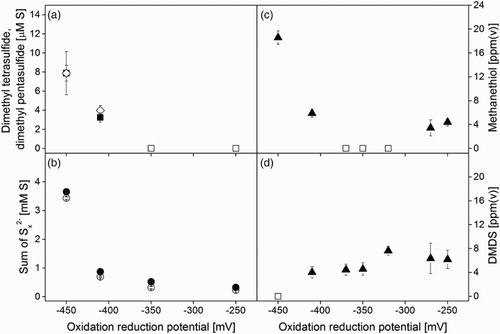
At ORP values above −410 mV, methanethiol and DMDS were continuously removed in the gas absorber, and therefore, no accumulation was observed in the headspace of the bioreactor ((c) and (d)). The DMDS concentration in the headspace of the bioreactor was around 6 ± 2 ppm(v). As this is comparable to the DMDS concentration in the absorber outlet (7 ± 1 ppm(v)) for the same ORP conditions, the gas phase and liquid phase in the gas absorber and bioreactor are in equilibrium.
Furthermore, no methanethiol was detected in the bioreactor when the system transited from sulphur to sulphate formation, that is, at ORP values between −370 and −320 mV ((c); see also Section 3.2). Most likely, the formation of sulphur particles allowed methanethiol to react away under the formation of dimethyl polysulphanes (Equations (5) and (6)) as previously observed by Van Leerdam et al.[Citation14] At an ORP of −450 mV, the methanethiol concentration in the headspace of the bioreactor suddenly increased to 20 ppm(v), whereas no more DMDS was detected ((c) and (d)). This is because insufficient oxygen was available to oxidize methanethiol to DMDS under these conditions (Equation (4)). As mentioned, we also saw this reflected in the concentrations in the gas absorber.
3.2. Effect of methanethiol on biological sulphide oxidation process
(a) and (b) show the two distinct ORP regions we found for sulphur and sulphate formation, respectively. Sulphur mainly formed below −370 mV, whereas sulphate was the main end product at ORP values above −320 mV. These findings are in good agreement with those of Klok et al. [Citation11] and Van den Bosch et al.[Citation10] In the transition zone between −370 and −320 mV (grey area in ), the selectivity for sulphur and sulphate formation was lower ((a)–(b)) than outside this area; consequently, the rate of abiotic thiosulphate formation was higher in this zone ((c)). Methanethiol had a clear effect on the selectivity for sulphate formation in the transition zone ((b)), for example, at −350 mV; as methanethiol inhibited biological sulphate formation ((b)), thiosulphate formation increased as a result of abiotic sulphide oxidation ((c)). The drop in biological sulphate formation (Equation (2)) resulted in lower oxygen requirements (Equation (2) v. Equation (3)) as can be seen in . We measured the effect of methanethiol on the oxidation of sulphide to sulphate under two different sulphate-forming conditions, namely, −250 and −350 mV. At these two ORPs, the O2/H2S consumption ratio decreased from 2.18 to 1.89 mol mol−1 and from 1.51 to 1.31 mol mol−1, respectively, after the start of methanethiol addition (). For both ORPs, this corresponds to a drop of 13 vol% in the required O2 supply rate.
Figure 4. Bioreactor performance during experiment runs with (●) and without (○) methanethiol: (a) sulphur selectivity, (b) sulphate selectivity, (c) thiosulphate selectivity. The area marked in grey is a transition zone between conditions favourable for sulphur (left) or sulphate (right) production.
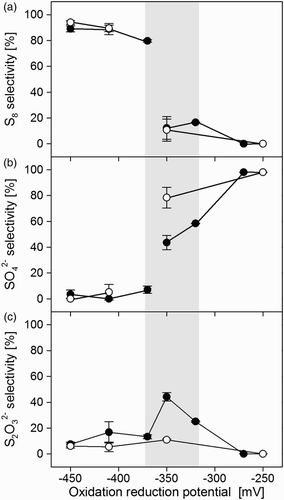
Figure 5. Molar O2/H2S supply ratio versus ORP for experimental runs with (●) and without (○;) methanethiol. The load of H2S was 36.8 mM d−1 and the load of methanethiol was 0.24 mM d−1.
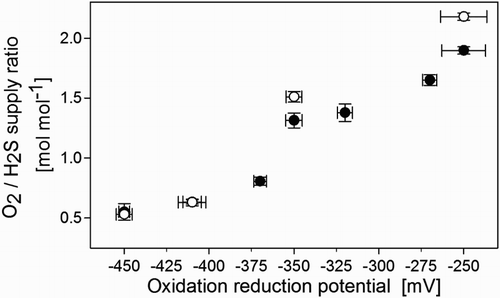
Although methanethiol clearly inhibited biological sulphate formation, the sulphur production was hardly affected ((a) and (b)). This is most likely related to the different enzymes that SOB use for the production of sulphur and sulphate, respectively. Sulphide oxidation in SOB involves several enzymes.[Citation19] In the first stage, sulphide is transformed into intracellular polysulphide. In the second stage, depending on redox conditions,[Citation8] intracellular polysulphide is either secreted out of the cell as solid biosulphur particles or further oxidized to sulphate. Since the oxidation to sulphate needs to channel six out of the eight electrons of sulphide to oxygen, high activity of the terminal part of the respiratory chain, that is, of cytochrome c oxidase, is critical for sulphate formation.[Citation8] Thiols inhibit cytochrome c oxidase by binding to its haem iron [Citation28–30] and forming a cytochrome–methanethiol complex; this inhibitory effect decreases with increasing steric hindrance of the thiols.[Citation30] As the redox state of the involved cytochromes is lowered by the binding of a thiol to cytochrome c oxidase, intracellular polysulphide oxidation to sulphate is hampered.[Citation8,Citation31] We studied the inhibitory effect of methanethiol on cytochrome c oxidase activity in more detail by measuring the oxidation rate of TMPD (an artificial electron donor for cytochrome c oxidase). shows that a strong inhibition of cytochrome c oxidase occurred in all samples that were subjected to methanethiol. It was possible to calculate the percentage of inhibition by comparing the cytochrome c oxidase activity of the control samples with that of samples exposed to methanethiol. The highest inhibition (86%) was observed for the cell-free extract spiked with methanethiol (, sample a). In this type of sample, the enzymes were not protected by a cell wall; therefore, methanethiol had easy access to cytochrome c oxidase, resulting in high inhibition. On the other hand, the cell-free extract of biomass collected from the sulphide-oxidizing reactor exposed to methanethiol (, sample b) also showed high inhibition (76%). The activity of the enzyme in a sample collected from a batch bottle exposed to methanethiol (, sample c) was least inhibited (53%). These results (samples b and c) indicate a high-binding affinity of methanethiol for the active site of cytochrome c oxidase, since even after sample preparation (sonication), strong inhibition remained.
Figure 6. Cytochrome c oxidase activity in cell-free extract of SOB obtained from: (a) a lab-scale sulphide-oxidizing bioreactor that had not yet been exposed to methanethiol but the cell-free extract was spiked with methanethiol to a final concentration of 0.05 mM. (b) A lab-scale sulphide-oxidizing bioreactor exposed to methanethiol for three days (0.37 mM d−1). (c) Batch bottles in which cells were exposed to 0.2 mM of methanethiol for 18 h. For each category, a control sample was analysed that was not exposed to methanethiol.
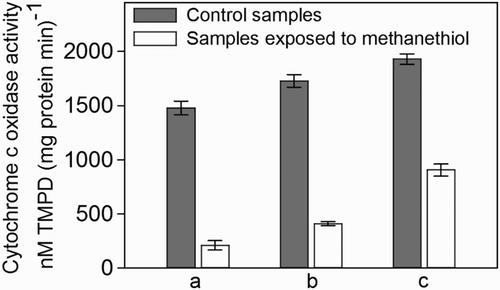
In our experiments, methanethiol never suppressed sulphate production by more than 44% ((b)), compared to ∼99% as observed by Van den Bosch et al.[Citation12] A clear difference from our experiments is that Van den Bosch et al. performed their experiments with low O2/H2S supply ratios (0.52 mol mol−1), leading to oxygen-limiting conditions. Even in the absence of methanethiol, hardly any sulphate would have been produced under such conditions.[Citation11] Moreover, the methanethiol loading in our experiments was up to seven times higher than those applied by Van den Bosch et al., that is, 240 versus 35–79 µmol L−1 d−1.
3.3. Effect of methanethiol on biomass growth
In the absence of methanethiol, growth of SOB occurred under all ORP conditions, ranging from −410 to −250 mV (). Growth was seriously hampered at −450 mV. According to the work by Klok et al.,[Citation11] SOB gain most energy for their growth from sulphate production (Equation (2)), and much less from sulphur formation (Equation (1)). As there was insufficient oxygen for sulphate production (Equation (2)), very little growth was possible at −450 mV.
Figure 7. Biomass accumulation at different molar O2/H2S supply ratios, with (●) and without (○;) methanethiol.
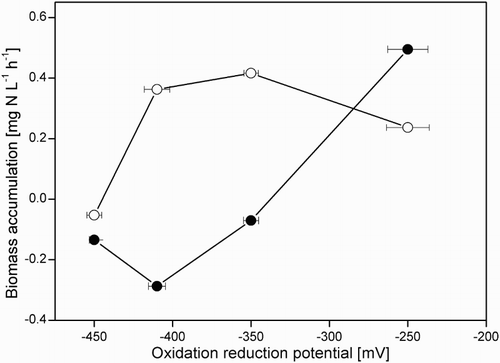
When methanethiol was supplied to the system, it had a significant effect on biomass growth (); growth was arrested at all ORP values, except at the highest ORP (−250 mV). Also at −250 mV, the sulphate production was not affected by methanethiol. Therefore, the arrested growth of the biomass at ORP values between −450 and −350 mV can be explained by lower energy obtained from sulphate formation inhibited by methanethiol (see Section 3.2) as follows from Klok's findings.[Citation11] Limited growth can be acceptable for industrial biodesulphurization systems, provided that the biomass minimum growth rate is higher than the wash-out rate.
3.4. Inhibition type of methanethiol and kinetic parameters of biological sulphide oxidation
To identify the type of inhibition exercised by methanethiol on biological sulphide oxidation, we performed respiration experiments with 20–50 μM methanethiol. The resulting Lineweaver–Burk plots () show that the reaction rate (r) increased with increasing concentrations of sulphide (CS), indicating that the inhibitory action was somehow mitigated by the substrate. As all lines intersect at the same point on the Y-axis and the specific maximal reaction rate (rmax) does not depend on the concentration of the inhibitor, methanethiol appears to be a competitive inhibitor.[Citation32] With this type of inhibition, the Michaelis–Menten constant (KM) is greater because the CS necessary to reach rmax is higher, which could explain the mitigating effect of the substrate. Thus, the reaction rate as given by Equation (11) changes into Equation (12), where CI stands for inhibitor concentration.(11)
(12)
Figure 8. Lineweaver–Burk plot obtained from a kinetic study of biological sulphide oxidation in the absence (○) and presence of methanethiol at concentrations of 0.02 mM (●) and 0.05 mM (▴). CS is the concentration of sulphide and r is the reaction rate. Temperature was 35°C, pH was 8.5 and salinity was 1.5 M.
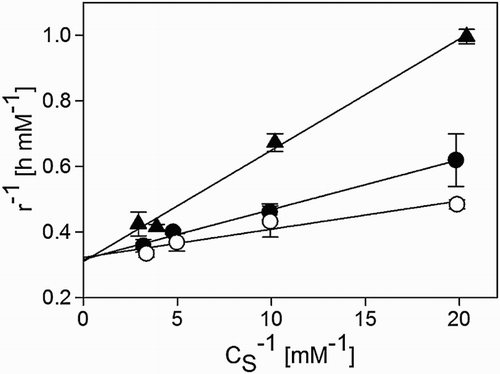
We estimated the unknown parameters in Equations (11) and (12) from respiration data, by using a non-linear least-squares method.[Citation33] We took the following stepwise approach to minimize the error. First, we estimated KM and rmax in Equation (11) to be 36 ± 3 µM and 0.324 ± 0.007 mM (mg N)−1 h−1, respectively. Second, these estimated parameters (KM and rmax) were introduced into Equation (12) to estimate the inhibition constant (ki) which was found to be 31 ± 5 µM. contains the additional uncertainty in the estimate of ki as a result of the uncertainty in rmax and KM.
Very low values of KM and ki represent high-binding affinities of sulphide and methanethiol for the involved enzymes. This low ki value also means that methanethiol is a strong inhibitor. An explanation for this could be the structural similarity between CH3S− and HS−.[Citation34] Accordingly, methanethiol would be the most toxic of all thiols.
As methanethiol appears to be a competitive inhibitor of sulphide oxidation by SOB, the stability of the biological H2S removal process under field conditions can likely be increased by increasing the biomass concentration. Obviously, a greater biomass concentration would lead to a greater total cytochrome oxidase concentration, hence to a lower ratio of methanethiol to cytochrome oxidase and to less biomass inhibition at constant methanethiol loading rates. Also because methanethiol is a competitive inhibitor, increasing the substrate (sulphide) concentration will lower the inhibition. In practice this can be achieved by applying lower redox value (e.g. <−390 mV) of the bioreactor suspension, which is correlated with the sulphide concentration.[Citation9]
4. Conclusions
This study shows that methanethiol inhibits the biological oxidation of sulphide to sulphate, whereas oxidation to sulphur is hardly affected. It is likely that this is caused by way of direct suppression of the cytochrome c oxidase activity in SOB.
In addition, we determined that dimethyl polysulphanes, resulting from a reaction between methanethiol and sulphur, reversibly adsorb onto biosulphur particles. This could offer an elegant way for removing those compounds from the bioreactor suspension along with the formed biosulphur particles.
Finally, our findings lead to the conclusion that the inhibitory effect of methanethiol in sour gases can be mitigated by increasing biomass concentrations and keeping redox conditions below −390 mV.
Acknowledgements
This work was carried out within the cooperation framework of Wetsus, European Centre of Excellence for Sustainable Water Technology (www.wetsus.nl). The authors also acknowledge Dr Dimitry Sorokin for discussions regarding oxidation pathways in SOB and René Veltman for performing the respiration tests.
Disclosure statement
No potential conflict of interest was reported by the authors.
Additional information
Funding
References
- Muradov N, Smith F. Thermocatalytic conversion of landfill gas and biogas to alternative transportation fuels. Energ Fuel. 2008;22:2053–2060. doi: 10.1021/ef8000532
- Gómez-Ramirez M, Zarco-Tovar K, Aburto J, et al. Microbial treatment of sulfur-contaminated industrial wastes. J Environ Sci Health, Part A. 2014;49:228–232. doi: 10.1080/10934529.2013.838926
- Li L, Han Y, Yan X, et al. H2s removal and bacterial structure along a full-scale biofilter bed packed with polyurethane foam in a landfill site. Bioresour Technol. 2013;147:52–58. doi: 10.1016/j.biortech.2013.07.143
- Arellano-Garcia L, González-Sánchez A, Van Langenhove H, et al. Removal of odorant dimethyl disulfide under alkaline and neutral conditions in biotrickling filters. Water Sci Technol. 2012;66:1641–1646. doi: 10.2166/wst.2012.365
- Badr K, Bahmani M, Jahanmiri A, et al. Biological removal of methanethiol from gas and water streams by using Thiobacillus thioparus: investigation of biodegradability and optimization of sulphur production. Environ Technol. 2014;35:1729–1735. doi: 10.1080/09593330.2014.881404
- Janssen AJH, Lens PNL, Stams AJM, et al. Application of bacteria involved in the biological sulfur cycle for paper mill effluent purification. Sci Total Environ. 2009;407:1333–1343. doi: 10.1016/j.scitotenv.2008.09.054
- Chen KY, Morris JC. Kinetics of oxidation of aqueous sulfide by oxygen. Environ Sci Technol. 1972;6:529–537. doi: 10.1021/es60065a008
- Visser JM, Robertson LA, Van Verseveld HW, et al. Sulfur production by obligately chemolithoautotrophic thiobacillus species. Appl Environ Microbiol Am Soc Microbiol. 1997;63:2300–2305.
- Janssen AJH, Meijer S, Bontsema J, Lettinga G. Application of the redox potential for controling a sulfide oxidizing bioreactor. Biotechnol Bioeng. 1998;60:147–155. doi: 10.1002/(SICI)1097-0290(19981020)60:2<147::AID-BIT2>3.0.CO;2-N
- Van den Bosch PLF, van Beusekom OC, Buisman CJN, et al. Sulfide oxidation at halo-alkaline conditions in a fed-batch bioreactor. Biotechnol Bioeng. 2007;97:1053–1063. doi: 10.1002/bit.21326
- Klok JBM, van den Bosch PLF, Buisman CJN, et al. Pathways of sulfide oxidation by haloalkaliphilic bacteria in limited-oxygen gas lift bioreactors. Environ Sci Technol. 2012;46:7581–7586. doi: 10.1021/es301480z
- Van den Bosch PLF, Fortuny-Picornell M, Janssen AJH. Effects of methanethiol on the biological oxidation of sulfide at natron-alkaline conditions. Environ Sci Technol. 2009;43:453–459. doi: 10.1021/es801894p
- Fredericks E, Harlow G. Determination of mercaptans in sour natural gases by gas liquid chromatography and microcoulometric titration. Anal Chem. 1964;36:263–266. doi: 10.1021/ac60208a006
- Van Leerdam RC, Bosch PLF, Lens PNL, et al. Reactions between methanethiol and biologically produced sulfur. Environ Sci Technol. 2011;45:1320–1326. doi: 10.1021/es102987p
- Steudel R. The chemistry of organic polysulfanes RS (n)-R (n > 2). Chem Rev. 2002;102:3905–3946. doi: 10.1021/cr010127m
- Steudel R, Holdt G, Göbel T. Ion-pair chromatographic separation of inorganic sulphur anions including polysulphide. J Chromatogr. 1989;475:442–446. doi: 10.1016/S0021-9673(01)89701-6
- Sorokin DY, Kuenen JG. Haloalkaliphilic sulfur-oxidizing bacteria in soda lakes. FEMS Microbiol Rev. 2005;29:685–702. doi: 10.1016/j.femsre.2004.10.005
- Sorokin D, van den Bosch PLF, Abbas B, et al. Microbiological analysis of the population of extremely haloalkaliphilic sulfur-oxidizing bacteria dominating in lab-scale sulfide-removing bioreactors. Appl Microbiol Biotechnol. 2008;80:965–975. doi: 10.1007/s00253-008-1598-8
- Muyzer G, Sorokin DY, Mavromatis K, et al. Complete genome sequence of “Thioalkalivibrio sulfidophilus” HL-EbGr7. Stand Genomic Sci. 2011;4:23–35. doi: 10.4056/sigs.1483693
- Pfennig N, Lippert KD. Über das vitamin B12-bedürfnis phototropher Schwefelbakterien. Arch Microbiol. 1966;55:245–256.
- Sorokin DY, Muntyan MS, Panteleeva AN, et al. Thioalkalivibrio sulfidiphilus sp. nov., a haloalkaliphilic, sulfur-oxidizing gammaproteobacterium from alkaline habitats. Int J Syst Evol Microbiol Soc. 2012;62:1884–1889. doi: 10.1099/ijs.0.034504-0
- Sorokin DY, Lysenko AM, Mityushina LL, et al. Thioalkalimicrobium aerophilum gen. nov., sp. nov. and Thioalkalimicrobium sibericum sp. nov., and Thioalkalivibrio versutus gen. nov., sp. nov., Thioalkalivibrio nitratis sp. nov. and Thioalkalivibrio denitrificans sp. nov., novel obligately alkaliphilic and obligately chemolithoautotrophic sulfur-oxidizing bacteria from soda lakes. Int J Syst Evol Microbiol Soc. 2001;51:565–580. doi: 10.1099/00207713-51-2-565
- Kleinjan WE, Keizer Ade, Janssen AJH. Kinetics of the chemical oxidation of polysulfide anions in aqueous solution. Water Res. 2005;39:4093–4100. doi: 10.1016/j.watres.2005.08.006
- Rizkov D, Lev O, Gun J, et al. Development of in-house reference materials for determination of inorganic polysulfides in water. Accredit Qual Assur. 2004;9:399–403. doi: 10.1007/s00769-004-0788-z
- Roman P, Bijmans MF, Janssen AJH. Quantification of individual polysulfides in lab-scale and full-scale desulfurisation bioreactors. Environ Chem. 2014;11:702–708. doi: 10.1071/EN14128
- Kleinjan WE, de Keizer A. Janssen AJH. Biologically produced sulfur. Elemental Sulfur and Sulfur-Rich Compounds I. Topics in current chemistry, 2003.
- Van den Bosch PLF, de Graaff M, Fortuny-Picornell M, et al. Inhibition of microbiological sulfide oxidation by methanethiol and dimethyl polysulfides at natron-alkaline conditions. Appl Microbiol Biotechnol. 2009;83:579–587. doi: 10.1007/s00253-009-1951-6
- Hu T-M, Ho S-C. Kinetics of redox interaction between cytochrome c and thiols. J Med Sci. 2011;31:109–115.
- Tomkova A, Antalik M, Bágel'ová J, et al. Absorption and Raman spectroscopy study of cyt c-thiol complexes in acidic solutions. Gen Physiol Biophys. 1992;3:273–288.
- Wilms J, Lub J, Wever R. Reactions of mercaptans with cytochrome c oxidase and cytochrome c. Biochim Biophys Acta, Bioenerg Elsevier. 1980;589:324–335. doi: 10.1016/0005-2728(80)90048-1
- Helmann JD. Prokaryotic redox switches. In: Jakob U, Reichmann D, editors. Oxidative stress and redox regulation. Dordrecht: Springer; 2013. p. 233–276.
- Sharma R. Enzyme inhibition: mechanisms and scope, In: R. Sharma, editor. Enzyme inhibition and bioapplications. InTech; 2012. Available from: http://www.intechopen.com/books/enzyme-inhibition-and-bioapplications/enzyme-inhibition-mechanisms-and-scope
- Keesman KJ. System identification: an introduction. London: Springer, Verlag; 2011.
- Debajyoti D. Biochemistry. Kolkata: Academic Press; 2005.
APPENDIX 1
Table A1. Estimated inhibition constant () with corresponding standard deviation (σ) for specific maximal reaction rate (rmax) and Michaelis–Menten constant (KM).
Table