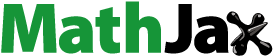
ABSTRACT
The hazardous waste penicillin fermentation residue (PR) is a huge hazard to the environment. The bio-oil produced by the pyrolysis of the penicillin fermentation residue has the potential to become a biofuel in the future. This paper studied the pyrolysis characteristics of PR at 400°C ∼700°C. According to the weight loss and weight loss rate of PR, the whole process of pyrolysis can be divided into three stages for analysis: dehydration and volatilization, initial pyrolysis, and pyrolytic char formation. The experimental results showed that the yield of the liquid phase is the highest (33.11%) at 600°C. GC-MS analysis results showed that high temperature is beneficial to reduce the generation of oxygenated hydrocarbons (73%∼31%) and the yield of nitrogenous compounds gradually increased (19%∼43%); the yield of hydrocarbons was low in 400°C∼600°C pyrolysis (2%∼5%) but significantly increased around 700°C (22%). In the temperature range of 400°C to 700°C, the proportion of C5-C13 in bio-oil gradually increased (26%-64%), and the proportion of C14-C22 gradually decreased (47%-16%). The catalyst can increase the proportion of hydrocarbons in the bio-oil component. And the Fe2O3/HZSM-5 mixed catalyst has a significant reduction effect on oxygen-containing hydrocarbons and nitrogen-containing compounds.
GRAPHICAL ABSTRACT

1. Introduction
China produces and exports a large number of antibiotics every year, up to more than 70 kinds, about 248000 tons [Citation1]. China accounts for 70 percent of the world's annual production of antibiotics, especially cephalosporins, streptomycin and penicillin [Citation2–4]. Antibiotic bacteria residue is a kind of biomass residue composed of strains, intermediate metabolites, and culture medium, which is very easy to deteriorate [Citation5]. If the antibiotic residues are not handled properly, they can cause various environmental problems, such as unpleasant odours, water pollution, and drug resistance of animals and plants [Citation6,Citation7]. In 2008, antibiotic residues were included in China's National Hazardous Waste List, which stipulates that antibiotic residues are treated as hazardous waste [Citation8]. At the same time, it encourages the research and development of antimicrobial residue reduction, harmlessness, and resource utilization technologies. In the United States, the European Union, and other countries, incineration is used to dispose of solid waste generated by the pharmaceutical industry. This method can quickly and greatly reduce the volume of antibiotic residues, achieving a reduction of more than 95%. Some large pharmaceutical companies in China (such as Huayao Group and CSPC) have also built incineration and disposal devices for antibiotic bacteria residues. However, the moisture content of antibiotic bacteria residue is as high as 80%, and the calorific value is low.
A large amount of fuel needs to be added during the incineration process, which leads to higher operating energy consumption and cost. In addition, the incineration of antibiotic residues must strictly implement the ‘Hazardous Waste Incineration Pollution Control Standard’. If the incineration is improper, it is easy to cause secondary pollution. Therefore, it is urgent and strategic to pay attention to the research on the harmlessness and resource disposal of antibiotic residues.
The pyrolysis technology can not only fully resource the organic components in the biomass to obtain pyrolysis char, but also obtain high-quality pyrolysis gas and liquid fuel through catalytic upgrading [Citation9]. Although pyrolysis technology has been proven to be an effective technology for the conventional biomass resource treatment of cellulose, lignin, hemicellulose, and coal, further research is needed in the pyrolysis and disposal of antibiotic residues [Citation10–12]. The dry basis of antibiotic bacteria residue has a high content of organic matter. Compared with biomass such as cellulose and lignin, it has more nutrients and bacterial metabolites and is a biomass raw material with great potential [Citation13]. If the antibiotic residue is converted into bio oil by pyrolysis, not only the reduction and harmless disposal of antibiotic residue can be solved, but also the resource disposal of antibiotic residue can be realized. However, the reaction mechanism of this transformation process still needs to be improved, and further research is needed to lay the foundation for the industrial application of bio-oil prepared by pyrolysis of antibiotic bacteria residue.
In recent years, the pyrolysis technology of antibiotic residues has received widespread attention. Many studies have found that pyrolysis temperature and heating rate are important factors affecting the distribution of pyrolytic products of antibiotic residue [Citation14,Citation15]. It not only affects the yields of gas, liquid and solid phases, but also affects the contents of nitrogen, oxygen and phosphorus in the products [Citation16,Citation17]. In addition, adding catalysts to the pyrolysis process is the main method of refining bio-oil. According to the characteristics of pyrolysis process and different biomass resources, choosing the appropriate catalyst can improve the pyrolysis efficiency of biomass [Citation18,Citation19]. Catalysts used in biomass pyrolysis mainly include alkali metal and alkaline earth metal catalysts, metal oxide catalysts, and molecular sieve catalysts [Citation20–22]. In the catalytic pyrolysis of γ-valerolactone (GVL) into aromatic hydrocarbons by MCM-41, β zeolite, ZSM-5, and HZSM-5 zeolite, it was found that HZSM-5 (Si/Al = 25) was the most effective catalyst for reactivity and selectivity [Citation23,Citation24]. In addition, HZSM-5 catalyst not only reduces the molecular weight distribution of pyrolysis oil, but also reduces the oxygen content of bio oil [Citation25]. In the study of biomass catalytic pyrolysis, the composite catalyst prepared by metal or metal oxides on molecular sieves has a better catalytic effect. Oxide catalysts usually include alkaline earth metal oxides and transition metal oxides, such as ZnO, CaO, MgO, Al2O3, and Fe2O3, etc [Citation26–29]. In the pyrolysis experiment, it was found that the use of metal oxide modified HZSM-5 zeolite can not only increase the proportion of aromatics and olefins, but also affect the molecular weight of pyrolysis oil and improve the catalytic efficiency [Citation27,Citation30,Citation31].
This study aims to analyze the effect of metal-modified HZSM-5 molecular sieve on the liquid yield and composition during PR pyrolysis. GC-MS was used to analyze the bio-oil under different experimental parameters, and the proportion of different types of compounds in the pyrolysis oil was further analyzed to provide a basis for bio-oil as a new energy source in the future.
2. Materials and methods
2.1. Experimental materials
2.1.1. Materials
PR was obtained from Hua bei Pharmaceutical Group, Shijiazhuang City, Hebei Province. After drying, grinding, and sieving, the PR is obtained as a dark yellow powder, which is stored in a glass desiccator for later use. Proximate analysis (wt%), ultimate analysis (wt%), and chemical composition (wt%) of PR powder are shown in . Refer to GB 5009.5-2010, GB/T 5009.6-2003, and NY/T 1676–2008 to measure protein (Kjeldahl method), oil (extraction method), and polysaccharide content (phenol-sulfuric acid). The elemental contents of the PR were determined by C, H, N, S, O elemental analyzer (Vario Macro Cube, Elementar, Germany). There are a lot of organic substances in PR, such as crude protein (45.4%), crude fat (3.85%), and carbohydrate (1.66%). Therefore, the volatile content in the proximate analysis was the highest (78.53%), and the content of C (44.88%) and O (34.12%) was higher in the ultimate analysis. Also, the content of N in penicillin fermentation residue was high (5.93%), which affected the utilization of organic matter.
Table 1. Properties of PR powder.
2.1.2. Catalysts preparation
The catalyst is a mixed catalyst that supports metal ions on HZSM-5. HZSM-5 molecular sieve was purchased from Nanjing Jicang Nanotechnology Co., Ltd. The carrier HZSM-5 molecular sieve is a polyhedron with a flat side structure. The molecular sieve contains Si, Al, and O elements, and a large number of holes are uniformly distributed on the surface. SEM and Mapping characterization of the HZSM-5 are shown in Fig.S1. The preparation methods of the five mixed catalysts(CuO/HZSM-5, Ag/HZSM-5, Fe2O3/HZSM-5, ZnO/HZSM-5, ZrO2/HZSM-5) were impregnation of HZSM-5 in metal compound solution (metal accounted for 10 wt%), drying and calcination. Taking the preparation of CuO / HZSM-5 as an example, dissolve 4.097 g Cu (NO3)2·3H2O in 10 g deionized water to prepare a solution, and then the solution was evenly dropped into a 10 g HZSM-5 molecular sieve. After ultrasonic homogenization (ultrasonic 120 min), it was dried (105°C, 12 h) and ground into powder. Finally, the mixed catalyst is calcined for 5 h and sealed for later use(10 °C / min heating to 550 °C and holding for 5 h).
2.2. Experimental
2.2.1. Experimental setup
Tube furnace produced by Hefei kejing Material Technology Co., Ltd. The pyrolysis experimental device is shown in .The experimental device mainly consists of a carrier gas source, flowmeter, temperature control device, vertical tubular furnace, condensing device, and collecting device. The units of the pyrolysis unit are connected with the polytetrafluoroethylene pipe. In the non- catalytic pyrolysis, 5.0 g (±0.01 g) PR was placed in a quartz basket. The pyrolysis experiment was carried out in high purity argon (>99.999%,500 ml / min) atmosphere and the device was blown for 30 min before pyrolysis., and increase the temperature at an average speed (10 °C / min) to 400, 500, 600, and 700°C for 2 h. After the reaction, the solid product was cooled to room temperature and weighed. The liquid product obtained by condensation was dried at low temperature until its mass remains the same, which is called bio-oil. To ensure full collection, the bio-oil was absorbed by acetone (AR, Tianjin Damao Chemical Co., Ltd., China) in the tank, and the PR catalyzed bio-oil was collected and concen- trated by rotary evaporation. Repeat all experiments three times to ensure the accuracy and repeatability of the results. In the catalytic pyrolysis, 5 g (± 0.01 g) of PR was placed in a quartz basket, and 2.5 (± 0.01 g)g of catalyst was placed on a 100 mesh screen near the lower gal- vanic couple., and close the furnace door, and increase the temperature at an average speed (10 °C / min) to, 600°C for 2 h. Other operations are the same as non-catalytic pyrolysis.
2.2.2. Analysis method
Yield analysis of pyrolytic products from PR. The solid and liquid products after pyrolysis were collected and weighed by electronic analytical balance (ab104-n). The product yield was calculated as follows:
(1)
(1)
(2)
(2)
(3)
(3)
Where wLi, wre, and wother are the percent yields of bio-oil, char, and others, respectively, mbio-oi, mfeedstock, and msolid-residue are the mass of bio-oi, feedstock, and solid residue, respectively.
Pyrolysis characteristics of PR. The ‘NETZSCH, STA449 F3’ thermogravimetric analyzer was used to analyze the weight loss and rate of weight loss of PR. In the thermogravimetric test, the quantity of N2 flow is 60 ml/min into the analysis furnace. During the thermogravimetric tests, a sample with the initial weight of 6 mg was heated from 30 °C to 1000 °C at three heating rates (10, 20, and50 °C/min), and then kept at 1000 ℃ for 10 min.
X-ray photoelectron spectroscopy. X-ray photoelectron spectroscopy (XPS) is an effective technique for solid surface analysis, which can be used to analyze elements and their chemical states at 3–5 nm thickness on a solid surface. In this study, XPS (Axis Ultradld, Kratos, UK) was used to analyze the main organic and inorganic functional groups in the catalyst. The results were analyzed using Xpspeak 4.1 software combined with the Avantage method.
Characterization of PR bio-oil. The components of the catalytic bio-oil were determined by gas chromatography–mass spectrometry (GC–MS, HP6890 series GC, HP5973 MS detector) with a capillary column (Agilent: HP-5MS, 19091S-433; 30 m × 0.25 mm inner diameter × 0.25μm d.f.). The chro- matographic methods were as follows: the initial temperature was 50 °C and kept for 5 min, then rose to 300 °C at 4 °C/min, and the shunt ratio was 1:30. The AMDIS 2.73 and NIST2017 programmes were used for the identification of compounds.
3. Results
3.1. Analysis of PR pyrolysis characteristics
TG (a) and DTG (b) curves showed that the heating rate had similar effects on the pyrolysis of penicillin fermentation residue. Therefore, only need to select the heating rate (20°C/min) to analyze the pyrolysis process of PR.
According to the rate of weight loss, the pyrolysis process is analyzed from three stages. The temperature range of 20∼220°C is the first stage of pyrolysis, and the process is mainly water evaporation; The second stage occurred in the range of 220°C ∼ 560°C, which was mainly the volatile separation stage. The volatiles is released quickly in the third stage of the temperature range of 275 ∼ 325°C. From the DTG curve, it is found that the devolatilization rate reaches the maximum around 300°C, and decreases significantly when it exceeds 600°C. When the temperature is higher than 600°C the PR further decomposes and undergoes cyclic condensation and re-solidification. When the temperature is raised to about 1000°C, the TG curve shows that the solid residue accounts for 23.23% of the original sample weight.
Thermogravimetric analysis showed that PR had good pyrolytic properties. The pyrolysis of PR can produce 76%∼78% of the pyrolysis gas (condensable and non-condensable), which indicates that the pyrolysis of PR has important research value for oil and gas production.
3.2. Pyrolysis temperature
3.2.1. Product yields
The results in shown that the yield of bio-oil first increased and then decreased in the temperature range of 400°C to 700°C. It reaches the maximum value at 600°C(33.11%±0.34 wt.%), and the yield decreases when it rises to 700°C (21.3%±0.41 wt.%).This is because low temperature facilitates the decomposition of organic matter to form more unstable transition state products, and the decomposition or isomerization of transition state compounds promotes the formation of bio-oil. However, high temperatures will further decompose condensable vapours into non-condensable gas, which willreduce the yield of the oil phase [Citation32]. The results are consistent with the pyrolysis process of lignocellulose, algae, and other biomass [Citation33–35]. This indicates that there is a suitable temperature threshold for biomass pyrolysis, and the yield of the oil phase is reduced beyond this temperature threshold [Citation33–35]. When the pyrolysis temperature gradually increased, the macromolecular organic compounds (protein, polysaccharide, lipid) in PR were continuously cracked, resulting in the decrease of residue (from 32.91% to 19.6%). During pyrolysis at 600 ∼ 700 °C, the yield of pyrolysis gas increased rapidly (45.41% ∼ 56.83%), the yield of bio-oil decreased sharply (33.11% ∼ 23.99%), and the yield of solid residue decreased slowly (21.51% ∼ 19.6%). This indicates that some of the macromolecular organic compounds which are easy to condense are cracked into non-condensable pyrolysis gas during high-temperature pyrolysis.
3.2.2. Component analysis
Detection and analysis of the relative content of the substances in the biological oil, results are shown in Table S1. Classification analysis, the compounds of biological oil can be divided into hydrocarbons, oxygenated hydrocarbons, and nitrogenous organic compounds. The statistical results are shown in .
Figure 4. Influence of temperatures on the components of pyrolytic bio-oil: (a) types of compounds; (b) oxygenated compounds; (c) nitrogen-containing compounds; (d) hydrocarbons.
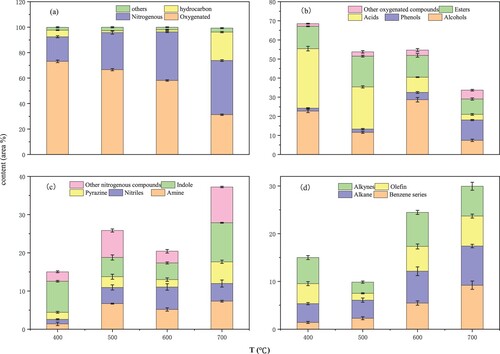
The composition analysis of bio-oil after pyrolysis at different temperatures (Table S2) showed that the proportion of acids and alcohols were higher in the pyrolysis oil products at the final temperature of 400–600°C, which accounted for 53.03%, 45.98%, and 53.18% of the total peak area, respectively. At 700°C, the peak area of acids in bio-oil products is significantly reduced, while the peak areas of alcohols, amines, and benzenes are higher, accounting for 32.24%.
shows that oxygen-containing hydrocarbons and nitrogen-containing substances account for the highest proportion. This was caused by the high content of protein substances (45.40%) in the PR. The peak area of oxygenated hydrocarbons is negatively correlated with the increase in temperature (73%∼31%), and the content of nitrogenous compounds increased gradually (19%∼43%). In addition, at 700°C, the proportion of hydrocarbons increased rapidly (22%). This is because high temperature provides more energy for the pyrolysis of bio-oil. Acidic substances are prone to cracking, condensation, and other reactions at high temperatures, which increases the chance of breaking long-chain substances and the possibility of ring-opening and cracking of cyclic compounds [Citation36,Citation37].
The classification statistics of the compound composition in the oil phase of the PR under the temperature range of 400°C ∼700°C (Table S1) [Citation38]. As the temperature increases, the percentage of alcohols and acids in oxygen-containing compounds decreases, and the percentage of phenols increases (Table S1, Fig.4b). This is consistent with the description of Nur Hidayah Zainan in the preparation of bio-oil by pyrolyzing Chlorella [Citation33], which is caused by the breaking of carbon-oxygen bonds at high temperatures. The proportion of nitrogenous substances in the oil phase increases significantly at 700°C, especially indole and pyrazine (Table S1, Fig.4c). Hao et al. also reached the same conclusion in the experiment of pyrolysis of cyanobacteria to prepare bio-oil and nitrogen oxide compounds [Citation39]. It shows that denitrification is a key problem in the study of oil production from pyrolytic biomass. The content of hydrocarbons increased significantly in the bio-oil pyrolyzed at 700°C, especially benzene and alkanes (Table S1, Fig.4d). This is the high temperature that provides enough energy to break the carbon-nitrogen bonds and carbon-oxygen bonds to form hydrocarbons.
As shown in , compounds can be divided into short chains (C1 ∼ C4, C5 ∼ C13) and long chains (C14 ∼ C22, > C22) according to the carbon chain length. The highest proportion of bio-oil is C5 ∼ C13 and C14 ∼ C22. The main component of known gasoline is C5∼C13, and the main component of diesel is C14 ∼ C22. Therefore, the bio-oil from the pyrolysis of PR is expected to become a new fuel after further deoxidation and denitrification treatment in the future. From 400 to 700°C, the proportion of C5 ∼ C13 in bio-oil gradually increases (26% to 64%), while the proportion of C14 ∼ C22 gradually decreases (47% to 16%). This change indicates that the increase in temperature is conducive to the generation of short-chain substances and the decomposition of long-chains. As the temperature increases, the proportion of compounds greater than C22 decreases (slightly increases at 600°C), indicating that the increase in temperature will accelerate the scission of long carbon chains and the decomposition of macromolecular substances [Citation40].
3.3. Effect of catalysis on pyrolysis products and properties
3.3.1. Product yield under different catalyst action
Metal ions were uniformly loaded on the HZSM-5 molecular sieve to prepare a mixed catalyst. The yield of PR products from catalytic pyrolysis is shown in . The types of metal catalysts seriously affect the yields of pyrolysis products. The yield of pyrolysis oil (15.96% – 16.9%) was lower than that of PR alone (33.11%), and the yield of solid residue (27.46% – 30.24%) was higher than that of PR alone (21.51%); the yield of catalytic pyrolysis others (53.8% – 55.64%) was significantly higher than that of PR alone (45.41%). There are two main reasons for this. First of all, the HZSM-5 molecular sieve has a rich pore structure and a large specific surface area will absorb some volatile substances, making the mass of the catalyst increased, the mass of the biological oil reduced. Secondly, catalytic pyrolysis promotes volatile decomposition and produces more substances with a relatively small molecular weight, which are not easy to be condensed and enter into the pyrolysis gas [Citation41,Citation42].
3.3.2. Composition analysis of bio-oil under different catalysts
The components of bio-oil from catalytic pyrolysis were analyzed by GC-MS (Table S2 ∼ Table S7). After analysis and summary, the distribution of carbon chains in bio-oil compounds is shown in . Experimental data shows that the ratio of C5 ∼ C22 under catalysis is higher than that of non-catalytic pyrolysis, indicating that catalytic pyrolysis can increase the proportion of the target component in bio-oil, which is a compound with a carbon chain of C5 ∼ C22. Among them, CuO/HZSM-5 and Ag/HZSM-5 had a better catalytic effect, and the ratio of C5 ∼ C22 in bio-oil (86.3% and 88.12%, respectively) was significantly higher than that of penicillin residue alone (81.68%). In addition, the proportion of C5 ∼ C13 in the process of catalytic pyrolysis of bio-oil decreases, and the proportion of C14∼C22 increases significantly, indicating that bio-oil contains more diesel components.
The classification was shown in according to the material composition of bio-oil. According to the analysis of compound composition (a), the production of oxygen-containing hydrocarbons was the highest (47.36%∼69.9%), followed by nitrogenous compounds (19.74%∼39.99%), and the production of hydrocarbons (1.43%∼15.02%) and other substances was less (1.52%∼5.23%).
Figure 8. Effect of catalytic pyrolysis of PR on bio-oil components: (a) types of compounds; (b) oxygenated compounds; (c) nitrogen-containing compounds; (d) hydrocarbons.
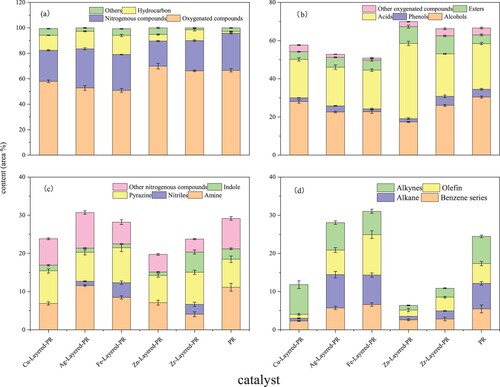
b shows the percentages of different oxygen-containing substances in catalytic and non-catalytic pyrolysis oils. By comparison, it is found that except for the ZnO/HZSM-5 catalyst, it can reduce the proportion of oxygen-containing compounds in the pyrolysis oil, and Fe2O3/HZSM-5 has the best effect. From the analysis of oxygen-containing hydrocarbons, the proportion of alcohols, phenols, and acids decreased, and the proportion of esters increased. It showed that Fe2O3/HZSM-5 catalyst can promote the cleavage of the C-O bond and the occurrence of esterification reaction. However, The increase in the proportion of acid in bio-oil will reduce the quality of the oil and cause equipment corrosion. Catalytic pyrolysis can effectively reduce the proportion of acid, which has guiding value for research on the utilization of bio-oil.
Among the components of bio-oil (c), the main components of nitrogen-containing compounds are amines, nitriles, pyrazines, indoles, and other substances. The catalytic pyrolysis of PR by ZnO/HZSM-5 can effectively reduce the content of nitrogen-containing compounds. It can effectively reduce the amine, pyrazine, and indole in the bio-oil, and the proportion of nitrogen-containing heterocyclic substances is relatively small and does not generate nitrile substances.
According to.8d, the catalytic pyrolysis of PR can significantly improve the generation of hydrocarbon substances. When penicillin fermentation residue was pyrolyzed alone, the composition of hydrocarbons was 1.43%, and the peak area (%)of hydrocarbons in bio-oil was significantly increased (5.17%∼15.02%) under the action of five catalysts. Among the various catalysts, Fe2O3/HZSM-5 has the most significant catalytic effect (15.02%). Besides, the main components of hydrocarbons in bio-oil are benzene series, alkanes, alkenes, and alkynes. This indicates that Fe2O3/HZSM-5 can promote the fracture of a carbon-oxygen single bond and a carbon-oxygen double bond, accompanied by the occurrence of complex alkylation, reforming isomerization, and other reactions, and improve the yield of hydrocarbons [Citation43]. At the same time, Fe2O3/HZSM-5 catalytic pyrolysis of penicillin fermentation broth can not only significantly increase the content of hydrocarbons, but also the proportion of benzene series in the four kinds of hydrocarbon synthesis is small, which makes chain hydrocarbons account for a relatively high proportion.
4. Conclusions
The results showed that 600℃ was the best pyrolysis temperature for PR. At this temperature, the bio-oil yield was the highest, and the total ratio of C5∼C13 and C14∼C22 was the largest. Catalytic pyrolysis can not only reduce the proportion of oxygen-containing hydrocarbons and Nitrogenous compounds in the bio-oil but also significantly increase the proportion of hydrocarbons and C5∼C22. This experiment has guiding value for the deoxygenation and denitrification of biological oil. After further refining, bio-oil is expected to become a new energy source.
Author contributions
Conceptualization, Lihui Feng, Chen Hong; methodology, Yi Xing, Zaixing Li; validation, Lihui Feng, Xiumei Zhao, Jianwei Lü, and Yongtao Lü; formal analysis, Lihui Feng; investigation, Lihui Feng; writing—original draft preparation, Lihui Feng; writing—review and editing, Lihui Feng, and Yan Qin. All authors have read and agreed to the published version of the manuscript.
Supplementary_Materials.docx
Download MS Word (456.2 KB)Acknowledgments
The authors appreciate the support and engagement of the National high-level talent special support plan (grant NO.ZYZZ2018001), Key R&D Program of Hebei Province (grant NO. 19273809D), for the financial support, and the North China Pharmaceutical Co, Ltd for the PR provided for this research work.
Data availability
Data will be made available on request.
Disclosure statement
No potential conflict of interest was reported by the author(s).
Additional information
Funding
References
- Ying GG, et al. China must reduce its antibiotic Use. Environ Sci Technol. 2017;51:1072–1073. https://doi.org/10.1021/acs.est.6b06424.
- Wang NC. Understanding antibiotic overprescribing in China: A conversation analysis approach. Soc Sci Med; 262:113251–113266.
- Liu X, Steele JC, Meng XZ. Usage, residue, and human health risk of antibiotics in Chinese aquaculture: A review. Environ Pollut; 223:161–169.
- Wang J, Zhang MY, Liu J, et al. Using a targeted ecopharmacovigilance intervention to control antibiotic pollution in a rural aquatic environment. Sci Total Environ; 696:134007–134014.
- Wang B, Li G, Cai C, et al. Assessing the safety of thermally processed penicillin mycelial dreg following the soil application: organic matter's maturation and antibiotic resistance genes. Sci Total Environ; 636:1463–1469.
- Chen Z, Zhang S, Wen Q, et al. Effect of aeration rate on composting of penicillin mycelial dreg. J Environ Sci; 37:172–178.
- Trubetskaya A, et al. A methodology for assessing and monitoring risk in the industrial wastewater sector. Water Resour Ind. 2021;25(100146). https://doi.org/10.1016/j.wri.2021.100146.
- Li C, Zhang G, Zhang Z, et al. Alkaline thermal pretreatment at mild temperatures for biogas production from anaerobic digestion of antibiotic mycelial residue. Bioresour Technol; 208:49–57.
- Vichaphund S, Aht-Ong D, Sricharoenchaikul V, et al. Characteristic of fly ash derived-zeolite and its catalytic performance for fast pyrolysis of Jatropha waste. Environ Technol; 35:2254–2261.
- Sotoudehnia F, Baba Rabiu A, Alayat A, et al. Characterization of bio-oil and biochar from pyrolysis of waste corrugated cardboard. J Anal Appl Pyrolysis; 104722:1–104722:12.
- Chandran R, et al. Characteristics of bio-oil from continuous fast pyrolysis of Prosopis juliflora. Energy. 2020;190:116387–116398. https://doi.org/10.1016/j.energy.2019.116387.
- Codignole Luz F, Cordiner S, Manni A, et al. Biomass fast pyrolysis in screw reactors: prediction of spent coffee grounds bio-oil production through a monodimensional model. Energy Convers Manage; 168:98–106.
- Wang Z, et al. Characteristics of biochars prepared by co-pyrolysis of sewage sludge and cotton stalk intended for use as soil amendments. Environ Technol; 41:1347–1357.
- Du Y, et al. Thermal behavior and kinetics of bio-ferment residue/coal blends during co-pyrolysis. Energy Convers Manage; 88:459–463.
- Tran QK, et al. Fast pyrolysis of pitch pine biomass in a bubbling fluidized-bed reactor for bio-oil production. J Ind Eng Chem; 98:168–179.
- Li Y, et al. Study on the nitrogen migration mechanism during penicillin fermentation residue fast pyrolysis based on the substance transformation and canonical variational theory. Sci Total Environ; 737:139739–139755.
- Li Y, et al. Fractal characteristics of biochars derived from Penicillin v potassium residue pyrolysis. J Anal Appl Pyrolysis. 2019;141:104636–104646. https://doi.org/10.1016/j.jaap.2019.104636.
- Cheng Y-T, Huber GW. Production of targeted aromatics by using Diels–Alder classes of reactions with furans and olefins over ZSM-5. Green Chem. 2012;14:3114–3126.
- Paysepar H, Rao KTV, Yuan Z, et al. Improving activity of ZSM-5 zeolite catalyst for the production of monomeric aromatics/phenolics from hydrolysis lignin via catalytic fast pyrolysis. Appl Catal, A; 563:154–162.
- Liu S, et al. Fast microwave-assisted catalytic co-pyrolysis of corn stover and scum for bio-oil production with CaO and HZSM-5 as the catalyst. Bioresour Technol; 204:164–170.
- Widayatno WB, et al. Upgrading of bio-oil from biomass pyrolysis over Cu-modified β-zeolite catalyst with high selectivity and stability. Appl Catal, B; 186:166–172.
- He S, et al. Catalytic pyrolysis of crude glycerol over shaped ZSM-5/bentonite catalysts for bio-BTX synthesis. Appl Catal, B; 235:45–55.
- Chen Y, et al. Pyrolysis of antibiotic mycelial dreg and characterization of obtained gas, liquid and biochar. J Hazard Mater. 2021;402:123826–123835.
- Vichaphund S, Sricharoenchaikul V, Atong D. Utilization of fly ash-derived HZSM-5: catalytic pyrolysis of Jatropha wastes in a fixed-bed reactor. Environ Technol; 38:1660–1672.
- Williams* PT, Nugranad N. Comparison of products from the pyrolysis and catalytic pyrolysis of rice husks. Energy. 2000;25:493–513.
- Lazdovica K, Kampars V, Grabis J. Effect of zinc-containing nanopowders on the catalytic intermediate pyrolysis of buckwheat straw by using TGA-FTIR method. J Anal Appl Pyrolysis; 152:104882–104891.
- Rahman MM, Chai M, Sarker M, et al. Catalytic pyrolysis of pinewood over ZSM-5 and CaO for aromatic hydrocarbon: Analytical Py-GC/MS study. J Energy Inst; 93:425–435.
- Wang L, Shen Y. Pyrolysis characteristics of cellulosic biomass in the presence of alkali and alkaline-earth-metal (AAEM) oxalates. Cellulose; 28:3473–3483.
- Song Y, Hu J, Liu J, et al. Catalytic effects of CaO, Al2O3, Fe2O3, and red mud on Pteris vittata combustion: emission, kinetic and ash conversion patterns. J Cleaner Prod; 252:119646–119657.
- Che Q, et al. Influence of physicochemical properties of metal modified ZSM-5 catalyst on benzene, toluene and xylene production from biomass catalytic pyrolysis. Bioresour Technol; 278:248–254.
- Botas JA, Serrano DP, García A, et al. Catalytic conversion of rapeseed oil for the production of raw chemicals, fuels and carbon nanotubes over Ni-modified nanocrystalline and hierarchical ZSM-5. Appl Catal, B; 145:205–215.
- Zhu X, et al. Tracking the conversion of nitrogen during pyrolysis of antibiotic mycelial fermentation residues using XPS and TG-FTIR-MS technology. Environ Pollut; 211:20–27.
- Zainan NH, Srivatsa SC, Li F, et al. Quality of bio-oil from catalytic pyrolysis of microalgae Chlorella vulgaris. Fuel; 223:12–19.
- Chen W-H, Eng CF, Lin Y-Y, et al. Independent parallel pyrolysis kinetics of cellulose, hemicelluloses and lignin at various heating rates analyzed by evolutionary computation. Energy Convers Manage; 221:113165–113177.
- Xiong Z, et al. Assessing the chemical composition of heavy components in bio-oils from the pyrolysis of cellulose, hemicellulose and lignin at slow and fast heating rates. Fuel Process Technol; 199:106299–106307.
- Rocha MV, Vinuesa AJ, Pierella LB, et al. Enhancement of bio-oil obtained from co-pyrolysis of lignocellulose biomass and LDPE by using a natural zeolite. Therm Sci Eng Prog; 19:100654–100662.
- Zong P, et al. Pyrolysis behavior and product distributions of biomass six group components: starch, cellulose, hemicellulose, lignin, protein and oil. Energy Convers Manage; 216:112777–112791.
- Trubetskaya A, Souihi N, Umeki K. Categorization of tars from fast pyrolysis of pure lignocellulosic compounds at high temperature. Renewable Energy; 141:751–759.
- Hao J, Qi B, Li D, et al. Catalytic co-pyrolysis of rice straw and ulva prolifera macroalgae: effects of process parameter on bio-oil up-gradation. Renewable Energy; 164:460–471.
- Debiagi PEA, et al. Algae characterization and multistep pyrolysis mechanism. J Anal Appl Pyrolysis; 128:423–436.
- Cheng S, Wei L, Julson J, et al. Upgrading pyrolysis bio-oil through hydrodeoxygenation (HDO) using non-sulfided Fe-Co/SiO2 catalyst. Energy Convers Manage; 150:331–342.
- Bharath G, et al. Catalytic hydrodeoxygenation of biomass-derived pyrolysis oil over alloyed bimetallic Ni3Fe nanocatalyst for high-grade biofuel production. Energy Convers Manage; 213:112859–112872.
- Cordero-Lanzac T, et al. Stability of an acid activated carbon based bifunctional catalyst for the raw bio-oil hydrodeoxygenation. Appl Catal, B; 203:389–399.