Abstract
To understand fully the function of mitochondria during the development of cells and organs, it is important to elucidate the dynamics of their morphology. However, the detailed morphology of mitochondria during meiosis has not yet been studied in algae. We examined the mitochondrial morphology of Chlamydomonas reinhardtii and classified zygotes into seven types by mitochondrial morphology in order to analyse the morphological change in mature and meiotic zygotes. We also investigated the oxygen consumption of living zygotes and the effects of tubulin and actin polymerization inhibitors on mitochondria, using fluorescence microscopy and oxygen electrodes. During zygote maturation, mitochondria fragmented into small particles, with a large decrease in oxygen consumption. When mature zygotes were exposed to light, mitochondria became tubular and formed a network, and oxygen consumption gradually recovered. At the same time, particle-like mitochondrial nucleoids became stringy and produced new nucleoid particles. Tubular mitochondria accumulated around the cell nucleus and then spread throughout the cell. Cell division followed (first and second rounds), and the resultant daughter cells had tubular mitochondria in a mesh-like arrangement. An inhibitor of tubulin polymerization, demecolcine, inhibited the assembly of mitochondria around the cell nucleus, whereas an inhibitor of actin polymerization, latrunculin B, inhibited the formation of tubular mitochondria. These results suggest that microtubules are probably involved in mitochondrial accumulation around the cell nucleus, whereas microfilaments may maintain the tubular network of mitochondria.
Introduction
In most eukaryotic cells, mitochondria are the primary energy-generating organelles. They form dynamic interconnected networks in many cell types of various species from yeast to humans (Bereiter-Hahn, Citation1990; Nunnari et al., Citation1997; Jakobs et al., Citation2003). Recent studies have investigated the dynamics of mitochondrial morphology in order to analyse the development of cells and diseases (Okamoto & Shaw, Citation2005; Chan, Citation2006). Such studies have focused on Saccharomyces cerevisiae, a budding yeast, in which mitotic cells have tubular mitochondrial networks. However, a single, large, branched mitochondrion can change into many spherical mitochondria during the pre-sporulation and late meiotic periods (Miyakawa et al., Citation1984). Seventeen proteins have been identified from mutants with mitochondrial morphological disorders (Okamoto & Shaw, Citation2005; Chan, Citation2006). These proteins control mitochondrial fusion, fission and tubulation. Dynamic changes in morphology have also been observed as mitochondria are distributed to progeny (Gorsich & Shaw, Citation2004). However, the dynamics of mitochondrial morphology during the entire life cycle, including mitotic and meiotic division, have only been studied in budding yeast.
In the isogamous alga, Chlamydomonas reinhardtii, mitochondrial morphology was initially observed during the asexual cycle (Ehara et al., Citation1995; Hiramatsu et al., Citation2006). Mitochondria were classified into five types based on observations using dimethylaminostyrylmethylpyridinium iodine (DASMPI; Ehara et al., Citation1995). However, based on staining living cells with DiOC6 (Hiramatsu et al., Citation2006), mitochondria were later found to have a mesh-like structure throughout the asexual cycle. Nevertheless, mitochondrial morphology during maturation, pre-meiosis, and meiosis remains unclear. The observation of mitochondria during these stages is very difficult because mature zygotes have thick, solid walls (Harris, Citation1989). We have successfully observed mitochondria during meiosis in living zygotes (Aoyama et al., Citation2006), however, the details of mitochondrial morphology, configuration, and movement during the mature and meiotic stages remain unclear. Furthermore, mitochondria are inherited by the selective elimination of the maternal mitochondrial genome immediately before the first meiotic division (Aoyama et al., Citation2006). We therefore examined mitochondria and mitochondrial nucleoid morphology from the completion of zygote formation through the completion of meiosis and estimated the relationship between morphology and the cytoskeleton, using fluorescence microscopy with SYBR Green I and DiOC6 dyes. We classified zygotes into seven types based on the various mitochondrial morphologies and estimated their appearance frequencies in order to analyse the transitions between mitochondrial morphologies. We also measured zygotic oxygen consumption and the influence of physiological tubulin and actin polymerization inhibitors on mitochondrial morphology. We found that mitochondria have various morphologies (e.g. mesh-like, particle-like), assembled around the cell nucleus, and subsequently spread throughout the cell before meiosis I. Zygotes with mesh-like mitochondria had higher oxygen consumption, whereas those with particle-like mitochondria had lower oxygen consumption. Microtubules are probably involved in mitochondrial assembly around the nucleus, as well as their distribution throughout the rest of the cell. Microfilaments probably help to form tubular and mesh-like mitochondria.
Materials and methods
Cell strains and culture
We used wild-type mt + and mt − strains of Chlamydomonas reinhardtii 137c (Chlamydomonas Collection Center, Duke University, USA). The cells were cultured separately on 1.2% agar plates at 22°C under a 12-h : 12-h light–dark cycle (Nakamura et al., Citation1986). Gametes were induced by incubating cultured cells in nitrogen-free medium (Nakamura et al., Citation1991). The methods of zygote formation, maturation, and germination were as described previously (Nakamura et al., Citation1991), except that zygotes were cultured for 7 days in the dark.
Cell staining
To stain cell nuclei, mitochondrial and chloroplast nucleoids in living zygotes, SYBR Green I (FMC Bioproducts, Rockland, ME, USA) was added to cell suspensions to a final dilution of 1:1000. To observe the morphology of mitochondria in living zygotes, cells were stained with DiOC6 (Molecular Probes, Eugene, OR, USA) at a final concentration of 0.1 µg/ml (Nishimura et al., Citation1998). Stained cells were observed under a fluorescence microscope (Olympus BHS-RFK, Olympus, Tokyo, Japan) following the methods of Nishimura et al. (Citation1998). Mature zygotes were stained by incubating them in a solution containing each stain for 30–60 min. The cells were then washed by gentle centrifugation and observed under a microscope.
Measurements of zygotic oxygen consumption
The oxygen content of 3 ml of water containing zygotes was measured using an oxygen electrode (Rank Brothers Ltd, England) at 25°C in the dark, following the manufacturer's instructions. Cell density was estimated using a haemocytometer.
Inhibition of microtubule and actin filament formation
To inhibit tubulin polymerization, zygotes were treated with demecolcine (Wako, Osaka, Japan) at final concentrations of 2.5 and 25 µg/ml (approximately 6.7 and 67 µM) immediately after light exposure and when zygotes were mature, respectively. To inhibit actin polymerization, zygotes were treated with latrunculin B (Wako, Osaka, Japan) at final concentrations of 30 and 300 nM immediately after light exposure and when zygotes were mature, respectively. Without washing out the inhibitors, cells were stained with DiOC6 at various stages of the culture. These cells were used for morphological observations and oxygen consumption experiments.
Results
Mitochondrial morphology in mature zygotes
One day after zygote formation, mitochondria were tubular and arranged in intricate mesh-like patterns (). Mesh-like mitochondria were usually localized at the surface of the cell, but tubular branches were often observed inside the cell, indicating that mitochondria had penetrated the chloroplast (). Mitochondrial nucleoids were scattered throughout zygotes (; arrowhead) and were distinguishable from chloroplast nucleoids (; arrow) by their smaller size. Furthermore, mitochondrial nucleoids were observed in mitochondria (; arrowhead).
Figs 1–18. Fluorescence microscopic observation of various mitochondria during zygote maturation in the dark. . Fluorescence micrographs of zygotes stained with DiOC6 (), SYBR Green I (), and both SYBR Green I and DiOC6 simultaneously (). . Images focused on the surface of a single zygote. . Images focused on the inside of a single zygote. The images of the cells were taken 0 (), 3 (), and 7 days () after transfer to darkness. Dark treatment for zygote maturation started immediately after day 1 of zygote formation. Asterisk: cell nucleus. Arrow: chloroplast nucleoid. Arrowhead: mitochondrial nucleoid. Scale bar: 5 µm.
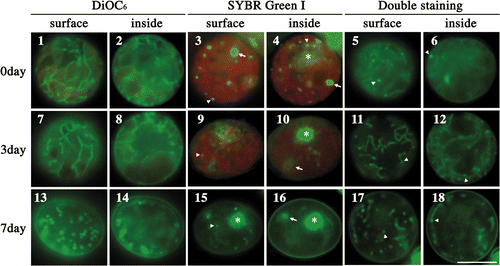
Fig. 19. Frequencies of zygotes classified into three types according to mitochondrial morphology after transferring young zygotes to darkness: zygotes with mesh-like mitochondria (grey line), zygotes with fragmented mitochondria (dashed line), and zygotes with particle-like mitochondria (black line). Mitochondrial features were determined by fluorescence microscopy. Standard deviations were calculated from three independent experiments. 311, 245, 220, 332 cells were counted at 1, 3, 5, 7 days, respectively.
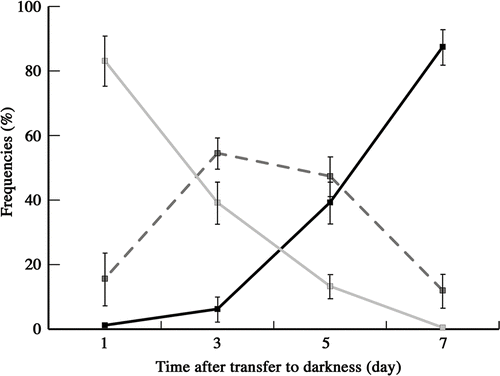
Figs 20–61. Fluorescence micrographs of mature zygotes after light exposure. Fluorescence micrographs of zygotes stained with DiOC6 (), SYBR Green I (), and both SYBR Green I and DiOC6 simultaneously (). . Images focused on the surface layer of a single zygote. . Images focused on the inside of a single zygote. The images were taken approximately 0 h (), 3 h (), 7 h (), 11 h (), 14 h (), 16 h () and 20 h () after light exposure. Asterisk: cell nucleus. Arrow: organellar nucleoid. Arrowhead: mitochondrial nucleoid. Scale bar: 5 µm.
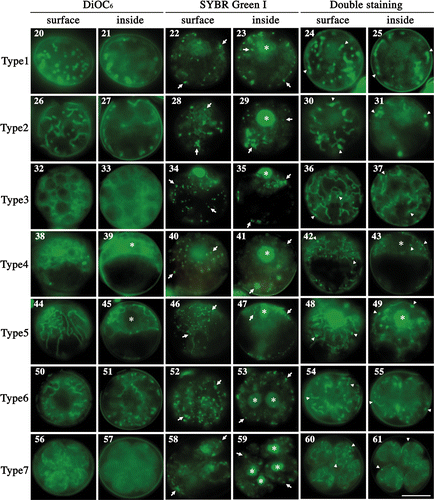
Zygotes were incubated to maturity in the dark for 1 week. As maturation progressed, mitochondria began to divide and form fragments (), the lengths of which gradually decreased. Ultimately, most mitochondria formed particle-like structures at the surface of mature zygotes (). In maturing and mature zygotes, mitochondrial nucleoids appeared as particles scattered over the zygotic surfaces (; arrowhead). Mitochondrial nucleoids were observed in mitochondria (; arrowhead), although a large nucleoid inside the zygotes was a chloroplast (; arrow). Thus, during maturation, mitochondrial nucleoids began to spread over the surface layer of the cell. However, it is not clear whether each mitochondrial particle had one nucleoid, or if, in mature zygotes, some particles had no nucleoids ().
Fig. 62. Frequencies of mature zygotes classified into seven types according to mitochondrial morphology after light exposure. Mitochondrial morphology was determined by fluorescence microscopy. Standard deviations were calculated from three independent observations. 332, 295, 291, 586, 666, 471, 171, 221 cells were counted at 0, 3, 6, 9, 12, 15, 18, 21 h, respectively.
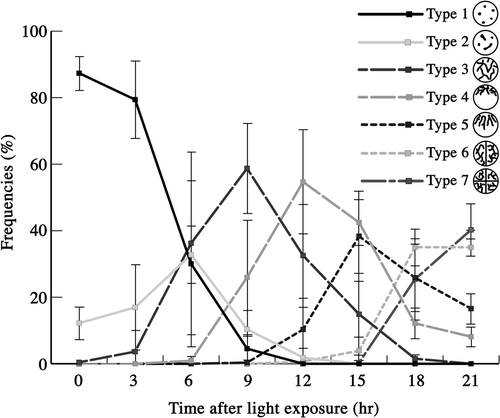
Transition of mitochondrial morphology during zygote maturation in the dark
We analysed mitochondrial morphology during zygote maturation in the dark () and classified the morphology into three types: mesh-like (), fragments (), and particles (). One day after young zygotes were transferred to the dark, 80% of zygotes had mesh-like mitochondria. Between days 3 and 5, the frequency of fragmented mitochondria increased to 50%. With fragmentation, the frequency of particles slowly increased to 40% at day 5 and was >80% after 7 days in the dark.
Figs 63–71. Changes in nucleoid morphology after exposing mature zygotes to light. Fluorescence micrographs of nucleoids in mature zygotes after light exposure. Zygotes were stained with SYBR Green I. . Fluorescence micrographs of a zygote. . An enlarged portion of a nucleoid shown by an arrow in , respectively. Images taken approximately 0 h (), 8 h () and 10 h () after light exposure. Arrow: particle-like or stringy nucleoid. Arrowhead: newly produced nucleoid. Scale bars: 5 µm (); 1 µm (). . Frequencies of mature zygotes classified into three types according to the morphology of the nucleoid after light exposure: zygotes with particle-like nucleoids with no apparent production of new nucleoids (see ) (dashed line), zygotes with less than five stringy nucleoids with apparent low production (see ) (black line), and zygotes with more than five stringy nucleoids or long stringy nucleoids with apparent high production (see ) (grey line). Standard deviations were calculated from three independent experiments. 108, 70, 247, 144, 145, 228, 155, 303, 256, 161, 126 cells were counted at 2, 4, 6, 8, 10, 12, 14, 16, 18, 20, 22 h, respectively.
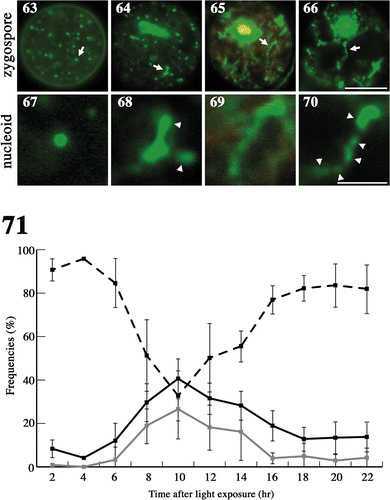
Transitions among types of mitochondrial morphology during pre-meiosis and meiosis
We observed mitochondrial morphology and organelle nucleoids during pre-meiosis and meiosis in living cells () and classified zygotes into seven types according to mitochondrial morphology. When mature zygotes that had been incubated in the dark for 7 days were exposed to light, they resumed division; four daughter cells were usually formed in 1 day. We could not distinguish mitochondrial nucleoids from those of chloroplasts by staining with SYBR Green I only (; arrows), therefore we stained zygotes with two dyes simultaneously: SYBR Green I and DiOC6 (; arrowheads).
At first, mitochondria were particle-like and scattered about the surface of cells, but not inside cells (type 1; ). It was not clear if each mitochondrial particle had one nucleoid (). Gradually, the mitochondria transformed in shape from particle-like to short and tubular (type 2; ). Mitochondrial nucleoids were observed in tubular mitochondria (), whereas large plastid nucleoids were observed inside zygotes () and could be distinguished from mitochondrial nucleoids by their larger size (). Tubular mitochondria continued to develop and became branch-like structures scattered throughout the cell (type 3; ) and contained mitochondrial nucleoids (). Mitochondria scattered about the cell surface began to assemble in the area surrounding the cell nucleus, with most mitochondria wrapping the cell nucleus in a three-dimensional manner (type 4; ). Most mitochondrial nucleoids also assembled in the area around the cell nucleus () and there were almost no mitochondria in the nucleus-free hemisphere. After assembly, tubular mitochondria began to radiate into the other hemisphere via the surface layer (type 5; ). Mitochondrial nucleoids also migrated and were then distributed throughout the entire zygote (). The first round of cell division resulted in two nuclei and the development of tubular mitochondria (type 6; ). Once meiosis was completed, there were four daughter cells (type 7; ), all of which had mesh-like mitochondria, and mitochondrial and chloroplast nucleoids distributed throughout the whole zygote (). The chloroplast nucleoids were larger than those of the mitochondria ().
We analysed the transitions between these seven types of mitochondrial morphologies (). Immediately following light exposure, most zygotes were type 1, whose frequency decreased between 3 and 9 h, until there were no type 1 zygotes after 12 h. The frequency of type 2 reached a peak at around 6 h, although the peak value was only 30%, then decreased with the appearance of type 3, becoming almost zero at 9 h. The transition from type 2 to type 3 was rapid. Type 3 and 4 zygotes reached their peaks at approximately 9 and 12 h, respectively. Subsequently, type 5 zygotes appeared. Between 9 and 15 h, the frequency of type 5 increased and peaked at approximately 60%. From 15 h, the frequencies of type 6 and 7 increased continuously. The sequential transition of classes after type 5 was more rapid than that between types 3 and 5. Thus, mitochondrial morphology of zygotes changed sequentially and dramatically from type 1 to type 7 when mature zygotes were exposed to light.
Changes in nucleoid morphology during pre-meiosis and meiosis
We observed nucleoid morphology after mature zygotes were exposed to light (). Mitochondrial nucleoids were localized mainly at the surface (). Immediately after light exposure, they were mostly particle-like (), but some nucleoids became short stringy forms at approximately 8 h () and then long stringy forms at approximately 10 h (). The stringy structures were derived from particle-like nucleoids, which also divided and formed new particle-like nucleoids (; arrowheads).
Fig. 72. Measurement of zygote oxygen consumption. Oxygen consumption was measured from the beginning of zygote maturation until the completion of meiosis. Black arrow: zygotes were transferred to the dark for maturation. Grey arrow: mature zygotes were exposed to light to induce meiosis. Standard deviations were calculated from five independent experiments.
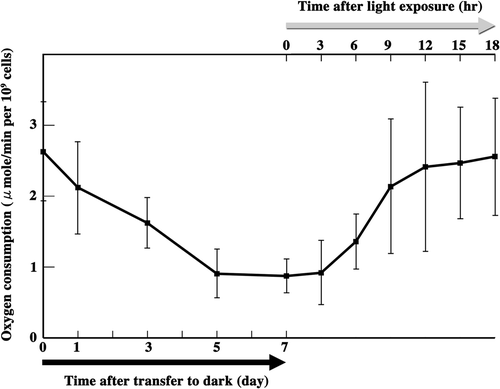
To estimate the timing of new nucleoid production, we measured the frequency of stringy nucleoids in zygotes after light exposure (). We classified zygotes into three types: zygotes with only particle-like nucleoids (type A; ), zygotes with less than five stringy nucleoids per zygote (type B; ), and zygotes with more than five stringy nucleoids per zygote, or with especially long stringy nucleoids (type C; ). The proportion of types B and C increased from 6 h and reached maximum values of 40 and 25% at approximately 10 h, respectively. The proportion of type C decreased gradually and was rarely observed after 16 h, whereas the frequency of type B was 20% at 18 h, remaining unchanged until 22 h. These results suggest that mitochondrial nucleoid DNA multiplied rapidly between 6 and 10 h after light exposure, but the production of new nucleoids did not stop completely, even after 18 h.
Oxygen consumption of zygotes during pre-meiosis and meiosis
We measured the oxygen consumption of zygotes between maturation and the end of meiosis (). Twenty-four hours after zygote formation, oxygen consumption was approximately 2.6 (SD; ±0.7) µmol/min per 109 cells and decreased gradually as maturation progressed. After 7 days in the dark, oxygen consumption decreased to approximately 0.9 (±0.3) µmol/min per 109 cells. Between 3 and 9 h after light exposure to initiate meiosis, oxygen consumption increased to approximately 2.1 (±1.0) µmol/min per 109 cells and reached approximately 2.5 (±0.8) µmol/min per 109 cells by 18 h. Thus, oxygen consumption decreased during maturation but recovered to a level similar to that of young zygotes within 12 h of exposing mature zygotes to light.
Figs 73–87. The effects of demecolcine on mitochondria during meiosis. . The frequency of each type of mitochondria was determined by fluorescence microscopy. Zygotes treated with demecolcine at concentrations of 2.5 µg/ml () and 25 µg/ml () after light exposure. Standard deviations were calculated from three independent experiments. 96, 174, 210, 244, 194, 100 cells were counted at 3, 6, 9, 12, 15, 18 h, respectively (). 69, 168, 326, 212, 198, 139 cells were counted at 3, 6, 9, 12, 15, 18 h, respectively (). . Fluorescence micrographs of zygotes treated with demecolcine. Cells were stained with DiOC6. . Images focused on the surface layer of a single zygote. . Images focused on the inside of a single zygote. The concentration of demecolcine was 2.5 µg/ml () or 25 µg/ml (). Micrographs were taken approximately 9 h (), 12 h (), 15 h (), and 18 h () after light exposure. Scale bars: 5 µm. . The effect of demecolcine on the oxygen consumption of mature zygotes after light exposure. Black solid line, 25 µg/ml demecolcine; black dashed line, 2.5 µg/ml demecolcine; grey solid line, no treatment (). Standard deviations were calculated from five independent experiments.
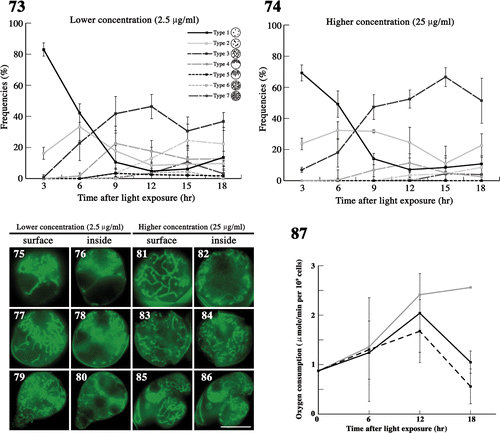
Effect of tubulin polymerization inhibitor on mitochondria in meiotic zygotes
We examined the effects of the tubulin polymerization inhibitor demecolcine on mitochondria after light exposure (). We treated zygotes with two different concentrations of demecolcine, i.e. 2.5 and 25 µg/ml (approximately 6.7 and 67 µM), immediately after light exposure. At the lower concentration, mitochondria transformed from particle-like (type 1) to tubular and dispersed throughout the zygote (type 3) until 9 h (). However, the frequency of zygotes with mitochondria near the cell nucleus (type 4) decreased to 20% at approximately 12 h (). Some zygotes showed incomplete accumulation (), a few zygotes had mitochondria arranged radially (type 5), whereas most had a diagonal arrangement (). In later stages, type 6 and 7 zygotes were rarely observed (). After 12 h, both mitochondria and cells began to exhibit abnormal morphology; cells began to resemble the budding of yeast (), and by 18 h, 10% of zygotes had formed four complete daughter cells ().
Figs 88–102. The effects of latrunculin B on mitochondria during meiosis. . The frequency of each type of mitochondria was determined by fluorescence microscopy. Zygotes treated with latrunculin B at 30 nM () and 300 nM () after light exposure. Standard deviations were calculated from three independent experiments: 74, 169, 122, 117, 283, 129 cells were counted at 3, 6, 9, 12, 15, 18 h, respectively (); 89, 270, 154, 190, 226, 85 cells were counted at 3, 6, 9, 12, 15, 18 h, respectively (). . Fluorescence micrographs of zygotes showing mitochondria treated with latrunculin B. Cells stained with DiOC6. . Micrographs of the surface layer of a single zygote. . Micrographs of the inside of a single zygote. The concentration of latrunculin B was 30 nM () or 300 nM (). The micrographs were taken approximately 6 h (), 15 h (), and 18 h () after light exposure. Arrow: aggregation of mitochondria. Arrowhead: loop-like mitochondria. Scale bars: 5 µm. . The effect of latrunculin B on the oxygen consumption of mature zygotes after light exposure. Black solid line, 300 nM latrunculin B; black dashed line, 30 nM latrunculin B; grey solid line, no treatment (). Standard deviations were calculated from five independent experiments.
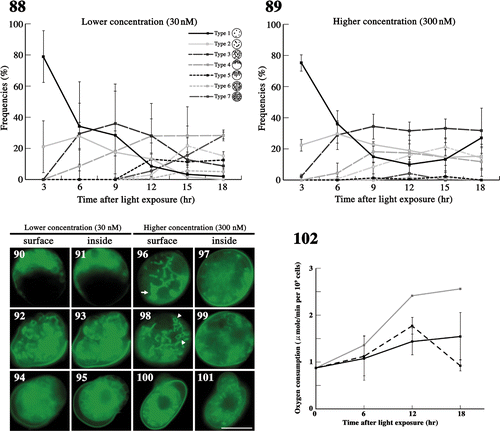
In the higher concentration of demecolcine, mitochondrial morphological changes nearly stopped at type 3 at 9 h (), and meiotic cell division did not occur. Furthermore, the frequency of type 2 increased at 18 h, probably caused by the fragmentation of type 3 mitochondria (). Abnormal mitochondrial morphology and daughter cell shape appeared with a frequency of approximately 10% at 18 h ().
We also measured oxygen consumption with demecolcine in zygotes (). At 6 h, there was no difference in oxygen consumption between inhibitor-treated and untreated zygotes. At 12 h, oxygen consumption of inhibitor-treated zygotes was similar to that at 6 h, but approximately 70–85% lower than in untreated zygotes. At 18 h, oxygen consumption of zygotes at both concentrations decreased sharply, matching the respective mature zygotes after 7 days in the dark. In particular, oxygen consumption of zygotes treated with demecolcine at 2.5 µg/ml was 20% that of untreated zygotes.
Effect of actin polymerization inhibitor on mitochondria in meiotic zygotes
We examined the effects of the actin polymerization inhibitor latrunculin B on mitochondria after light exposure (), treating zygotes with two different concentrations of latrunculin B: 30 and 300 nM.
At the lower concentration, mitochondria migrated toward the zygotic cell nucleus (; type 4). However, radial migration into the nucleus-free hemisphere (type 5) and distribution throughout the whole cell (type 6 and 7) were slightly hindered, and the frequency curves of types 4–6 did not have clear peaks (). Finally, 30% of zygotes formed four distinct daughter cells at 18 h (). Abnormal cell division and abnormal mitochondrial morphology appeared at approximately 12 h, both with frequencies of 20% at 18 h ().
Fig. 103. Dynamics of mitochondria and their nucleoids during pre-meiosis and meiosis in zygotes. Particle-like mitochondria (type 1) that are fragmented during maturation connect to form tubular structures (type 2) after light exposure. The tubular networks in whole zygotes (type 3) assemble around the cell nucleus (type 4) and form a radial structure (type 5). These dynamics are controlled by microtubules. Mitochondria spread into two and four progeny cells (type 6 and 7). The high-level replication of mitochondrial nucleoids is synchronized with the formation of the mitochondrial tubular network. Low-level replication continues until tetrad formation.
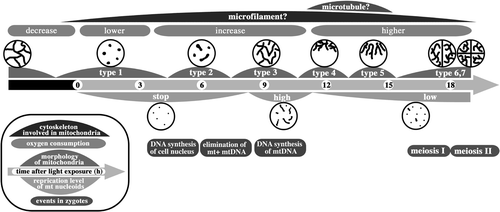
In the higher concentration of latrunculin B, approximately 20% incompletely connected mitochondria (type 2) were observed throughout meiosis (). Changes in mitochondrial morphology were strongly inhibited. In all zygotes, parts of tubular mitochondria aggregated, forming large blocks in the early stages of meiosis (; arrow). Loop-like mitochondria were often observed, probably because of abnormalities in the mitochondrial network (; arrowhead). Thus, abnormal mitochondrial morphology was observed after 9 h (). At 18 h, zygotes with particle-like mitochondria (type 1) increased and the frequency of zygotes with short tubular mitochondria (type 2) remained constant, at 20%. Normal cell division producing four daughter cells was not observed.
We also measured oxygen consumption with latrunculin B in zygotes (). At 6 h, there was no difference in oxygen consumption between inhibitor-treated and untreated zygotes. At 12 h, oxygen consumption of inhibitor-treated zygotes was similar to that at 6 h, but this was approximately 60–75% lower than that of untreated zygotes. At 18 h, oxygen consumption decreased greatly in 30 nM treated zygotes, comparable to the respective mature zygotes after 7 days in the dark. On the other hand, oxygen consumption of zygotes treated with 300 nM latrunculin B did not decrease after 12 h.
Discussion
We performed a detailed examination of mitochondrial behaviour from day 1 of zygote formation through to the completion of meiosis (). Mesh-like mitochondria became particle-like during maturation, accompanied by a decline in oxygen consumption. After exposing zygotes to light, mitochondria became tubular and then assembled near the cell nucleus, and oxygen consumption recovered. Mitochondrial nucleoids were replicated actively. Finally, mitochondria dispersed throughout the cell following a radial structure during meiotic cell division. These mitochondrial dynamics are characteristic of both pre-meiotic and meiotic zygotes, as well as the asexual cycle of C. reinhardtii (Hiramatsu et al., Citation2006).
In Saccharomyces cerevisiae, mitochondrial fusion and fission is important for the mitochondrial transfer to progeny. Three fission proteins (Dnm1p, Mdv1p, and Fis1p) control morphology during meiosis (Gorsich & Shaw, Citation2004). The loss of any one of these, or of fusion proteins, causes defects in mitochondrial distribution during meiosis and sporulation and in inheritance. We found that mitochondrial morphology changed dynamically, especially in the transition to a radial distribution after assembling around the cell nucleus. This could play a role in mitochondrial transfer to progeny, as in yeast, because the pattern occurs immediately before the first meiotic cell division. This suggests that C. reinhardtii may have fission and fusion proteins that facilitate distinct mitochondrial behaviour in meiotic zygotes.
In mice, mitochondria assemble around the cell nucleus during meiosis I, but not meiosis II (Inoki et al., Citation2000). In S. cerevisiae, mitochondria move toward the cell centre and associate with the nuclear membrane during meiosis (Miyakawa et al., Citation1984), but in C. reinhardtii, mitochondria do not assemble around the cell nucleus during mitotic division (Hiramatsu et al., Citation2006), but assemble just before the first meiotic cell division, not during the second division. These results raise the possibility that the assembly of mitochondria around the cell nucleus during meiosis is ubiquitous, occurring in algae as well as animal cells.
Mitochondrial morphology is generally related to mitochondrial activity. In S. cerevisiae, mitochondria become fragmented during stationary phase (Miyakawa et al., Citation1994) and early stages of apoptosis (Okamoto & Shaw, Citation2005). Such mitochondria have low activity, whereas high activity is associated with mitochondrial elongation (Chan, Citation2006). We obtained similar results indirectly by measuring oxygen consumption. Cells with mesh-like or tubular mitochondria had high oxygen consumption, whereas those with particle-like mitochondria had low oxygen consumption. Inhibition of the formation of the normal tubular network of mitochondria using latrunculin B maintained low oxygen consumption and the oxygen consumption of demecolcine-treated zygotes was similarly low. In such zygotes, mitochondria did not accumulate near the cell nucleus, but were tubular. Thus, mitochondrial accumulation may be necessary for the complete recovery of mitochondrial activity, albeit for unknown reasons.
In C. reinhardtii, previous DNA analysis showed that paternal inheritance of mitochondria occurs by the selective elimination of maternal mitochondrial DNA about 6–8 h after the exposure of matured zygotes to light (Aoyama et al. Citation2006). In the present study, particle-like mitochondria started to return to tubular and mesh-like structures between around 6 and 8 h, and selective elimination of maternal mitochondrial nucleoids at the beginning of mitochondrial network formation and surviving nucleoids would be reproduced. However, clear elimination was not observed under fluorescence microscopy, although short stringy nucleoids were observed in zygotes, peaking at approximately 10 h like type-3 zygotes. In vegetative cells, short stringy nucleoids are characteristic of nucleoids that produce new nucleoids, accompanied by nucleoid DNA synthesis (Hiramatsu et al., Citation2006), and is probably necessary to complete the mitochondrial network in zygotes.
In animal cells and Schizosaccharomyces pombe, the mitochondrial movement is controlled by microtubules (Heggeness et al., Citation1987; Hirokawa, Citation1998). In S. pombe, microtubules transfer mitochondria to daughter cells (Yaffe et al., Citation1996) whereas, in plant cells and S. cerevisiae, mitochondria rely on the actin cytoskeleton for movement. In S. cerevisiae, actin filaments and intermediate filaments participate in mitochondrial translocation (Hermann & Shaw, Citation1998; Boldogh et al., Citation2001). In Nicotiana tabacum L., mitochondria move along the actin cytoskeleton and mitochondrial positioning in the cell is related to the location of microtubules (Van Gestel et al., Citation2002). Chlamydomonas reinhardtii has two types of actin genes (Sugase et al., Citation1996; Lee et al., Citation1997). Actin is generally localized in the cell anterior from prophase to anaphase (Harper et al., Citation1992) and in the fertilization tubule in mt + gametes (Detmers et al., Citation1985). However, the structure of microfilaments in zygotes and the relationship between microfilaments and organelle movement is unknown. Treatment of zygotes with an actin polymerization inhibitor inhibited mitochondrial migration around the cell nucleus and led to some abnormal mitochondria, which aggregated and formed loop-like structures. Furthermore, the oxygen consumption of such zygotes decreased. Thus, we assume that the normal tubular morphology of mitochondria is maintained by microfilaments and that abnormal mitochondrial morphology leads to lower oxygen consumption.
The assembly of mitochondria around the cell nucleus was inhibited by a tubulin polymerization inhibitor. In vegetative cells, the cytoplasmic microtubules descend from the basal bodies toward the posterior end of the cell before nuclear division and form an umbrella-like structure (Holmes & Dutcher, Citation1989; Dutcher, Citation2003). In contrast, basal bodies and flagellar roots disappear in young zygotes, and these structures re-form at the beginning of tetrad formation after light exposure (Harris, Citation1989). New basal bodies are occasionally visible at the beginning of the meiotic prophase after light exposure (Cavalier-Smith, Citation1974). We found that the umbrella-like structure was consistent with a dispersed mitochondrial arrangement, extending from the cell nucleus to the other hemisphere. The timing of new basal body formation (Harris, Citation1989) was consistent with the beginning of mitochondrial condensation around the cell nucleus. Thus, we assume that microtubules that extend from basal bodies play a role in mitochondrial accumulation around the cell nucleus and the radiating structure at the surface.
Acknowledgements
This work was supported by a Grant-in-Aid for Scientific Research A to TK (No. 19207004) from the Ministry of Education, Culture, Sports, Science and Technology of Japan.
References
- Aoyama , H , Hagiwara , Y , Misumi , O , Kuroiwa , T and Nakamura , S . 2006 . Complete elimination of maternal mitochondrial DNA during meiosis resulting in the paternal inheritance of the mitochondrial genome in Chlamydomonas . Protoplasma , 228 : 231 – 242 .
- Bereiter-Hahn , J . 1990 . Behavior of mitochondria in the living cell . Int. Rev. Cytol. , 122 : 1 – 63 .
- Boldogh , IR , Yang , HC and Pon , LA . 2001 . Mitochondrial inheritance in budding yeast . Traffic , 2 : 368 – 374 .
- Cavalier-Smith , T . 1974 . Basal body and flagellar development during the vegetative cell cycle and the sexual cycle of Chlamydomonas reinhardtii . J. Cell Sci. , 16 : 529 – 556 .
- Chan , DC . 2006 . Mitochondria: dynamic organelles in disease, aging, and development . Cell , 125 : 1241 – 1252 .
- Detmers , PA , Carboni , JM and Condeelis , J . 1985 . Localization of actin in Chlamydomonas using anti-actin and NBD-phallacidin . Cell Motil. , 5 : 415 – 430 .
- Dutcher , SK . 2003 . Elucidation of basal body and centriole functions in Chlamydomonas reinhardtii . Traffic , 4 : 443 – 451 .
- Ehara , T , Osafune , T and Hase , E . 1995 . Behavior of mitochondria in synchronized cells of Chlamydomonas reinhardtii (Chlorophyta) . J. Cell Sci. , 108 : 499 – 507 .
- Gorsich , SW and Shaw , JM . 2004 . Importance of mitochondrial dynamics during meiosis and sporulation . Mol. Biol. Cell , 15 : 4369 – 4381 .
- Harper , JD , McCurdy , DW , Sanders , MA , Salisbury , JL and John , PC . 1992 . Actin dynamics during the cell cycle in Chlamydomonas reinhardtii . Cell Motil. Cytoskeleton , 22 : 117 – 126 .
- Harris, E.H., editor. (1989). The Chlamydomonas Sourcebook, Academic Press, San Diego, USA
- Heggeness , MH , Simon , M and Singer , SJ . 1987 . Association of mitochondria with microtubules in cultured cells . Pro. Natl. Acad. Sci. USA , 75 : 3863 – 3866 .
- Hermann , GJ and Shaw , JM . 1998 . Mitochondrial dynamics in yeast . Annu. Rev. Cell Dev. Biol. , 14 : 265 – 303 .
- Hiramatsu , T , Misumi , O , Kuroiwa , T and Nakamura , S . 2006 . Morphological changes in mitochondrial and chloroplast nucleoids and mitochondria during the Chlamydomonas reinhardtii cell cycle . J. Phycol. , 42 : 1048 – 1058 .
- Hirokawa , N . 1998 . Kinesin and dynein superfamily proteins and the mechanism of organelle transport . Science , 279 : 519 – 526 .
- Holmes , JA and Dutcher , SK . 1989 . Cellular asymmetry in Chlamydomonas reinhardtii . J. Cell Sci. , 94 : 273 – 285 .
- Inoki , Y , Hakamata , Y , Hamamoto , T , Kinouchi , T , Yamazaki , S , Kagawa , Y and Endo , H . 2000 . Proteoliposomes colocalized with endogenous mitochondria in mouse fertilized egg . Biochem. Biophys. Res. Commun. , 278 : 183 – 191 .
- Jakobs , S , Martini , N , Schauss , AC , Egner , A , Westermann , B and Hell , SW . 2003 . Spatial and temporal dynamics of budding yeast mitochondria lacking the division component Fis1p . J. Cell Sci. , 116 : 2005 – 2014 .
- Lee , VD , Finstad , SL and Huang , B . 1997 . Cloning and characterization of a gene encoding an actin-related protein in Chlamydomonas . Gene , 197 : 153 – 159 .
- Miyakawa , I , Aoi , H , Sando , N and Kuroiwa , T . 1984 . Fluorescence microscopic studies of mitochondrial nucleoids during meiosis and sporulation in the yeast, Saccharomyces cerevisiae . J. Cell Sci. , 66 : 21 – 38 .
- Miyakawa , I , Higo , K , Osaki , F and Sando , N . 1994 . Double staining of mitochondria and mitochondrial nucleoids in the living yeast during the life cycle . J. Gen. Appl. Microbiol. , 40 : 1 – 14 .
- Nakamura , S , Chibana , H and Kuroiwa , T . 1991 . Domination by female cells over preferential digestion of chloroplast nucleoids in Chlamydomonas reinhardtii . Plant Cell Physiol. , 32 : 359 – 364 .
- Nakamura , S , Ito , S and Kuroiwa , T . 1986 . Behavior of chloroplast nucleus during chloroplast development and degeneration in Chlamydomonas reinhardtii . Plant Cell Physiol. , 27 : 775 – 784 .
- Nishimura , Y , Higashiyama , T , Suzuki , L , Misumi , O and Kuroiwa , T . 1998 . The biparental transmission of the mitochondial genome in Chlamydomonas reinhardtii visualized in living cells . Eur. J. Cell Biol. , 77 : 124 – 133 .
- Nunnari , J , Marshall , WF , Stright , A , Murray , A , Sedat , JW and Walter , P . 1997 . Mitochondrial transmission during mating in Saccharomyces cerevisiae is determined by mitochondrial fusion and fission and the intramitochondrial segregation of mitochondrial DNA . Mol. Biol. Cell , 8 : 1233 – 1242 .
- Okamoto , K and Shaw , JM . 2005 . Mitochondrial morphology and dynamics in yeast and multicellular eukaryotes . Annu. Rev. Genet. , 39 : 503 – 536 .
- Sugase , Y , Hirono , M , Kindle , K and Kamiya , R . 1996 . Cloning and characterization of the actin-encoding gene of Chlamydomonas reinhardtii . Gene , 168 : 117 – 121 .
- Van Gestel , K , Kohler , RH and Verbelen , JP . 2002 . Plant mitochondria move on F-actin, but their positioning in the cortical cytoplasm depends on both F-actin and microtubules . J. Exp. Bot. , 53 : 659 – 667 .
- Yaffe , MP , Hayata , D , Verde , F , Eddison , M , Toa , T and Nurse , P . 1996 . Microtubules mediate mitochondrial distribution in fission yeast . Pro. Natl. Acad. Sci. USA , 93 : 11664 – 11668 .