ABSTRACT
In cyanobacteria and other photosynthetic organisms, the mechanism of glucose-6-phosphate dehydrogenase (G6PDH) regulation has long been hypothesized to be a disulphide bond formation/reduction between –SH groups of two internal cysteine residues. In this study, four cysteine codons in the zwf gene of Synechocystis sp. PCC6803 were changed to serine codons, and then each mutation was separately transferred to the chromosome, yielding four cysteine-mutant strains designated ZWFCS101, ZWFCS187, ZWFCS265 and ZWFCS445. Thus, cysteine-mutant G6PDH was analysed in cyanobacteria. Cell-free extracts of the other three mutants were used as an enzyme solution. The mutant ZWFCS187, located close to the active site, completely lost all enzyme activity. To test whether the redox sensitivity of the mutant enzymes was changed, reduced dithiothreitol (DTTred) was added to the enzyme solution immediately prior to the assay. Three of the mutant G6PDHs were found to still be sensitive. This result is inconsistent with other reports where the cysteine-mutant G6PDH lost redox sensitivity. The results herein indicate that redox regulation is not the sole mechanism for the regulation of G6PDH activity, and the regulation most probably involves a sum of interactions at the cellular level, as well as additional contributing factors.
Introduction
Cyanobacteria are the only prokaryotic group that perform oxygen-generating plant-like photosynthesis. They regulate carbon metabolism during light/dark transition by the activation/deactivation of the oxidative pentose phosphate (OPP) and reductive pentose phosphate (RPP) cycles (Smith, Citation1982; Hagen & Meeks, Citation2001). Glucose-6-phosphate dehydrogenase (G6PDH; EC 1.1.1.49) is the first enzyme of the OPP cycle and plays a key role in the regulation of this metabolic pathway (Schaeffer & Stanier, Citation1978). The enzyme is in a deactivated form in light and converted into the active form in the dark. Several factors affect the transition from the active to inactive form of the enzyme. An increase of ATP, NADPH, RuBP and a higher cytosolic pH in the cell during the photosynthetic period decrease the catalytic activity, and resulting metabolic activity (Grossman & McGowan, Citation1975; Schaeffer & Stanier, Citation1978; Lubberding & Bot, Citation1984). The active form of the enzyme is proportionally induced after photosynthetic operation is stopped during a light/dark transition (Schaeffer & Stanier, Citation1978).
The transition between the active and inactive forms of the enzyme was reported to be under redox regulation (Udvardy et al., Citation1983; Cossar et al., Citation1984). In Anacystis nidulans (also known as Synechococcus sp. strain PCC7942), G6PDH turns into the active form when it is treated with reduced dithiothreitol (DTTred), while the oxidized form of the enzyme exhibits higher catalytic activity (Cseke et al., Citation1981). It was suggested that energized electrons from the photosynthetic system were transferred to G6PDH via thioredoxine which caused the reduction of disulphide bonds between –SH groups of intramolecular cysteine residues (Cossar et al., Citation1984; Gleason, Citation1996). Therefore, the enzyme is in a reduced hypoactive form in light and the OPP cycle is terminated due to blockage of the first step catalysed by G6PDH. On the other hand, there is no photosynthetic electron flow in the dark. The enzyme is oxidized due to losing electrons and converted into more active forms (Cossar et al., Citation1984; Gleason, Citation1994).
According to this scenario, at least two cysteine codons must be present in the G6PDH genes (zwf) of cyanobacterial strains. Pioneer cyanobacterial molecular genetic studies confirmed these cysteine codons in the zwf genes in conserved positions. There are two cysteine codons in the Synechococcus sp. PCC7942 zwf gene (Scanlan et al., Citation1992), four in Anabaena sp. PCC7120 (Newman et al., Citation1995) and three in Nostoc punctiforme ATCC29133 (Summers et al., Citation1995). The Synechocystis sp. PCC6803 zwf gene contains four cysteine codons at positions 101, 187, 265 and 445 (Kaneko et al., Citation1996). However, cysteine codon positions are not conserved in marine strains of cyanobacteria which contain more cysteine codons at different positions such as 23, 60, 144, 184, 300 and 301 (Rocap et al., Citation2003; Palenik et al., Citation2006). Theoretically, redox modulation through disulphide bonds is possible, even though cysteine codon positions of zwf are not conserved in cyanobacterial freshwater and marine strains.
Plant G6PDH has also been reported to be regulated through redox modulation (Anderson et al, Citation1978; Hauschild & von Schaewen, Citation2003). A site-directed mutagenesis study on six cysteine codons of plastidic isoforms of G6PDH in potatoes revealed that redox sensitivity of the enzyme was lost when cysteines at two positions (149 and 157) were changed to serine (Wenderoth et al., Citation1997). Other researchers reported that the cytosolic isoform was not redox sensitive (Graeve et al., Citation1994). Based on 3-D modelling analyses, the plastidic isoform of Arabidopsis thaliana G6PDH was also reported to be regulated by disulphide bond formation/reduction between cysteines, at positions 99 and 107 (Nee et al., Citation2009). In plastidic isoforms, all the cysteines are located near the N-terminus of the NADP-binding domain, whereas they are sparsely distributed throughout the polypeptide both in cyanobacterial and cytosolic isoforms. Phylogenetic analyses indicate that the plant cytosolic isoform and the red and green algal isoforms comprise a single group, while cyanobacterial sequences occur as a separate group (Wendt et al., Citation1999). On the basis of these data, Wendt et al. (Citation1999) suggested that redox modulation of the plastidic isoform and cyanobacterial G6PDH may have evolved independently.
Guo et al. (Citation2014), in their study on purified recombinant Synechocystis sp. PCC6803 G6PDH, reported that the enzyme lost redox sensitivity when the cysteines at the positions of 187, 265 and 445 were changed to serine. However, the effectors such as G6P, NADP, various types of thioredoxins, ribulose-1,5-bisphosphate (RuBP), glutamine and other proteins available in cyanobacterial cells were not analysed in this study. The authors suggested further studies to clarify the role of redox modulation in the regulation of G6PDH.
In this study, we changed four cysteine codons in the Synechocystis sp. PCC6803 zwf gene to serine codons separately, and each cysteine-mutant zwf gene was transferred back to the Synechocystis sp. PCC6803 chromosome, yielding zwf mutants, each with a mutation at a certain cysteine position. G6PDH activity in cell-free extracts of these cysteine-mutant Synechocystis sp. PCC6803 strains was not consistent with the results found in the purified recombinant enzyme preparations.
Materials and methods
Bacterial strains and growth conditions
Escherichia coli DH5α strain was used as the host strain for cloning studies, and the cells were made competent by the Inoue method (Sambrook & Russell, Citation2001). LB and 2xYT media were used for growth of E. coli DH5α cells. Ampicillin or kanamycin was added to the culture medium at a concentration of 50 μg ml–1 where applicable (Sambrook & Russell, Citation2001).
BG11 medium was used to grow wild-type and mutant strains of Synechocystis sp. PCC6803 cells while Anabaena sp. PCC7120 cells were grown in BG110 which is BG11 without NaNO3 (Rippka, Citation1988). Liquid cultures were incubated (New Brunswick Innova42, Eppendorf, Hamburg, Germany) at 28°C in 40 µmol photons m−2 s−1 light intensity at 90 rpm. Cysteine-mutants were grown in BG11 medium supplemented with 50 µg ml–1 kanamycin. The bacterial strains used in this study are listed in .
Table 1. Bacterial strains and plasmids used in this study.
Primers
The primers used in this study for mutagenesis, sequencing and PCR amplification are listed in .
Table 2. The oligonucleotide primers used in this study.
Cloning procedures
Restriction analysis, ligation and transformation were performed according to standard molecular biology techniques (Sambrook & Russell, Citation2001). A series of cloning and subcloning studies were carried out to yield plasmid clones ().
A StuI site was created downstream of the zwf gene to insert a kanamycin resistant gene (npt) for selection of cysteine mutant cells. The AAGCCT sequence downstream of the zwf gene of Synechocystis sp. PCC6803 that was previously cloned as pSG37 (Karakaya et al., Citation2008) was changed to a StuI recognition site (AGGCCT) by means of the overlap-extension method (Horton & Pease, Citation1991) using the Stumut 1, 2, 3 and 4 primers (). The amplicon of overlap-extension was then double digested with EcoRI/XbaI and a 566 bp fragment containing a StuI site was cloned into EcoRI/XbaI-digested pUC19, which yielded 3225 bp pSG40. This clone was used for insertion of the npt cassette downstream of the zwf gene. Therefore, a npt fragment of 1149 bp was amplified with KanF and KanR primers () using pSG37 as the template. Amplified npt fragments were then ligated into StuI-digested pSG40, which yielded 4381 bp pSG43.
To allow for common restriction endonuclease uniqueness, some additional subcloning studies were carried out. The 3.7 kb zwf fragment in pSG37 was isolated after digestion with NotI/SacI and then ligated into the NotI/SacI-digested pGEM-T Easy vector (Promega, Madison, USA), which yielded 6702 bp pSG39. To insert the npt cassette downstream of the zwf gene, pSG39 was double digested with XbaI/PacI, and the fragment carrying the npt cassette was isolated from pSG43 and ligated into XbaI/PacI-digested pSG39, which yielded 7858 bp pSG45. The resulting pSG45 plasmid carries a zwf fragment with an npt cassette downstream of the zwf gene (). This manipulated zwf fragment was used for positive selection of cysteine mutants against kanamycin after insertion of the mutations into the chromosomal DNA of ∆zwf mutant Synechocystis sp. PCC6803.
Exchange of cysteine codons of the zwf gene to serine codons
The zwf gene in pSG45 was used to change cysteine codons at positions 101, 187, 265 and 445 to serine codons. The inverse PCR method (Ochman et al., Citation1989) was used for site-directed mutagenesis of the cysteine codons. Mutagenic primer pairs were designed for each cysteine position: 101F and 101R for cys101, 187F and 187R for cys187, 265F and 265R for cys265 and 445F and 445R for cys445 (). Long PCR enzyme mix (Thermo Fisher Scientific, Waltham, USA) was used as a thermostable DNA polymerase in the inverse PCR reactions. Inverse PCR reactions were carried out for each cysteine position in the zwf gene. To remove methylated and hemi-methylated PCR products, the inverse PCR amplicons were digested with DpnI before transferring the unmethylated mutant PCR products into E. coli DH5α cells by transformation. Transformant cells were selected against kanamycin resistance conferred by the inverse PCR product of pSG45. The plasmids carrying cysteine/serine change mutations were isolated and named as pCS101, pCS187, pCS265 and pSC445 (). The zwf genes in the plasmids were sequenced in their entirety using the primers ZWF6803F1, ZWF6803F2, ZWF6803RA and ZWF6803RB (Macrogen Inc., Amsterdam, the Netherlands) (). The sequences were analysed with bioinformatics packet Mega7 (Kumar et al., Citation2016), and the mutations were determined to be in their expected positions.
Transformation of cysteine-mutant zwf fragments into ∆zwf mutant Synechocystis sp. SZD1 cells
Plasmids carrying the cysteine-mutant zwf gene with the npt cassette were transferred to the ∆zwf mutant SZD1 cells according to Zang et al. (Citation2007). Transformant cultures were then incubated on the surface of BG11 agarose plates. After 18–24 hours of incubation at 28°C under continuous light, 10 µg ml–1 kanamycin was added underneath the solid BG11 medium for positive selection of the transformant cells. When the colonies were seen on the media, several randomly selected colonies were transferred to fresh solid BG11 medium supplemented with 25 µg ml–1 kanamycin and incubated as previously described. This procedure was repeated three times consecutively.
The colonies that were still growing at the end of the procedure were transferred to BG11 liquid medium supplemented with 25 µg ml–1 kanamycin. As the cultures were growing, new cultures were started, but supplemented with gradually increasing amounts of kanamycin (25–50 µg ml–1). The cultures that were able to grow in 50 µg ml–1 kanamycin were taken as cysteine mutants and designated ZWFCS101, ZWFCS187, ZWFCS265 and ZWFCS445. Each cysteine-mutant strain was tested for the correct insertion of the mutations in the chromosomal DNA and for segregation of mutant chromosomes by PCR amplification. Therefore, chromosomal DNA was prepared from wild-type and the mutant strains as described by Lind et al. (Citation1985) and the 4.85 kb zwf fragment carrying the zwf gene and the flanking parts was amplified using the primers Fzwf and Rzwf (). The zwf gene regions of the PCR products were sequenced in their entirety for confirmation of the mutation in the chromosome.
Preparation of cell-free extracts and measurement of glucose-6-phosphate dehydrogenase activity
One litre cultures of wild-type and cysteine mutant Synechocystis sp. PCC6803 strains were grown in BG11 medium in a 5 l flask at 28°C under continuous light until cultures reached the late logarithmic phase. Cell-free extracts from the wild type and cysteine mutant cultures were prepared as described previously (Özkul & Karakaya, Citation2015).
The amount of protein in the enzyme solution was determined by the protein-dye binding method according to Bradford (Citation1976) by using a Micro Protein 1 Kit (Sigma-Aldrich, St Louis, USA). Measurement of enzyme activity was performed according to Özkul & Karakaya (Citation2015). A unit of enzyme activity was defined as formation of 1 µmol NADPH in 1 minute. Specific activity was expressed as units per mg protein.
Dithiothreitol treatment of G6PDH was carried out according to the studies performed previously (Cossar et al., Citation1984; Wenderoth et al., Citation1997). Assays were performed at room temperature using Tris-maleate buffer (pH 7.5) deoxygenized by N2 treatment. DTTred (Sigma Aldrich, St Louis, USA) was applied to the enzyme solution at the final concentrations of 10, 20 and 50 mM before assaying the activity. Each concentration of DTTred was applied to the enzyme solution for 10, 20 and 30 minutes. The assay was performed using 200 µg of protein containing the DTT-treated enzyme solution. Activity measurement was repeated three times for each case and the statistical significance of the results was determined using SPSS software (Release 17 Chicago, USA).
Results
Sequencing of mutant zwf genes and confirmation of cysteine mutations in pSG- plasmids
Each of the pSG101, pSG187, pSG265 and pSG445 plasmids containing cysteine/serine codon changes in corresponding positions was created by inverse PCR (see Methods section and ). The TGC cysteine codons at positions 101 and 187 were changed to TCC serine codons, and TGT cysteine codons at positions 265 and 445 were changed to TCT serine codons by inserting a G/C transversion mutation at the second position of the codons. The codon usage ratio of Synechocystis sp. PCC6803 was taken into consideration in serine codon preference (Kaneko et al., Citation1996). The full-length zwf gene in each plasmid was sequenced to detect any undesired mutations during inverse PCR reactions. Sequencing results confirmed that no undesired mutation was occurred.
Integration of cysteine-mutant zwf fragments into the SZD1 genome
Synechocystis sp. PCC6803 cells have the ability to take foreign DNA into the cell spontaneously and integrate it into the chromosome (Kufryk et al., Citation2002; Zang et al., Citation2007). Therefore, cysteine-mutant zwf genes in pSG101, 187, 265 and 445 were transformed into the ∆zwf mutant SZD1 cells of Synechocystis sp. PCC6803. Insertions of all of the plasmids contained part of the cysK gene, the zwf gene, the (npt) cassette and part of uvrA gene, except that each carries a point mutation in a certain position. In the SZD1 chromosome, a 2 kb omega interposon conferring the spectinomycin resistant gene (aadA) was replaced with a 1.25 kb region of the zwf gene including the cysteine codons (Karakaya et al., Citation2008). Other parts of the region were conserved in both the cysteine-mutant fragments and the zwf region of the SZD1 chromosome ().
Fig. 2. Comparison of the zwf fragments of (a) the plasmids pCS101, pCS187, pCS265, pCS445and pSG45 with (b) the zwf region of the chromosome of SZD1 cells.
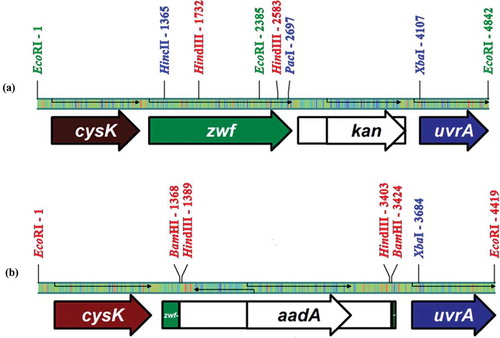
Once the plasmids were found to carry the mutation in the targeted positions, ∆zwf mutant SZD1 cells were transformed for each plasmid separately. A double recombination is required between the plasmid and chromosomal copy of the zwf region through two crossovers upstream and downstream of the zwf gene. Thus, the cysteine-mutant zwf gene, together with the npt gene, was expected to replace the omega fragment in the SZD1 chromosome.
To confirm the precise integration of cysteine mutations into the SZD1 chromosome, zwf regions of ZWFCS101, 187, 265 and 445 cells were amplified using Fzwf and Rzwf as primers () and chromosomal DNA from each strain as template. The amplified zwf gene regions of the ZWFCS101, 187, 265 and 445 chromosomes were 4847 bp in length. The fragments were cut by HindIII at two recognition sites which yielded three fragments of the following lengths: 2264 bp, 1732 bp and 851 bp. HindIII restriction fragment analysis confirmed that all of the strains contained the expected fragment, which indicated that cysteine-mutant zwf genes were integrated into the chromosome successfully ().
Fig. 3. HindIII restriction fragment lengths of the amplicons of the zwf gene regions of the mutant and wild-type strains of Synechocystis sp. PCC6803. Lines 1–6 are the restriction fragments of ZWFCS101, ZWFCS187, ZWFCS265, ZWFCS445 and SG45, respectively. Line 7 is SZD1 and line 8 is wild-type Synechocystis sp. PCC6803 HindIII fragments. Line 9 is an uncut 4847 zwf amplicon of SG45. Lines 1 and 10 are DNA markers (1 kb DNA ladder, Promega, Madison, USA).
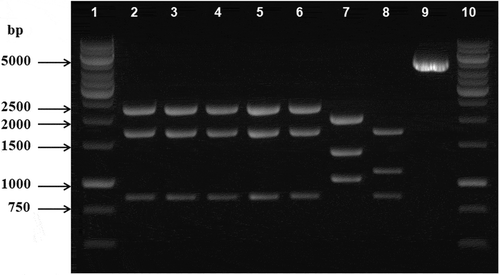
Also, the zwf region of each mutant strain was amplified and the entire length of the zwf was sequenced again. Sequencing data confirmed that the mutations were integrated into the correct positions.
Determination of the effect of reduced DTT on cysteine-mutant G6PDH activity
Wild-type Synechocystis sp. PCC6803, Anabaena sp. PCC7120 and E. coli DH5α cells were used as control groups for assaying G6PDH activity and effects of DTTred on G6PDH activity. Cell-free extracts were prepared from cysteine-mutant and control strains and used as enzyme solutions. At first, G6PDH activity of each strain was assayed under the following conditions: 2 mM G6P, 40 μM NADP and cell-free extract containing 200 μg protein.
Initially, G6PDH activity was assayed in Synechocystis sp. PCC6803 and SG45 strains to determine whether the (npt) gene inserted downstream of zwf affected G6PDH activity in SG45 and cysteine-mutant strains. Assay results demonstrated that there was no difference between G6PDH activities of either strain (data not shown).
G6PDH activities were measured in cell-free extracts of the cysteine mutant and control strains without DTTred treatment. These activities were designated normal activities. Certain levels of G6PDH activities were measured in all the strains except for ZWFCS187, which exhibited no G6PDH activity (). Optimum DTTred concentration and treatment time were found as 50 mM and 30 min, respectively (data not shown). To determine the effect of DTTred on wild-type and cysteine-mutant G6PDHs, 50 mM DTTred was applied to the extracts for 30 min immediately before measurement of G6PDH activity. DTTred treated cell-free extracts were then assayed for G6PDH activity (). G6PDH activity of wild-type Synechocystis sp. PCC6803 and Anabaena sp. PCC7120 decreased 34.9% and 36.8%, respectively. Cysteine-mutant G6PDHs of the ZWFCS101, ZWFCS265 and ZWFCS445 strains demonstrated 44.8%, 61.8% and 62.4% less than their corresponding untreated activities, respectively (). DTTred treatment on cyanobacterial G6PDH in cell-free extracts of both wild-type and cysteine-mutant strains decreased the activity significantly, indicating cysteine mutants did not behave differently than wild-type. G6PDH of E. coli DH5α was not affected by DTTred treatment as expected because of the heterotrophic nature of its metabolism.
Fig. 4. Effect of DTTred on G6PDH activity in cell-free extracts of the mutant strains, ZWFCS101, ZWFCS265 and ZWFCS445. Synechocystis sp. PCC6803 and Anabaena sp. PCC7120 are control groups for photosynthetic metabolism and E. coli DH5α for heterotrophic metabolism. Dotted bars represent G6PDH activity without DTTred treatment while black bars represent G6PDH activity after treatment with 50 mM DTTred for 30 minutes. Each bar expresses the mean of three independent measurements.
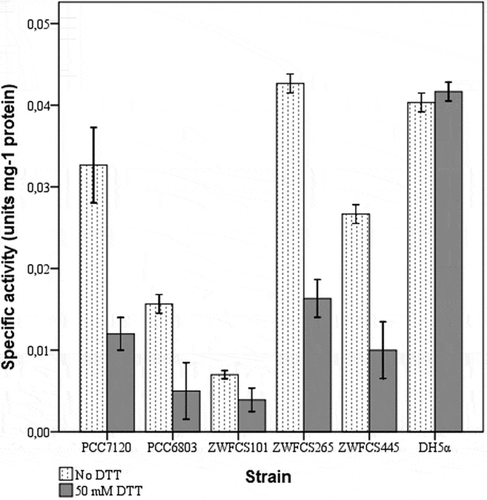
Discussion
The results of the current study indicate that a cysteine/serine change in G6PDH has no effect on the redox sensitivity of the enzyme. Three of the four mutant enzymes were affected by DTTred, similar to the wild-type enzyme. Therefore, loss of cysteine residues in positions 101, 265 and 445 did not affect redox sensitivity of the enzyme. The reduction rates of the mutant G6PDHs were comparable to the wild-type activities of Synechocystis sp. PCC6803 and Anabaena sp. PCC7120. These data indicate that the formation/reduction of a disulphide bond between –SH groups of internal cysteine residues of Synechocystis sp. PCC6803 G6PDH is not solely a light/dark regulation mechanism. Guo et al. (Citation2014) reported that cysteine/serine mutation decreased G6PDH activity more than four-fold in pure preparations. Although both studies were conducted on the same organism, Synechocystis sp. PCC6803, the results differ significantly. The only experimental difference is the degree of enzyme solution purity. We assayed enzyme activity in cell-free extracts while Guo et al. (Citation2014) utilized pure recombinant enzyme preparations supplemented with thioredoxin. This implies that sensitivity of the enzyme to reducing agents is affected by other metabolites in the cell, such as G6P, NADP/NADPH, glutamine and pH. The opcA polypeptide has long been suggested to be responsible for the redox sensitivity of the enzyme. Recombinant G6PDH pure enzyme of Nostoc punctiforme ATCC29133 loses redox inactivation and reactivation when OpcA is absent (Hagen & Meeks, Citation2001). Similarly, based on the study of light/dark modulation of thiol oxidation in Synechocystis sp. PCC6803, Guo et al. (Citation2014) suggested that Zwf alone might not sensitive to general redox status, and cysteines inside the polypeptide may be functionally involved in a Zwf/OpcA interaction, facilitating redox regulation. G6PDH of Anabaena sp. PCC7120 is also a well-known redox modulated enzyme (Gleason, Citation1996). Recently Mihara et al. (Citation2018) found that G6PDH activity of Anabaena sp. PCC7120 was regulated by the redox states of OpcA rather than the Zwf polypeptide itself. Analyses of the OpcA polypeptide indicated that Cys393 and Cys399 of OpcA were responsible for the redox regulation. This result is consistent with our suggestion that internal disulphide bonds are not the sole mechanism for redox regulation of G6PDH of cyanobacteria.
The ZWFCS187 mutant exhibited no G6PDH activity and the results of this study do not explain such a complete loss of activity. Nevertheless, some predictions are proposed. The cysteine residue at position 187 is only seven residues away from the active site of the enzyme. A cysteine/serine change close to the active site may affect the active conformation of the enzyme resulting in a total loss of catalytic activity. However, such a mutant was reported to have enzyme activity by Guo et al. (Citation2014). Therefore, the mutation point of the zwf gene of ZWFCS187 was further analysed to check for experimental mistakes. Eventually, the zwf region of the chromosome of ZWFCS187 was amplified and sequenced twice and the expected sequence of the mutation position was confirmed. The reasons for this inconsistency remain unclear.
Similar research was also carried out on plant G6PDHs. Site-directed mutagenesis studies on potato G6PDH showed that the plastidic isoform lost redox sensitivity when two of the six cysteine residues, in certain positions, were changed to serine. However, the cytosolic isoform was not affected by any of the cysteine to serine changes (Wenderoth et al., Citation1997). The positions of the six cysteine residues in both the cytosolic and plastidic isoforms are completely different. Cysteine residues in the plastidic isoform are clustered in the NADP-binding domain of the protein, while those of the cytosolic isoform are sparsely distributed throughout the protein. Moreover, cyanobacterial G6PDHs do not possess structural similarity in cysteine positions to plastidic G6PDH, which is the only G6PDH isoform in which redox modification has been shown to be a mechanism for light regulation.
Although cysteine residues are represented in cyanobacterial G6PDH as potential disulphide bond targets, comparison of cysteine number and positions in freshwater and marine strains raises some questions. First of all, G6PDH of freshwater strains possess two to four cysteines and only two of them are conserved in most strains, at positions 187 and 445. A cysteine to serine exchange at position 187 causes a complete loss of catalytic activity. Such a change at position 445 does not affect either catalytic activity or redox sensitivity. Additionally, G6PDHs of marine cyanobacterial strains exhibit a completely different cysteine distribution to freshwater strains, and possess six cysteine residues in conserved positions. This implies that a common disulphide bond-formation/reduction mechanism is not present in cyanobacterial groups. Moreover, Nee et al. (Citation2009) suggested that cysteine residues credited with forming a disulphide bond might play a role in keeping the enzyme in an active conformation, rather than disulphide bond formation. Therefore, it may be concluded that the cysteine residue at position 187 in G6PDH of Synechocystis sp. PCC6803 plays a role in keeping the active site of the enzyme in the correct position rather than forming a disulphide bond with the cysteine at position 445.
The presence of the light that initiates photosynthetic operation is only one metabolic regulation factor in photosynthetic organisms. Other factors also affect enzyme activity in photosynthesizing cells: substrate and co-factor concentrations, ATP, RuBP, redox potential of the cell and pH changes (Schaeffer & Stanier, Citation1978; Gleason, Citation1996). In this study, cysteine-mutant zwf genes were separately integrated into the Synechocystis sp. PCC6803 chromosome to express G6PDH in its natural environment. It was observed that the redox sensitivity of the mutant enzymes was not affected in cell-free extracts. Consequently, our results indicated that the whole cell is involved in the activity state of an enzyme, and a key step such as activation/deactivation of G6PDH is a result of the sum of various factors and mechanisms, rather than the formation or reduction of a single disulphide bond.
Author contributions
K. Özkul: Original concept, experimental design and application, analyses of data, drafting and editing manuscript.H.Karakaya: Original concept, experimental design, analyses of data, drafting and editing manuscript.
Acknowledgements
We are grateful to Dr Kamil Alakuş of Ondokuz Mayıs University for statistical analyses.
Disclosure statement
No potential conflict of interest was reported by the authors.
Additional information
Funding
References
- Anderson, L.E., Nehrlich, S.C. & Champigny, M. (1978). Light modulation of enzyme activity. Activation of the light effect mediator by reduction and modulation of enzyme activity by thiol-disulphide exchange. Plant Physiology, 61: 601–605.
- Bradford, M.M. (1976). A rapid and sensitive method for the quantitation of microgram quantities of protein utilizing the principle of protein-dye binding. Analytical Biochemistry, 72: 248–254.
- Cossar, J.D., Rowell, P. & Steward, D.P. (1984). Thioredoxin as a modulator of glucose-6-phosphate dehydrogenase in a N2-fixing cyanobacterium. Journal of General Microbiology, 130: 991–998.
- Cseke, C., Balogh, A. & Farkas, G.L. (1981). Redox modulation of glucose-6-phosphate dehydrogenase in Anacytis nidulans and its uncoupling by phage infection. FEBS Letters, 126: 85–88.
- Gleason, F.K. (1994). Thioredoxin in cyanobacteria: structure and redox regulation of enzyme activity. In The Molecular Biology of Cyanobacteria (Bryant, D.A., editor), 714–729. Kluwer, Dordrecht.
- Gleason, F.K. (1996). Glucose-6-phosphate dehydrogenase from the cyanobacterium, Anabaena sp. PCC 7120: purification and kinetics of redox modulation. Archives of Biochemistry and Biophysics, 334: 277–283.
- Graeve, K., von Schaewen, A. & Scheibe, R. (1994). Purification, characterization, and cDNA sequence of glucose-6-phosphate dehydrogenase from potato (Solanum tuberosum L.). Plant Journal, 5: 353–361.
- Grossman, A. & McGowan, R.E. (1975). Regulation of glucose-6-phosphate dehydrogenase in blue-green algae. Plant Physiology, 55: 658–662.
- Guo, J., Nguyen, A.Y., Dai, Z., Su, D., Gaffrey, M.J., Moore, R.J., Jacobs, J.M., Monroe, M.E., Smith, R.D., Koppenaal, D.W., Pakrasi, H.B. & Qian, W.J. (2014). Proteome-wide light/dark modulation of thiol oxidation in cyanobacteria revealed by quantitative site-specific redox proteomics. Molecular and Cellular Proteomics, 13: 3270–3285.
- Hagen, K.D. & Meeks, J.C. (2001). The unique cyanobacterial protein OpcA is an allosteric effector of glucose-6-phosphate dehydrogenase in Nostoc punctiforme ATCC 29133. Journal of Biological Chemistry, 276: 11477–11486.
- Hauschild, R. & von Schaewen, A. (2003). Differential regulation of glucose-6-phosphate dehydrogenase isoenzyme activities in potato. Plant Physiology, 133: 47–62.
- Horton, R.M. & Pease, L.R. (1991). Recombination and mutagenesis of DNA sequences using PCR. In Directed Mutagenesis: A Practical Approach (McPherson, M.J., editor), 217–247. IRL Press, Oxford.
- Kaneko, T., Sato, S., Kotani, H., Tanaka, A., Asamizu, E., Nakamura, Y., Miyajima, N., Hirosawa, M., Sugiura, M., Sasamoto, S., Kimura, T., Hosouchi, T., Matsuno, A., Muraki, A., Nakazaki, N., Naruo, K., Okumura, S., Shimpo, S., Takeuchi, C., Wada, T., Watanabe, A., Yamada, M., Yasuda, M. & Tabata, S. (1996). Sequence analysis of the genome of the unicellular cyanobacterium Synechocystis sp. strain PCC6803. II. Sequence determination of the entire genome and assignment of potential protein coding regions. DNA Research, 3: 109–136, 185–209.
- Karakaya, H., Ay, M.T., Ozkul, K. & Mann, N.H. (2008). A Δzwf (glucose-6-phosphate dehydrogenase) mutant of the cyanobacterium Synechocystis sp. PCC 6803 exhibits unimpaired dark viability. Annals of Microbiology, 58: 281–286.
- Kufryk, I.G., Sachet, M., Schmetterer, G. & Vermaas, W.F.J. (2002). Transformation of the cyanobacterium Synechocystis sp. PCC 6803 as a tool for genetic mapping: optimization of efficiency. FEMS Microbiology Letters, 206: 215–219.
- Kumar, S., Stecher, G. & Tamura, K. (2016). MEGA7: Molecular Evolutionary Genetics Analysis version 7.0 for bigger datasets. Molecular Biology and Evolution, 33: 1870–1874.
- Lubberding, H.J. & Bot, P.V.M. (1984). The influence of temperature on glucose-6-phosphate dehydrogenase and 6-phosphogluconate dehydrogenase and the regulation of these enzymes in a mesophilic and a thermophilic cyanobacterium. Archives of Microbiology, 137: 115–120.
- Lind, L.K., Kallas, S.R., Lonneborg, A., Oquist, G. & Guastafssan, P. (1985). Cloning of the β-phycocyanin gene from Anacystis nidulans. FEBS Letters, 188: 27–32.
- Mihara, S., Wakao, H., Yoshida, K., Higo, A., Sugiura, K., Tsuchiya, A., Nomata, J., Wakabayashi, K. & Hisabori, T. (2018). Thioredoxin regulates G6PDH activity by changing redox states of OpcA in the nitrogen-fixing cyanobacterium Anabaena sp. PCC 7120. Biochemical Journal, 475: 1091–1105.
- Nee, G., Zaffagnini, M., Trost, P. & Issakidis-Bourguet, E. (2009). Redox regulation of chloroplastic glucose-6-phosphate dehydrogenase: a new role for f-type thioredoxin. FEBS Letters, 583: 2827–2832.
- Newman, J., Karakaya, H., Scanlan, D.J. & Mann, N.H. (1995). A comparison of gene organization in the zwf region of the genomes of the cyanobacteria Synechococcus sp PCC 7942 and Anabaena sp. PCC 7120. FEMS Microbiology Letters, 133: 187–193.
- Ochman, H., Ajioka, J.W., Garza, D. & Hartl, D.L. (1989). Inverse polymerase chain reaction. In PCR Technology: Principles and Applications for DNA Amplification (Erlich, H.A., editor), 105–111. Stockton Press, New York.
- Özkul, K. & Karakaya, H. (2015). Characterisation of an opcA mutant of the unicellular cyanobacterium Synechocystis sp. PCC 6803. Current Microbiology, 71: 572–578.
- Palenik, B., Ren, Q., Dupont, C.L., Myers, G.S., Heidelberg, J.F., Badger, J.H., Madupu, R., Nelson, W.C., Brinkac, L.M., Dodson, R.J., Durkin, A.S., Daugherty, S.C., Sullivan, S.A., Khouri, H., Mohamoud, Y., Halpin, R. & Paulsen, I.T. (2006). Genome sequence of Synechococcus CC9311: insights into adaptation to a coastal environment. Proceedings of the National Academy of Sciences of the USA, 103: 13555–13559.
- Rippka, R. (1988). Isolation and purification of cyanobacteria. In Methods of Enzymology (Parker, L. & Glazer, A.N., editors), 3–27. Academic Press, San Diego, California.
- Rippka, R., Deruells, J., Waterbury, J.B., Herdman, M. & Stainer, R.Y. (1979). Generic assignment, strain histories and properties of pure cultures of cyanobacteria. Journal of General Microbiolology, 111: 1–61.
- Rocap, G., Larimer, F.W., Lamerdin, J., Malfatti, S., Chain, P., Ahlgren, N.A., Arellano, A., Coleman, M., Hauser, L., Hess, W.R., Johnson, Z.I., Land, M., Lindell, D., Post, A.F., Regala, W., Shah, M., Shaw, S.L., Steglich, C., Sullivan, M.B., Ting, C.S., Tolonen, A., Webb, E.A., Zinser, E.A. & Chisholm, S.W. (2003). Genome divergence in two Prochlorococcus ecotypes reflects oceanic niche differentiation. Nature, 424: 1042–1047.
- Sambrook, J. & Russell, D.W. (2001). Molecular Cloning: A Laboratory Manual. 3rd ed. Cold Spring Harbor Laboratory Press, Cold Spring Harbor, New York.
- Scanlan, D.J., Newman, J., Sebaihia, M., Mann, N.H. & Carr, N.G. (1992). Cloning and sequence analysis of the glucose-6-phosphate dehydrogenase gene from the cyanobacterium Synechococcus PCC 7942. Plant Molecular Biology, 19: 877–880.
- Schaeffer, F. & Stanier, R.Y. (1978). Glucose-6-phosphate dehydrogenase of Anabaena sp. kinetic and molecular properties. Archives of Microbiology, 116: 9–19.
- Smith, A.J. (1982). Modes of cyanobacterial carbon metabolism. In The Biology of Cyanobacteria (Carr, N.G. & Whitton, B.A., editors), 47–85. Blackwell, Oxford.
- Summers, M.L., Meeks, J.C., Chu, S. & Wolf, R.E. (1995). Nucleotide sequence of an operon in Nostoc sp. strain ATCC 29133 encoding four genes of oxidative pentose phosphate cycle. Plant Physiology, 107: 267–268.
- Udvardy, J., Juhasz, A. & Farkas, G. (1983). Interaction between hysteretic regulation and redox modulation of glucose-6-phosphate dehydrogenase from Anacystis nidulans. FEBS Letters, 152: 97–100.
- Wenderoth, I., Scheibe, R. & Schaewen, A. (1997). Identification of the cysteine residues involved in redox modification of plant plastidic glucose-6-phosphate dehydrogenase. Journal of Biological Chemistry, 272: 26985–26990.
- Wendt, U.K., Hauschild, R., Lange, C., Pietersma, M., Wenderoth, I. & von Schaewen, A. (1999). Evidence for functional convergence of redox regulation in G6PDH isoforms of cyanobacteria and higher plants. Plant Molecular Biology, 40: 487–494.
- Yanisch-Peron, C., Vieira, J. & Messing, J. (1985). Improved M13 phage cloning vectors and host strains: nucleotide sequence of the M13 mp18 and pUC19 vectors. Gene, 33: 103–119.
- Zang, X., Liu, B., Liu, S., Arunakumara, K.K.I.U. & Zhang, X. (2007). Optimum conditions for transformation of Synechocystis sp. PCC 6803. Journal of Microbiology, 45: 241–245.