ABSTRACT
Cyanobacteria secrete exopolysaccharide (EPS), which can be intimately associated with the cell surfaces (known as capsular polysaccharide, CPS), or released into the surrounding environment (released polysaccharide, RPS). The aim of this study was to explore the role of EPS in cell aggregation and biofilm formation in the unicellular cyanobacterium Synechocystis sp. strain PCC 6803. Three mutants (Δsll5043, Δslr1063 and Δslr1076) were obtained through disrupting three putative glycosyltransferase genes (sll5043, slr1063 and slr1076) separately. Decreased contents and altered monosaccharide composition of both RPS and CPS were observed in the three mutants compared with the wild type (WT). RPS from the WT (WT-RPS) had high emulsification activity, while RPSs from the mutants had no emulsification activity. Unlike the WT, the three mutants formed cell aggregates and biofilms. Compared with the WT, the three mutants possessed lower cell hydrophobicity and less negative cell surface charge, which could result from the altered production of CPS. 40 μg ml–1 WT-RPS significantly decreased cell hydrophobicity of the three mutants, and significantly inhibited cell aggregation and biofilm formation, while the RPS from each mutant itself had no effect on cell hydrophobicity of the mutant, and did not affect cell aggregation and biofilm formation. The inhibitory effect of WT-RPS on cell aggregation and biofilm formation was thus suggested to be related to its ability to reduce cell hydrophobicity. The difference in cell aggregation and biofilm formation between the WT and the three mutants could be related to differences in cell hydrophobicity, cell surface charge and physicochemical properties of RPS. Our results in this study indicated that both CPS and RPS affected cell aggregation and biofilm formation in Synechocystis sp. strain PCC 6803, and WT-RPS inhibited cell aggregation and biofilm formation.
Introduction
Biofilms are social consortia of microorganisms, encased in an extracellular matrix, and attached to solid surfaces (McDougald et al., Citation2011). Cyanobacteria often form multicellular aggregates or biofilms constrained by a self-synthesized mucilaginous matrix in natural environments. Previous studies have demonstrated that cyanobacteria switch their lifestyles from a single-cell state to cell aggregate or biofilm in response to environmental stress conditions. Formation of multicellular colonies in the bloom-forming cyanobacterium Microcystis can be induced by biotic and abiotic factors, e.g. heterotrophic bacteria, zooplankton grazing, high Ca2+ concentration and low temperature (Xiao et al., Citation2018). A thermophilic cyanobacterium, Thermosynechococcus vulcanus RKN, exhibits cell aggregation to avoid excess light stress (Kawano et al., Citation2011). High salinity and changes in nutrient concentration induce biofilm formation in Synechocystis sp. strain PCC 6803 (hereafter Synechocystis 6803) (Kera et al., Citation2018; Allen et al., Citation2019).
Microalgal biofilms have recently received attention as an innovative cultivation technique for production of bioproducts (Mantzorou & Ververidis, Citation2019). This technique could resolve the drawbacks associated with suspended cultivation systems, such as high water requirements and difficulty in biomass harvesting and recovery. In biofilm-based cultivation systems, less water (about 1/10th) is required for cultivation of microalgae compared with traditional suspended cultivation systems (Katarzyna et al., Citation2015). Microalgal cells in biofilms are concentrated in a small footprint area and can easily be removed from the solid surfaces by scraping (Barros et al., Citation2015). In addition, there is a growing interest in the application of microalgal biofilms in wastewater treatment, bioremediation, soil improvement and aquaculture (Roeselers et al., Citation2008). It is therefore important to understand the mechanisms involved in biofilm formation not only for clarifying the physiological adaptation of cyanobacteria to harsh environmental conditions but also for potential biotechnological applications of cyanobacteria.
Information on the mechanisms underlying cell aggregation and biofilm formation in cyanobacteria is limited. Cell aggregation of T. vulcanus strain RKN was found to be due to accumulation of extracellular cellulose, and regulated by three cyanobacteriochromes (SesA, SesB and SesC) via cyclic di-GMP signalling (Enomoto et al., Citation2015; Maeda et al., Citation2018). Reduction of intracellular spermidine content increases biofilm formation in Synechocystis 6803 (Kera et al., Citation2018). The planktonic nature of Synechococcus elongatus results from the self-suppression of biofilm formation, which depends on a factor secreted into the extracellular milieu (Schatz et al., Citation2013).
Cyanobacteria synthesize and secrete exopolysaccharide (EPS), which can be intimately associated with the cell surfaces (capsular polysaccharide, CPS), or released into the surrounding environment (released polysaccharide, RPS) (Pereira et al., Citation2009). The genes putatively involved in the synthesis of cyanobacterial EPSs are clustered and located in different regions of the cyanobacterial genomes (Pereira et al., Citation2009). Based on the annotation in the CyanoBase genome database (Nakao et al., Citation2010) and the analysis performed by Pereira et al. (Citation2015), two gene clusters (sll5042-sll5060 and slr1063-slr1077) of Synechocystis 6803 are putatively involved in EPS synthesis. The genes in the sll5043-sll5060 cluster are predicted to encode glycosyltransferases, flippases, polysaccharide polymerases and export proteins. The proteins encoded by the genes in the slr1063-slr1077 cluster show similarity to glycosyltransferases and polysaccharide polymerases. In this study, three putative glycosyltransferase genes (sll5043, slr1063 and slr1076) were selected from the above two gene clusters, and three mutants were generated through disrupting the three genes separately. EPS production and the capacity to form cell aggregates and biofilms in the wild type (WT) and the mutants were analysed in order to explore the role of EPS in cell aggregation and biofilm formation in Synechocystis 6803.
Materials and methods
Strains and culture conditions
The unicellular cyanobacterium Synechocystis 6803 was obtained from the Freshwater Algae Culture Collection at the Institute of Hydrobiology of China. The cyanobacterium was cultured in BG11 medium (Rippka et al., Citation1979) at 25°C. BG11 medium supplemented with 50 μg ml–1 kanamycin sulphate was used for growth of mutant strains of Synechocystis 6803. The illumination of 40 μmol photons m−2 s−1 was provided by cool-white fluorescent lamps with a light: dark cycle of 14: 10 h. The cultures were shaken manually three times per day. The optical density of the culture at 730 nm (OD730) was determined by using a UV/Vis spectrophotometer (UV-1800, Mapada, China) to measure the cyanobacterial growth.
Escherichia coli (DH5α) used as the cloning host was grown at 37°C in Luria–Bertani (LB) broth. When appropriate, carbenicillin (100 μg ml–1) or kanamycin sulphate (50 μg ml–1) was added to LB medium.
Gene inactivation
Cyanobacterial genomic DNA was extracted using the xanthogenate-SDS (XS) DNA extraction method (Tillett & Neilan, Citation2000). The DNA fragments containing the studied genes and the flanking regions were amplified by PCR with specific primers (Supplementary table 1). Each DNA fragment was cloned into the pMD19-T vector (TaKaRa), and then disrupted with a cassette conferring kanamycin resistance in the same orientation as the targeted gene at a unique restriction site. The kanamycin resistance cassette was amplified from pHis2.1 with the primers Kanr F (5’-CGCGGAACCCCTATTTGTTTATTT) and Kanr R (5’-TGACGCTCAGTGGAACGAAAAC). The Klenow fragment was used to perform DNA blunting. All plasmids used and constructed in this study are listed in Supplementary table 2.
Synechocystis 6803 cells were transformed with the plasmids containing the gene disruptions by natural transformation (Zang et al., Citation2007). The kanamycin-resistant transformants were selected on the BG11 plates containing 50 μg ml–1 kanamycin sulphate. Complete chromosome segregation was confirmed by PCR on genomic DNA from the three mutants (Δsll5043, Δslr1063 and Δslr1076) using the primers in Supplementary table 3.
Extraction of EPS
Cyanobacterial cultures of late log phase (30 days) were centrifuged at 10 000 × g for 15 min at 4°C. The supernatant was further centrifuged at 30 000 × g for 30 min at 4°C to remove possible remaining cell particles. The resulting supernatant was concentrated to 10% of its initial volume under reduced pressure at 45°C, and then dialysed against distilled water using dialysis tubes with cutoff molecular weight of 3500 Da at 4°C for 72 h. After dialysis, the RPS samples were lyophilized for further characterization.
CPS was extracted with 3% glutaraldehyde solution (Li et al., Citation2009). Cyanobacterial cells harvested by centrifugation (10 000 × g, 15 min, 4°C) were re-suspended in 3% glutaraldehyde solution and incubated at 4°C overnight. After centrifugation at 10 000 × g for 15 min at 4°C, the supernatant containing CPS was sequentially filtered with 0.45- and 0.22-μm pore-size membranes. The filtrate was concentrated, dialysed and lyophilized as described above.
EPS content
Because EPS from each mutant was different from EPS from the WT, quantification of EPS by the phenol–sulphuric acid method (Dubois et al., Citation1956) was inappropriate for analysing the change of EPS content in the mutants. The EPS content was determined by weighing the lyophilized EPS and normalized by the cell counts. The cells were counted in a hemocytometer under an optical microscope (ECLIPSE E100, Nikon, Tokyo, Japan).
Monosaccharide composition of EPS
Uronic acid content was measured using the meta-hydroxydiphenyl method with glucuronic acid as reference (Blumenkr & Asboehan, Citation1973). The neutral monosaccharide composition of EPS was analysed by gas chromatography-mass spectrometry (GC-MS). Lyophilized EPS was subjected to hydrolysis with 2M trifluoroacetic acid for 2 h at 120°C. The hydrolysis product was evaporated to dryness. The neutral sugars in the hydrolysate were converted into acetylated aldononitrile derivative for GC-MS analysis (Hu et al., Citation2016). GC-MS runs were performed on 7890A gas chromatography (Agilent Technologies, Santa Clara, California, USA) interfaced to a 5977A mass spectrometer detector and an HP-5MS capillary column (30 m × 250 μm × 0.25 μm). The following temperature procedure was employed: column temperature was initially fixed at 110°C, then increased to 250°C at 4°C min–1 and held for 5 min at 250°C.
Emulsification activity of RPS
Emulsification activity of RPS was determined according to the method of Cooper & Goldenberg (Citation1987). 2 ml of olive oil was added to test tubes containing 2 ml of RPS solution at a concentration of 1.5 mg ml–1. The mixture was vigorously vortexed for 2 min and allowed to stand for 24 h. The emulsification index was calculated as follows: Emulsification index (%) = he ÷ ht × 100, where he refers to the height of the emulsion layer and ht refers to the total height.
Rheological analysis
The lyophilized RPS was dissolved in distilled water. The final concentration of RPS was 0.5 mg ml–1. The rheological measurement (viscometry) was performed at 20°C using a RheoStress 600 torque rheometer (HAAKE, Karlsruhe, Germany) equipped with cone and plate geometry (60 mm diameter, cone angle 1°). The flow curves were determined at 0.01 s−1 to 1000 s−1 shear rates.
Cell aggregation and biofilm formation assay
The three mutants obtained in this study were observed to form cell aggregates during cultivation in BG11 medium, but the cell aggregates switched completely to single cells during late log phase. The unicellular mutant cultures grown for 25 days were used to inoculate experiments of cell aggregation and biofilm formation assay. Cell aggregation was evaluated using the aggregation index (Kawano et al., Citation2011). After 10 days of growth, 4 ml of cyanobacterial culture was sampled and treated with ultrasonication at 80 W and 20 Hz for 1 min (JY92-IIN, Scientz, Ningbo, China) to disaggregate cell aggregates of the mutants. The OD730 of the resulting cell suspension was measured as ODtotal. Another 4 ml of cyanobacterial culture was sampled and transferred to a test tube. After standing for 30 min, the supernatant was gently pipetted from the test tube, and the OD730 was measured as ODNA. The aggregation index was calculated as follows: Aggregation index (%) = (ODtotal − ODNA) ÷ ODtotal × 100.
Quantification of biofilms was conducted according to Fisher et al. (Citation2013) with minor modifications. 2 ml of cyanobacterial culture with initial OD730 of 0.1 was cultivated statically in a test tube for 7 days. After the suspended fraction containing non-adherent cells was removed, test tubes were washed gently with distilled water. Each test tube was then stained with 2 ml of crystal violet (CV) solution (0.1%, w v–1) for 15 min. After removal of the staining solution, test tubes were washed three times with distilled water. The CV associated with the biofilm cells was dissolved in 10 ml of 95% ethanol. The optical density of the resulting CV solution at 560 nm (OD560) served as an estimate of biofilm formation.
To assess the effect of RPS on cell aggregation and biofilm formation, each mutant was grown in BG11 media supplemented with RPS from the WT (WT-RPS) and RPS from the mutant itself, respectively. Since our preliminary study found that lyophilization of WT-RPS significantly decreased its inhibitory effect on biofilm formation, the dialysed RPS solution was directly used. Carbohydrate content in the RPS solution was determined using glucose as a reference by the phenol–sulphuric acid method (Dubois et al., Citation1956). For the WT, Δsll504, Δslr1063 and Δslr1076, the actual polysaccharide content in the RPS solution was calculated by multiplying the measured carbohydrate content (expressed as glucose equivalent) with experimentally determined correction factors of 2.17, 4.57, 4.75 and 3.88, respectively. The correction factor was experimentally determined by dividing the polysaccharide content in the solution (measured by weighing the lyophilized polysaccharide) by the carbohydrate content (measured by the phenol–sulphuric acid method) in advance. Additionally, since treatment of WT-RPS at 121°C for 15 min was found to significantly reduce its biofilm-inhibiting activity, the RPS solution was autoclaved at 105°C for 15 min. After autoclave treatment, 100 μl of RPS solution was spread onto R2A agar plates (Reasoner & Geldreich, Citation1985) to ensure that microorganisms were absent in the RPS solution.
Effect of WT-RPS on dispersion of cell aggregate and biofilm of the mutants
After 10 days of growth, the mutant cultures were centrifuged at 1000 × g for 10 min and washed twice with fresh BG11 medium. The cell pellets were resuspended in BG11 medium supplemented with 40 μg ml–1 WT-RPS. The control without WT-RPS addition was carried out at the same time. After 2 days of incubation, the aggregation index was measured.
After 7 days of static growth, biofilms formed in test tubes were rinsed with BG11 medium, and incubated further in BG11 medium containing 40 μg ml–1 WT-RPS for 2 days. The control without WT-RPS addition was carried out at the same time. Biofilms were then quantified by CV staining.
Cell surface properties
After 10 days of growth, the cyanobacterial cultures were sampled. The mutant cultures, with aggregated cells, were disaggregated into single cells by ultrasonication as described above. The cell suspension was then centrifuged at 5000 × g for 10 min and washed twice with fresh BG11 medium. The cell pellets were resuspended in fresh BG11 medium. The zeta potentials of cyanobacterial cells were measured by the ZetaSize (Nano-Z, Marlvern, UK) after the cell suspension was diluted to OD730 ≈ 0.5. The cell surface hydrophobicity was measured according to the method of Fattom & Shilo (Citation1984). 2 ml of n-hexadecane was added to 5 ml of cell suspension with an OD730 of ≈ 0.8 (ODi). The mixture was vortexed for 1 min. After 10 min of equilibration, the OD730 of the lower aqueous phase (ODf) was measured. The hydrophobicity of cyanobacteria, expressed as the percentage of the applied cell suspension absorbance which had been excluded from the aqueous phase, was calculated using the following equation: Hydrophobicity (%) = (ODi – ODf) ÷ ODi × 100.
To assess the effect of RPS on cell hydrophobicity, the cell pellets harvested by centrifugation were resuspended in BG11 media supplemented with WT-RPS and RPSs from the mutants, respectively. The dialysed RPS solution was used directly.
Statistical analysis
All data are presented as mean ± standard deviation (SD) from three independent biological replicates. Data were analysed using a one-way analysis of variance (ANOVA) with Duncan post-hoc tests. The assumption of normal distribution and homoscedasticity were tested using Shapiro–Wilk and Levene’s tests, respectively. ANOVA test results are shown in Supplementary tables 4–9. A p value < 0.05 was considered significant.
Results
Changes of EPS content and physicochemical properties in the mutants defective in putative glycosyltransferases
The three mutants (Δsll5043, Δslr1063 and Δslr1076) were obtained through disrupting the three putative glycosyltransferase genes (sll5043, slr1063 and slr1076) separately in Synechocystis 6803 (Supplementary fig. 1). No significant difference in growth was found between each mutant and the WT (Supplementary fig. 2). The content of both RPS and CPS in each mutant was lower than that in the WT (). Compared with the WT, the RPS content of three mutants (Δsll5043, Δslr1063 and Δslr1076) declined by 31.8 ± 14.4%, 64.7 ± 7.5% and 88.7 ± 2.9%, respectively, and the CPS content of the three mutants declined by 72.6 ± 11.4%, 79.6 ± 3.7% and 74.1 ± 4.5%, respectively.
The monosaccharide composition of both RPS and CPS from three mutants differed from that from the WT (), with a lower proportion of rhamnose in total neutral sugars in both RPS and CPS, while the proportion of fucose in total neutral sugars in both RPS and CPS from each mutant was higher. The proportion of galactose in total neutral sugars in RPS from each mutant was lower than that from the WT. Compared with the WT, the uronic acid contents of CPSs from three mutants (Δsll5043, Δslr1063 and Δslr1076) decreased by 33.4 ± 3.4%, 22.0 ± 6.9% and 31.1 ± 11.6%, respectively, and the uronic acid contents of RPSs from three mutants decreased by 67.0 ± 0.6%, 44.8 ± 13.8% and 76.1 ± 3.8%, respectively.
Table 1. Monosaccharide composition of the exopolysaccharides from the WT and three mutants of Synechocystis 6803
The emulsification index of 1.5 mg ml–1 WT-RPS was 100%, but no emulsion activity was observed for 1.5 mg ml–1 RPS from each of the three mutants. The apparent viscosity of RPS from each mutant was lower than that of WT-RPS (Supplementary fig. 3).
Differences in cell aggregation, biofilm formation and cell surface properties between three mutants and the WT
When inoculated with unicellular cyanobacterial culture, cell aggregates started to appear in the culture of each mutant on the fifth to sixth day of incubation and disaggregated into single cells after 21–24 days of incubation. Mutant cultures grown for 10 days were rich in cell aggregates, with a high aggregation index (64.1–73.3%), while the culture of the WT contained only single cells ( and Supplementary figs 4–8) and when inoculated with a culture with cell aggregates, these disaggregated into single cells after 21–24 days of incubation.
Fig. 2. Quantification of (a) cell aggregates and (b) biofilms formed by the WT and the mutants of Synechocystis 6803
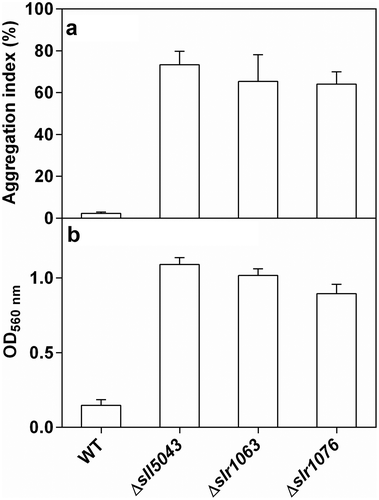
When inoculated with a unicellular cyanobacterial culture, biofilms of the three mutants started to appear in test tubes on days 3–4 of static cultivation, and dispersed completely after 20 days of incubation. shows that the mutants had high biofilm formation ability compared with the WT. Although the weak absorption of chlorophyll a might affect optical density measurements at 560 nm in , Supplementary fig. 9 highlights biofilm formation which was observed in the mutants after 7 days of static cultivation, while the biofilm was scarcely formed in WT. Differences in cell surface properties were found between the three mutants and the WT. Compared with the WT, mutant cells possessed less negative zeta potential, and the cell hydrophobicity of the three mutants (Δsll5043, Δslr1063 and Δslr1076) decreased by 13.7 ± 3.1%, 26.7 ± 5.2% and 28.0 ± 5.9%, respectively ().
Influence of WT-RPS on cell aggregation, biofilm formation and cell hydrophobicity in the three mutants
40 μg ml–1 WT-RPS significantly inhibited cell aggregation and biofilm formation of the three mutants, while the RPS from each mutant itself had no effect on cell aggregation and biofilm formation of the mutant (). In the mutant Δsll5043, 15 μg ml–1 WT-RPS had no effect on cell aggregation, whereas the inhibitory effect of 26 μg ml–1 WT-RPS on cell aggregation was lower than that of 40 μg ml–1 WT-RPS (Supplementary fig. 10a). Low concentration of WT-RPS (15 and 26 μg ml–1) had no effect on biofilm formation of the mutant Δsll5043 (Supplementary fig. 10b). This indicated that the inhibitory effect of WT-RPS on cell aggregation and biofilm formation was concentration-dependent. Additionally, 40 μg ml–1 WT-RPS stimulated the dispersion of cell aggregates and biofilms of the three mutants (Supplementary fig. 11), further demonstrating the inhibitory effect of WT-RPS on cell aggregation and biofilm formation.
Fig. 4. Effect of WT-RPS on (a) cell aggregation and (b) biofilm formation of the mutants of Synechocystis 6803. Φ, without addition of RPS; M-RPS, with addition of RPS from the mutant itself (40 μg ml–1); WT-RPS, with addition of 40 μg ml–1 WT-RPS. Different lowercase letters at the top of bars indicate significant differences between the data of the same strain
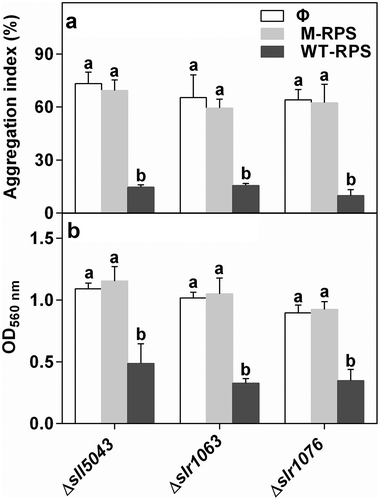
40 μg ml–1 WT-RPS significantly decreased cell hydrophobicity of three mutants (Δsll5043, Δslr1063 and Δslr1076) by 92.0 ± 3.2%, 88.3 ± 1.1% and 90.4 ± 6.5%, respectively, while the RPS from each mutant itself had no effect on cell hydrophobicity of the mutant (). For the WT and the mutant Δsll5043, cell hydrophobicity decreased with the increasing concentration of WT-RPS in BG11 medium (Supplementary fig. 12).
Fig. 5. Effect of WT-RPS on cell hydrophobicity of the mutants of Synechocystis 6803. Φ, without addition of RPS; M-RPS, with addition of RPS from the mutant itself (40 μg ml–1); WT-RPS, with addition of 40 μg ml–1 WT-RPS. Different lowercase letters at the top of bars indicate significant differences between the data of the same strain
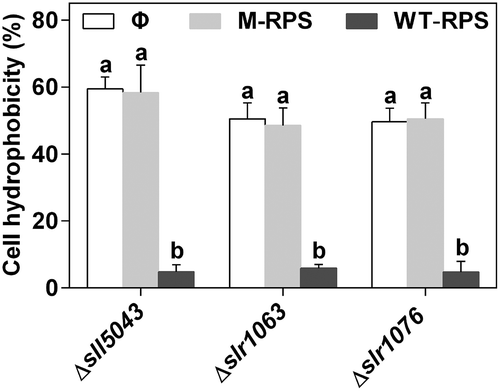
Discussion
Changes of EPS content and physicochemical properties in the mutants
In this study, compared with the WT, the contents of CPS and RPS in the three mutants decreased by 72.6–79.6% and 31.8–88.7%, respectively. Similarly, compared with the WT of Synechocystis 6803, the CPS content has been previously reported to decrease by <50% in the mutant lacking the putative EPS synthesis genes sll1722-24 encoding glycosyltransferases (Foster et al., Citation2009). The EPS content has also been reported in other mutants of Synechocystis 6803 defective in the putative EPS synthesis genes encoding EPS exporting proteins Wza (sll1581), Wzb (slr0328) and Wzc (sll0923 and sll5052), and ExoD enzyme (slr1875) (Jittawuttipoka et al., Citation2013; Pereira et al., Citation2019). Compared with the WT, the RPS content is lower in the mutants Δsll0923 (2-fold decrease) and Δslr0328 (35% decrease), and the CPS content is lower in the mutants Δsll0923 (2-fold decrease), Δslr1875 (2-fold decrease) and Δsll1581 (4-fold reduction). However, the RPS content is unaffected in the mutant Δsll1581 and slightly increased in the mutants Δslr1875 and Δsll5052, and the CPS content is unaffected in the mutants Δsll5052 and Δslr0328.
It has been previously reported that compared with the WT, the monosaccharide composition of CPS is altered in the mutants of Synechocystis 6803 with deletion of putative EPS synthesis genes slr1610 (predicted to encode a methyltransferase), slr0982 (predicted to encode an ATP binding component of an ABC transporter) and slr0977 (predicted to encode a permease component of an ABC transporter) (Fisher et al., Citation2013). Similarly, the monosaccharide composition of RPS and CPS in the three mutants was found to be different from that in the WT in this study. Physical properties of microbial EPSs are related to their chemical structure (Sutherland, Citation1994). The contents of uronic acid and hydrophobic deoxy sugars (rhamnose and fucose) affect the solubility and hydrophobic feature of EPS (Sutherland, Citation2001; Giroldo et al., Citation2003). The high proportion of deoxy sugars in terminal monosaccharides increases the stickiness of EPS (Vieira et al., Citation2008), while the presence of uronic acid in the side-chains may increase the solubility of EPS (Sutherland, Citation1994). The changes of emulsification activity and viscosity of RPS in the three mutants could result from the change in chemical characterization of RPS.
Cell aggregation and biofilm formation in the mutants
In this study, the three EPS-deficient mutants of Synechocystis 6803 (Δsll5043, Δslr1063 and Δslr1076) showed high levels of cell aggregation and biofilm formation. This phenomenon is consistent with some previously reported results in Synechocystis 6803. The EPS-deficient mutant strains (Δslr0977, Δslr0982 and Δslr1610) with altered monosaccharide composition of CPS form biofilm during static growth (Fisher et al., Citation2013). The mutant Δsll1581, exhibiting the lower content of CPS and the unaffected content of RPS (Jittawuttipoka et al., Citation2013), shows a higher level of cell aggregation in 0.8 × BG11 medium compared with the WT (Allen et al., Citation2019). However, inconsistent with the result in this study, the mutant Δsll0923 of Synechocystis 6803, exhibiting the lower content of RPS and CPS (Jittawuttipoka et al., Citation2013), shows a WT level of cell aggregation in 0.8 × BG11 medium (Allen et al., Citation2019).
In this study, lower negative cell surface charge (negative zeta potential) and cell surface hydrophobicity were found in the three mutants when compared with the WT. Lower negative zeta potentials have been previously observed in the mutants Δsll0923 and Δsll1581 of Synechocystis 6803, while the mutants Δslr1875 and Δsll5052 display zeta potentials similar to WT cells (Jittawuttipoka et al., Citation2013). The change in cell surface charge and hydrophobicity could be attributed to the altered content and/or chemical characterization of CPS in the mutants. In this study, WT-RPS exhibiting high emulsification activity was found to decrease cell surface hydrophobicity, while RPSs from the mutants exhibiting no emulsification activity had no effect on cell hydrophobicity. Similarly, biosurfactants have been previously reported to influence cell hydrophobicity in bacteria. Emulsan, the exocellular polymeric emulsifier produced by Acinetobacter calcoaceticus, reduces cell hydrophobicity of A. calcoaceticus and Streptococcus pyogenes (Rosenberg et al., Citation1983). Rhamnolipid decreases cell hydrophobicity of Bacillus subtilis BUM and Rhodococcus erythropolis 3586 (Zhao et al., Citation2011; Feng et al., Citation2013). Moreover, antibiofilm EPS from Streptococcus phocae PI80 with high emulsification activity inhibits intercellular adhesion E. coli cells (Kanmani et al., Citation2011). The influence of WT-RPS on cell hydrophobicity could be related to its emulsification activity. Cell surface hydrophobicity and cell surface charge are two important factors in microbial adhesion and biofilm formation (Mukherjee et al., Citation2012). Since cell surfaces carry a net negative charge, electrostatic interactions in microbial adhesion are repulsive, while hydrophobic interactions are attractive. Hydrophobic and electrostatic interactions jointly affect microbial adhesion. For the three mutants in this study, the less negative cell surface charge reduced the electrostatic repulsion between the cells and was favourable to the formation of aggregates and biofilms. The decreased cell surface hydrophobicity in the mutants reduced the adherence between the cells, and thus had a detrimental effect on the formation of aggregates and biofilms. RPSs from the three mutants were unable to decrease cell hydrophobicity and had no effect on the formation of aggregates and biofilms. By contrast, for the WT, the greater negative cell surface charge and the presence of WT-RPS able to decrease cell hydrophobicity were detrimental to cell aggregation and biofilm formation, while the higher cell surface hydrophobicity was favourable to cell aggregation and biofilm formation. Therefore, the differences in cell aggregation and biofilm formation between the WT and the three mutants could be related to differences in cell hydrophobicity, cell surface charge and physicochemical properties of RPS.
The inhibitory effect of WT-RPS on cell aggregation and biofilm formation in the mutants
40 μg ml–1 WT-RPS significantly inhibited cell aggregation and biofilm formation of the three mutants, while the RPS from each mutant itself had no effect on cell aggregation and biofilm formation of the mutant (). In the mutant Δsll5043, 15 μg ml–1 WT-RPS had no effect on cell aggregation, whereas the inhibitory effect of 26 μg ml–1 WT-RPS on cell aggregation was lower than that of 40 μg ml–1 WT-RPS (Supplementary fig. 10a). Low concentration of WT-RPS (15 and 26 μg ml–1) had no effect on biofilm formation of the mutant Δsll5043 (Supplementary fig. 10b). This indicated that the inhibitory effect of WT-RPS on cell aggregation and biofilm formation was concentration-dependent. Additionally, 40 μg ml–1 WT-RPS stimulated the dispersion of cell aggregates and biofilms of the three mutants (Supplementary fig. 11), further demonstrating the inhibitory effect of WT-RPS on cell aggregation and biofilm formation.
High concentration (40 μg ml–1) WT-RPS significantly decreased cell hydrophobicity of the three mutants, and significantly inhibited cell aggregation and biofilm formation, while the RPS from each mutant alone had no effect. Furthermore, in the mutant Δsll5043, cell hydrophobicity decreased with the increasing concentration of WT-RPS in BG11 medium, and the inhibitory effect of WT-RPS on cell aggregation and biofilm formation was concentration-dependent. The inhibitory effect of WT-RPS on cell aggregation of the mutant Δsll5043 was increased with the increasing concentration of WT-RPS from 15 μg ml–1 to 40 μg ml–1. Low concentration of WT-RPS (15 and 26 μg ml–1) had no effect on biofilm formation. The above results demonstrated that the inhibitory effect of WT-RPS which exhibited high emulsification activity on cell aggregation and biofilm formation directly related to reduction of hydrophobic interaction between the cells. Exopolysaccharides possessing emulsification activity have been previously found in a desert cyanobacterium Anabaena variabilis (Bhatnagar et al., Citation2012), a paddy field cyanobacterium Cyanothece epiphytica (Borah et al., Citation2018), a terrestrial cyanobacterium Nostoc flagelliforme (Shen et al., Citation2019) and a marine cyanobacterium Cyanothece sp. CCY 0110 (Mota et al., Citation2020). The ecological role of EPS in cell aggregation and biofilm formation should be further investigated in these cyanobacteria. In a natural open water body, the cyanobacterial RPS might become diffuse, and thus have a weak influence on cell aggregation and biofilm formation. However, in a closed habitat, e.g. a photobioreactor, the cyanobacterial RPS might accumulate to a relatively high concentration and have a stronger influence on cell aggregation and biofilm formation.
In conclusion, our data in this study indicated that not only CPS but also RPS were able to affect cell aggregation and biofilm formation in Synechocystis 6803, and WT-RPS inhibited cell aggregation and biofilm formation. To our knowledge, this is the first report on the effect of RPS on cell aggregation and biofilm formation in cyanobacteria. Synechocystis 6803 is a potential model organism for studies of phototrophic cell aggregation and biofilm formation (Allen et al., Citation2019). The data obtained in this study will be useful in understanding the mechanism of cell aggregation and biofilm formation in cyanobacteria.
Author contributions
J. Li: original concept, experiments, data analysis and drafting manuscript; D.Y. Fang: experiments and data analysis; R.M. Ye: experiments and data analysis; C.F. Zhou: original concept and data analysis; P.F. Li: original concept, data analysis and editing manuscript.
Supplemental Material
Download TIFF Image (2.7 MB)Supplemental Material
Download TIFF Image (2.4 MB)Supplemental Material
Download TIFF Image (37.6 KB)Supplemental Material
Download TIFF Image (748 KB)TEJP-2020-0001-File015.tif
Download TIFF Image (483.8 KB)TEJP-2020-0001-File014.tif
Download TIFF Image (490.5 KB)TEJP-2020-0001-File013.tif
Download TIFF Image (444 KB)TEJP-2020-0001-File011.tif
Download TIFF Image (13.5 MB)TEJP-2020-0001-File007.pdf
Download PDF (91 KB)Disclosure statement
No potential conflict of interest was reported by the authors.
Supplementary Information
The following supplementary material is accessible via the Supplementary Content tab on the article’s online page at http://doi.org/10.1080/09670262.2019.1777469
Supplementary table 1. The oligonucleotide primers used for amplifying the DNA fragments containing the targeted genes and the flanking regions.
Supplementary table 2. Plasmids used in this study.
Supplementary table 3. The oligonucleotide primers used to confirm complete chromosome segregation in the mutants.
Supplementary table 4. One-way ANOVA test results of .
Supplementary table 5. One-way ANOVA test results of .
Supplementary table 6. One-way ANOVA test results of .
Supplementary table 7. One-way ANOVA test results of Supplementary fig. 10.
Supplementary table 8. One-way ANOVA test results of Supplementary fig. 11.
Supplementary table 9. One-way ANOVA test results of Supplementary fig. 12.
Supplementary fig. 1. Chromosome segregation checked by agarose electrophoresis of PCR products in the mutants.
Supplementary fig. 2. Growth curves of the WT and three mutants of Synechocystis 6803.
Supplementary fig. 3. Comparison of the apparent viscosity between RPS from the WT and RPSs from the mutants of Synechocystis 6803. Δsll5043-RPS, Δslr1063-RPS and Δslr1076-RPS were RPS from Δsll5043, Δslr1063 and Δsl1076, respectively.
Supplementary figs 4–8. Cell aggregation of the WT and three mutants of Synechocystis 6803. . Cell aggregation of the WT and three mutants observed in test tubes. . Microscopic image of the unicellular WT. Figs 6–8. Microscopic images of cell aggregation of the mutants Δsll5043, Δslr1063 and Δslr1076, respectively. Scale bars: , 20 μm; Figs 6–8, 100 μm.
Supplementary fig. 9. Biofilm formation of the WT and three mutants of Synechocystis 6803 in test tubes after 7 days of growth in BG11 medium.
Supplementary fig. 10. Effect of various concentrations of WT-RPS on cell aggregation (a) and biofilm formation (b) of the mutant Δsll5043. Different lowercase letters at the top of bars indicate significant differences between the data.
Supplementary fig. 11. Effect of WT-RPS on dispersion of cell aggregates and biofilms formed by the mutants of Synechocystis 6803. Φ, without addition of WT-RPS; WT-RPS, with addition of 40 μg ml–1 WT-RPS. The asterisks at the top of the bars indicate significant difference compared with control (without addition of WT-RPS).
Supplementary fig. 12. Effect of various concentrations of WT-RPS on cell hydrophobicity of the WT (a) and the mutant Δsll5043 (b). Different lowercase letters at the top of bars indicate significant differences between the data (p < 0.05).
Additional information
Funding
References
- Allen, R., Rittmann, B.E. & Curtiss, R. (2019). Axenic biofilm formation and aggregation by Synechocystis sp. strain PCC 6803 are induced by changes in nutrient concentration and require cell surface structures. Applied and Environmental Microbiology, 85: e02192–18.
- Barros, A.I., Goncalves, A.L., Simoes, M. & Pires, J.C.M. (2015). Harvesting techniques applied to microalgae: a review. Renewable and Sustainable Energy Reviews, 41: 1489–1500.
- Bhatnagar, M., Pareek, S., Ganguly, J. & Bhatnagar, A. (2012). Rheology and composition of a multi-utility exopolymer from a desert borne cyanobacterium Anabaena variabilis. Journal of Applied Phycology, 24: 1387–1394.
- Blumenkr, N. & Asboehan, G. (1973). New method for quantitative-determination of uronic acids. Analytical Biochemistry, 54: 484–489.
- Borah, D., Nainamalai, S., Gopalakrishnan, S., Rout, J., Alharbi, N.S., Alharbi, S.A. & Nooruddin, T. (2018). Biolubricant potential of exopolysaccharides from the cyanobacterium Cyanothece epiphytica. Applied Microbiology and Biotechnology, 102: 3635–3647.
- Cooper, D.G. & Goldenberg, B.G. (1987). Surface-active agents from 2 Bacillus species. Applied and Environmental Microbiology, 53: 224–229.
- Dubois, M., Gilles, K.A., Hamilton, J.K., Rebers, P.A. & Smith, F. (1956). Colorimetric method for determination of sugars and related substances. Analytical Chemistry, 28: 350–356.
- Enomoto, G., Ni-Ni-Win., Narikawa, R. & Ikeuchi, M. (2015). Three cyanobacteriochromes work together to form a light color-sensitive input system for c-di-GMP signaling of cell aggregation. Proceedings of the National Academy of Sciences USA, 112: 8082–8087.
- Fattom, A. & Shilo, M. (1984). Hydrophobicity as an adhesion mechanism of benthic cyanobacteria. Applied and Environmental Microbiology, 47: 135–143.
- Feng, W., Swift, S. & Singhal, N. (2013). Effects of surfactants on cell surface tension parameters and hydrophobicity of Pseudomonas putida 852 and Rhodococcus erythropolis 3586. Colloids and Surfaces B-Biointerfaces, 105: 43–50.
- Fisher, M.L., Allen, R., Luo, Y. & Curtiss, R. (2013). Export of extracellular polysaccharides modulates adherence of the cyanobacterium Synechocystis. PLoS ONE, 8: e74514.
- Foster, J.S., Havemann, S.A., Singh, A.K. & Sherman, L.A. (2009). Role of mrgA in peroxide and light stress in the cyanobacterium Synechocystis sp. PCC 6803. FEMS Microbiology Letters, 293: 298–304.
- Giroldo, D., Vieira, A.A.H. & Paulsen, B.S. (2003). Relative increase of deoxy sugars during microbial degradation of an extracellular polysaccharide released by a tropical freshwater Thalassiosira sp. (Bacillariophyceae). Journal of Phycology, 39: 1109–1115.
- Hu, T., Jiang, C., Huang, Q. & Sun, F. (2016). A comb-like branched β-D-glucan produced by a Cordyceps sinensis fungus and its protective effect against cyclophosphamide-induced immunosuppression in mice. Carbohydrate Polymers, 142: 259–267.
- Jittawuttipoka, T., Planchon, M., Spalla, O., Benzerara, K., Guyot, F., Cassier-Chauvat, C. & Chauvat, F. (2013). Multidisciplinary evidences that Synechocystis PCC6803 exopolysaccharides operate in cell sedimentation and protection against salt and metal stresses. PLoS ONE, 8: e55564.
- Kanmani, P., Kumar, R.S., Yuvaraj, N., Paari, K.A., Pattukumar, V. & Arul, V. (2011). Production and purification of a novel exopolysaccharide from lactic acid bacterium Streptococcus phocae PI80 and its functional characteristics activity in vitro. Bioresource Technology, 102: 4827–4833.
- Katarzyna, L., Sai, G. & Singh, O.A. (2015). Non-enclosure methods for non-suspended microalgae cultivation: literature review and research needs. Renewable & Sustainable Energy Reviews, 42: 1418–1427.
- Kawano, Y., Saotome, T., Ochiai, Y., Katayama, M., Narikawa, R. & Ikeuchi, M. (2011). Cellulose accumulation and a cellulose synthase gene are responsible for cell aggregation in the cyanobacterium Thermosynechococcus vulcanus RKN. Plant and Cell Physiology, 52: 957–966.
- Kera, K., Nagayama, T., Nanatani, K., Saeki-Yamoto, C., Tominaga, A., Souma, S., Miura, N., Takeda, K., Kayamori, S., Ando, E., Higashi, K., Igarashi, K. & Uozumi, N. (2018). Reduction of spermidine content resulting from inactivation of two arginine decarboxylases increases biofilm formation in Synechocystis sp. strain PCC 6803. Journal of Bacteriology, 200: e00664–17.
- Li, P., Cai, Y., Shi, L., Geng, L., Xing, P., Yu, Y., Kong, F. & Wang, Y. (2009). Microbial degradation and preliminary chemical characterization of Microcystis exopolysaccharides from a cyanobacterial water bloom of Lake Taihu. International Review of Hydrobiology, 94: 645–655.
- Maeda, K., Tamura, J., Okuda, Y., Narikawa, R., Midorikawa, T. & Ikeuchi, M. (2018). Genetic identification of factors for extracellular cellulose accumulation in the thermophilic cyanobacterium Thermosynechococcus vulcanus: proposal of a novel tripartite secretion system. Molecular Microbiology, 109: 121–134.
- Mantzorou, A. & Ververidis, F. (2019). Microalgal biofilms: a further step over current microalgal cultivation techniques. Science of the Total Environment, 651: 3187–3201.
- McDougald, D., Rice, S.A., Barraud, N., Steinberg, P.D. & Kjelleberg, S. (2011). Should we stay or should we go: mechanisms and ecological consequences for biofilm dispersal. Nature Reviews Microbiology, 10: 39–50.
- Mota, R., Vidal, R., Pandeirada, C., Flores, C., Adessi, A., De Philippis, R., Nunes, C., Coimbra, M.A. & Tamagnini, P. (2020). Cyanoflan: a cyanobacterial sulfated carbohydrate polymer with emulsifying properties. Carbohydrate Polymers, 229: 115525.
- Mukherjee, J., Karunakaran, E. & Biggs, C. A. (2012). Using a multi-faceted approach to determine the changes in bacterial cell surface properties influenced by a biofilm lifestyle. Biofouling, 28: 1–14.
- Nakao, M., Okamoto, S., Kohara, M., Fujishiro, T., Fujisawa, T., Sato, S., Tabata, S., Kaneko, T. & Nakamura, Y. (2010). CyanoBase: the cyanobacteria genome database update 2010. Nucleic Acids Research, 38: D379–D381.
- Pereira, S., Zille, A., Micheletti, E., Moradas-Ferreira, P., De Philippis, R. & Tamagnini, P. (2009). Complexity of cyanobacterial exopolysaccharides: composition, structures, inducing factors and putative genes involved in their biosynthesis and assembly. FEMS Microbiology Reviews, 33: 917–941.
- Pereira, S.B., Mota, R., Vieira, C.P., Vieira, J. & Tamagnini, P. (2015). Phylum-wide analysis of genes/proteins related to the last steps of assembly and export of extracellular polymeric substances (EPS) in cyanobacteria. Scientific Reports, 5: 14835.
- Pereira, S.B., Santos, M., Leite, J.P., Flores, C., Eisfeld, C., Büttel, Z., Mota, R., Rossi, F., De Philippis, R., Gales, L., Morais-Cabral, J.H. & Tamagnini, P. (2019). The role of the tyrosine kinase Wzc (Sll0923) and the phosphatase Wzb (Slr0328) in the production of extracellular polymeric substances (EPS) by Synechocystis PCC 6803. MicrobiologyOpen, 8: e00753.
- Reasoner, D.J. & Geldreich, E.E. (1985). A new medium for the enumeration and subculture of bacteria from potable water. Applied and Environmental Microbiology, 49: 1–7.
- Rippka, R., Deruelles, J., Waterbury, J.B., Herdman, M. & Stanier, R.Y. (1979). Generic assignments, strain histories and properties of pure cultures of cyanobacteria. Journal of General Microbiology, 111: 1–61.
- Roeselers, G., van Loosdrecht, M.C.M. & Muyzer, G. (2008). Phototrophic biofilms and their potential applications. Journal of Applied Phycology, 20: 227–235.
- Rosenberg, E., Gottlieb, A. & Rosenberg, M. (1983). Inhibition of bacterial adherence to hydrocarbons and epithelial cells by emulsan. Infection and Immunity, 39: 1024–1028.
- Schatz, D., Nagar, E., Sendersky, E., Parnasa, R., Zilberman, S., Carmeli, S., Mastai, Y., Shimoni, E., Klein, E., Yeger, O., Reich, Z. & Schwarz, R. (2013). Self-suppression of biofilm formation in the cyanobacterium Synechococcus elongatus. Environmental Microbiology, 15: 1786–1794.
- Shen, S.G., Lin, Y.H., Zhao, D.X., Wu, Y.K., Yan, R.R., Zhao, H.B., Tan, Z.L., Jia, S.R. & Han, P.P. (2019). Comparisons of functional properties of polysaccharides from Nostoc flagelliforme under three culture conditions. Polymers, 11: 263.
- Sutherland, I.W. (1994). Structure-function relationships in microbial exopolysaccharides. Biotechnology Advances, 12: 393–448.
- Sutherland, I.W. (2001). Exopolysaccharides in biofilms, flocs and related structures. Water Science and Technology, 43: 77–86.
- Tillett, D. & Neilan, B. A. (2000). Xanthogenate nucleic acid isolation from cultured and environmental cyanobacteria. Journal of Phycology, 36: 251–258.
- Vieira, A.A.H., Ortolano, P.I.C., Giroldo, D., Oliveira, M.J.D., Bittar, T.B., Lombardi, A.T. & Sartori, A.L. (2008). Role of hydrophobic extracellular polysaccharide of Aulacoseira granulata (Bacillariophyceae) on aggregate formation in a turbulent and hypereutrophic reservoir. Limnology and Oceanography, 53: 1887–1899.
- Xiao, M., Li, M. & Reynolds, C.S. (2018). Colony formation in the cyanobacterium Microcystis. Biological Reviews, 93: 1399–1420.
- Zang, X., Liu, B., Liu, S., Arunakumara, K.K.I.U. & Zhang, X. (2007). Optimum conditions for transformation of Synechocystis sp. PCC 6803. Journal of Microbiology, 45: 241–245.
- Zhao, Z., Selvam, A. & Wong, J. W. C. (2011). Effects of rhamnolipids on cell surface hydrophobicity of PAH degrading bacteria and the biodegradation of phenanthrene. Bioresource Technology, 102: 3999–4007.