ABSTRACT
The harmful effects of ultraviolet (UV) radiation have increased the demand for new sources of UV photoprotectants to develop cosmetic and skin care products. In this study, a filamentous cyanobacterial strain was isolated from the topsoil collected in an arid and exposed region in Chetimari, Niger. On the basis of 16S rDNA sequences, the isolated strain was identified as Pseudanabaena sp. CCNU1. UV-B treatment of Pseudanabaena sp. CCNU1 significantly enhanced the production of active photoprotectants, including UV-absorbing mycosporine-like amino acids (MAAs) and carotenoids. UV-B irradiation mainly induced the production of one MAA, identified as palythine-serine. The palythine-serine content was as high as 16 mg g−1 dry weight after a 4-day UV-B treatment. Eight carotenoids were detected in the methanol extracts of UV-B-treated Pseudanabaena sp. CCNU1: β-carotene, echinenone, canthaxanthin, zeaxanthin, synechoxanthin, and three myxoxanthophyll derivatives. With the exception of β-carotene and echinenone, these carotenoids were positively correlated with the UV-B treatment duration. Thus, Pseudanabaena sp. CCNU1 was identified as a unique organism that produces a considerable abundance of palythine-serine as well as carotenoids to protect against UV-B radiation. Pseudanabaena sp. CCNU1 may be useful as a producer of UV photoprotectants, although the genetic basis of the relevant biosynthetic pathways remains to be precisely characterized in the future.
Introduction
Mycosporine-like amino acids (MAAs) are low molecular weight (188–756 Da), water-soluble, colourless UV-absorbing compounds (Shick & Dunlap, Citation2002; Carreto & Carignan, Citation2011; Shang et al., Citation2018). The conjugation of cyclohexenone or cyclohexeniminone rings to various amino acids leads to the formation of different aminocyclohexenone-type MAAs or aminocyclohexene imine-type MAAs (Shick & Dunlap, Citation2002; Chrapusta et al., Citation2017). The diversity in the substituents on the central rings explains the differences in UV absorbance, with a maximum wavelength between 312 and 360 nm and a high molar absorptivity of 2.81 × 104 to 5.00 × 104 M−1 cm−1 (Shick & Dunlap, Citation2002). More than 40 structurally diverse MAAs or MAA derivatives have been detected in bacteria, cyanobacteria, algae, fungi, corals and dinoflagellates, with at least some of them considered to be promising active ingredients useful for the cosmetics industry (Wada et al., Citation2015; Chrapusta et al., Citation2017; Derikvand et al., Citation2017; Rosic, Citation2019). A formulation containing the MAA shinorine as an active ingredient has been developed and included in commercially available sunscreen products (Cardozo et al., Citation2007).
Cyanobacteria are the oldest photoautotrophic prokaryotes that can produce chemical energy from light energy via plant-like oxygenic photosynthesis. The sunlight required for photosynthesis inevitably exposes cyanobacteria to UV radiation. Starting approximately 2.6–3.5 billion years ago, cyanobacteria evolved sophisticated ways to synthesize MAAs to mitigate the adverse effects of UV irradiation, enabling their ubiquitous distribution on Earth in the absence of an ozone layer (Jain et al., Citation2017). The abundance and composition of MAAs vary among cyanobacterial species and are influenced by environmental factors, including light fluctuation and nutrient availability (Carreto & Carignan, Citation2011; Chrapusta et al., Citation2017). In this study, we analysed the production of MAAs and other photoprotectants in a new cyanobacterial strain isolated from the terrestrial topsoil in Chetimari, Niger.
Materials and methods
Sample collection and cyanobacterial isolation
Topsoil was collected from Chetimari (13°12′23″N, 12°25′16″E) in south-eastern Niger (), which receives intense solar radiation and is characterized by extreme drought conditions for most of the year. The collected topsoil was sealed in plastic bags and transported to our laboratory in China. The soil was gently submerged in liquid BG11 medium to promote cyanobacterial growth at 25°C under 30 μmol photons m−2 s−1 photosynthetically active radiation (PAR; 400–700 nm). The upper green solution was then spread evenly on BG11 medium solidified by 1% agar to produce individual cyanobacterial colonies. Finally, a cyanobacterial strain was isolated and cultured in liquid BG11 medium. The cyanobacterial strain was morphologically characterized with a confocal laser scanning microscope (TCS SP5; Leica).
Fig. 1 Collection site and morphology of the new cyanobacterial isolate. (a) Terrestrial topsoil samples were collected in Chetimari, Niger. (b) Microscopic analyses revealed the filamentous morphology of the isolated cyanobacterial strain. (c) The cyanobacteria cultured in liquid medium formed aggregates. The scale bar in panel b represents 10 μm
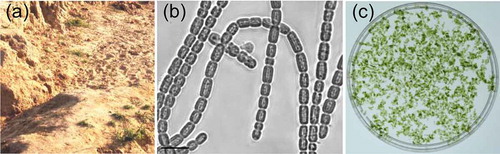
16S rDNA amplification and phylogenetic analysis
Genomic DNA was extracted from the cultured cyanobacterial isolate as described by Zhang et al. (Citation2019). The genomic DNA was used as the template for the amplification of 16S rDNA by PCR with primers of 16S_27F (5′-AGAGTTTGATCCTGGCTCAG-3′) and 16S_1494R (5′-TACGGCTACCTTGTTACGAC-3′). The PCR product was sequenced and submitted to GenBank (accession number MN892538). Additionally, the sequence was subjected to a BLAST analysis and subsequently used to construct a phylogenetic tree with the MEGA software (version 6.0) to classify the cyanobacterial isolate (Zhang et al., Citation2019).
MAA production and extraction
An actively growing culture of the cyanobacterial isolate was divided into several uncovered Petri dishes and exposed to 0.15 W m−2 UV-B radiation and 30 μmol photons m−2 s−1 PAR. The UV-B radiation was emitted from fluorescent UV-B lamps (TL 40W/12 RS; Philips, Germany). Ultra-white glass was used to filter the radiation below 280 nm (Chen et al., Citation2020) (Supplementary fig. S1). The biologically effective UV-B radiation was calculated based on the biological spectral weighting function described by Flint & Caldwell (Citation2003). The UV-B-treated cultures were collected at specific time-points and centrifuged at 4000 × g for 5 min, after which the pelleted cyanobacteria were resuspended in methanol and incubated at 4°C overnight to extract MAAs. Samples were centrifuged at 4000 × g for 10 min, and the collected supernatants underwent a spectroscopic analysis (280–800 nm) with a UV/Vis spectrophotometer (UV-2700; Shimadzu) to confirm MAA production.
Detection and characterization of MAAs
The methanol extracts of new cyanobacterial isolate were dried in a vacuum concentrator (Labconco, UK) and then resuspended in Milli-Q water (18.2 MΩ cm−1). An equal volume of chloroform was added to eliminate the pigments in the solutions before the MAAs were characterized by liquid chromatography-mass spectrometry (LC-MS) with the 6540 UHD Accurate-Mass Q-TOF system (Agilent). The solutions were injected into a reversed-phase high pressure liquid chromatography (HPLC) system with in-line absorption spectroscopy and an Inertsil ODS-SP column (5 μm, 4.6 mm × 250 mm; GL Sciences Inc., Japan). The MAAs were detected at 320 nm after the following gradient elution involving mobile phase A (methanol) and mobile phase B (water) at a flow rate of 1 ml min−1: 0–7 min, 1–20% mobile phase A; 7–9 min, 20–50% mobile phase A; 9–17 min, 50–80% mobile phase A; 17–22 min, 80% mobile phase A. The eluted samples were analysed by electrospray ionization mass spectrometry (positive ion mode). Additionally, the MAA content was calculated based on an extinction coefficient of 10 500 M−1 cm−1 (Wada et al., Citation2015).
Characterization and quantification of carotenoids
The new cyanobacterial isolate cultured under 30 μmol photons m−2 s−1 PAR was centrifuged at 4000 × g for 5 min. The pigments in the pelleted cells were extracted in pre-cooled methanol and then immediately injected into an HPLC system (Shimadzu LC-20AT) equipped with a DAD detector (SPD-M20A). The Inertsil ODS-SP column (5 μm, 4.6 mm × 250 mm; GL Sciences Inc.) was used to separate pigments, which were detected at 470 nm. The following gradient elution with mobile phase A (methanol) and mobile phase B (water) was completed with a flow rate of 0.5 ml min−1: 0–20 min, 90% mobile phase A; 20–90 min, 90–100% mobile phase A; 90–91 min, 100% mobile phase A; 91–100 min, 100–90% mobile phase A. The carotenoids were identified by comparing the retention times and absorption spectra with those of known pigments extracted from Synechocystis sp. PCC 6803 and Nostoc flagelliforme (Takaichi et al., Citation2001; Yang et al., Citation2019). The carotenoid content changes were determined based on their HPLC peak areas and a normalization against the chlorophyll (Chl) a content.
Statistical analysis
Data were analysed by Tukey’s test with the SPSS software (version 11.5). The significance threshold was set at 0.05.
Results
Classification of a new cyanobacterial isolate
The isolated cyanobacterial strain had filaments that were straight, wavy or arcuate, and lacked branches (). The cells in the filaments were cylindrical and blunt at both ends, 2.3–7.6 μm long and 2.7–4.4 μm wide (), with constrictions at the cross walls between adjacent cells. The cyanobacterial cells formed aggregates when cultured in liquid medium (). Based on the 16S rDNA phylogenetic tree, the isolated strain was classified as the genus Pseudanabaena (Supplementary fig. S2) and named Pseudanabaena sp. CCNU1 (i.e. abbreviation of Central China Normal University). Consistent with this classification, the Pseudanabaena sp. CCNU1 16S rDNA was more similar to the corresponding sequences in Pseudanabaena sp. SCSIO and Pseudanabaenaceae CENA319 (98% and 97% identity, respectively) than to other related strains (< 95%) (Supplementary table S1).
Effects of UV-B radiation on MAA production
The cellular Chl a content was responsible for an absorbance peak at 665 nm and contributed to the absorbance at 400–600 nm along with the carotenoids. The absorbance at 300–400 nm reflected the substantial MAA production induced by the UV-B treatment (). The relatively small peak at 320 nm suggested MAA was constitutively produced at low levels in Pseudanabaena sp. CCNU1 (). The MAA production increased slightly after a 6 h exposure to 0.15 W m−2 UV-B radiation but increased significantly more after a 24 h UV-B treatment (). The changes to the MAA contents were positively related with the duration of the UV-B treatment, suggesting the MAAs may protect Pseudanabaena sp. CCNU1 from UV-B radiation.
Fig. 2 Characterization of MAAs in Pseudanabaena sp. CCNU1. The MAA production in Pseudanabaena sp. CCNU1 was induced by an exposure to 0.15 W m−2 UV-B radiation for up to 24 h. (a) The absorbance spectra of the methanol extracts were normalized against the absorption at 665 nm. (b) The MAAs in the methanol extracts of Pseudanabaena sp. CCNU1 treated with 0.15 W m−2 UV-B radiation for 7 days were analysed by liquid chromatography–mass spectrometry (c) to determine their molecular weight (d) and obtain in-line absorption spectra. The proposed MAA structure is presented in panel c
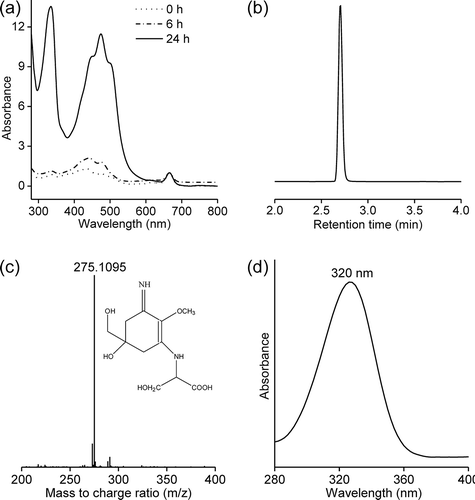
An HPLC analysis at 320 nm revealed an obvious peak at 2.7 min, suggesting the UV-B irradiation predominantly induced the production of one MAA in Pseudanabaena sp. CCNU1 (). The mass spectrum of the compound corresponding to this peak included a prominent ion peak for a protonated fragment [M+H]+ at m/z 275.1077 (). Additionally, this compound had an in-line absorbance spectrum centred at 320 nm (). The mass and absorbance spectra were consistent with the characteristics of the previously identified MAA palythine-serine (Singh et al., Citation2010). Accordingly, palythine-serine may be the dominant or the only MAA produced in Pseudanabaena sp. CCNU1. In response to continuous exposure to UV-B radiation, the palythine-serine content increased, peaking at 1.6% of the Pseudanabaena sp. CCNU1 dry weight after a 4-day treatment (). There were no significant changes to palythine-serine production in Pseudanabaena sp. CCNU1 at the subsequent time-points ().
Fig. 3 Analysis of the Pseudanabaena sp. CCNU1 MAA contents. The Pseudanabaena sp. CCNU1 culture was exposed to 30 μmol photons m−2 s−1 photosynthetically active radiation and 0.15 W m−2 UV-B radiation for up to 8 days. Different letters on the error bars indicate significant differences (Tukey’s HSD, p ≤ 0.05). Data are presented as the mean ± standard deviation (n = 3)
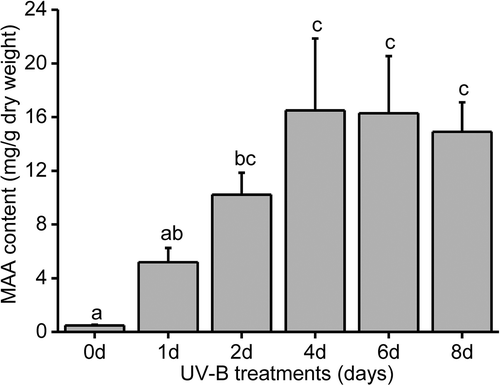
Effects of UV-B radiation on carotenoid production
The increased absorbance at 400–600 nm (after normalizing against the absorbance of Chl a) indicated that the UV-B treatment significantly enhanced carotenoid production (). The HPLC analysis revealed nine major pigments in the methanol extracts for Pseudanabaena sp. CCNU1 (). The pigment corresponding to peak 7 was identified as Chl a based on its typical absorption spectrum and the similarity between its retention time and that of Chl a from Synechocystis sp. PCC 6803 (, b, 5g) (Chen, Citation2014). The pigments for peaks 1, 4, 5, 8, and 9 had retention times similar to those of synechoxanthin, myxoxanthophyll, zeaxanthin, echinenone, and β-carotene from Synechocystis sp. PCC 6803, respectively (), with typical absorption spectra for these carotenoids (). The retention times of the pigments for peaks 2 and 6 were similar to those of a myxoxanthophyll compound and canthaxanthin from N. flagelliforme, respectively (). The absorption spectra of the pigments for peaks 2 and 6 were consistent with the characteristics of myxoxanthophyll and canthaxanthin, respectively (). Unexpectedly, the pigment corresponding to peak 3 was not detected in Synechocystis sp. PCC 6803 or N. flagelliforme. However, the absorption spectrum for this pigment was identical to that of the pigments for peaks 2 and 4. Accordingly, peaks 2, 3, and 4 probably represented myxoxanthophyll derivatives ().
Fig. 4 (a) HPLC profiles of the methanol extracts for Pseudanabaena sp. CCNU1, (b) Synechocystis sp. PCC 6803 and (c) Nostoc flagelliforme analysed at a detection wavelength of 470 nm. The Synechocystis sp. PCC 6803 and N. flagelliforme data as well as the in-line absorption spectra (see ) were used to identify the major pigments in Pseudanabaena sp. CCNU1 as follows: peak 1, synechoxanthin; peak 2, myxoxanthophyll derivative 1; peak 3, myxoxanthophyll derivative 2; peak 4, myxoxanthophyll derivative 3; peak 5, zeaxanthin; peak 6, canthaxanthin; peak 7, chlorophyll a; peak 8, echinenone; peak 9, β-carotene
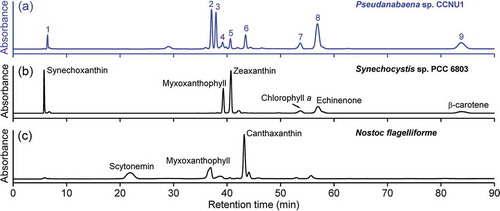
Fig. 5 In-line absorption spectra of the pigments separated by HPLC. The peak number in each spectrum corresponds to the number in . (a) synechoxanthin; (b) myxoxanthophyll derivative 1; (c) myxoxanthophyll derivative 2; (d) myxoxanthophyll derivative 3; (e) zeaxanthin; (f) canthaxanthin; (g) chlorophyll a; (h) echinenone; (i) β-carotene
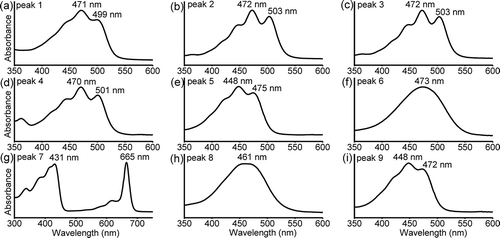
In Pseudanabaena sp. CCNU1, the echinenone content remained relatively stable throughout a 4-day UV-B treatment, whereas a sharp decrease in the β-carotene content was observed 4 days after initiating the UV-B irradiation (). In contrast, the contents of the other carotenoids increased as the UV-B treatment time increased, suggesting they may be necessary for Pseudanabaena sp. CCNU1 responses to UV-B radiation (). The decrease in the β-carotene content in the UV-B-treated Pseudanabaena sp. CCNU1 may have been due to an increase in the conversion of β-carotene to other carotenoids ().
Fig. 6 Carotenoid contents in Pseudanabaena sp. CCNU1. The cyanobacterial culture was treated with 0.15 W m−2 UV-B radiation for 1 and 4 days. PAR, photosynthetically active radiation. Different letters on the error bars indicate significant differences (Tukey’s HSD, p ≤ 0.05). Data are presented as the mean ± standard deviation (n = 4 or 5)
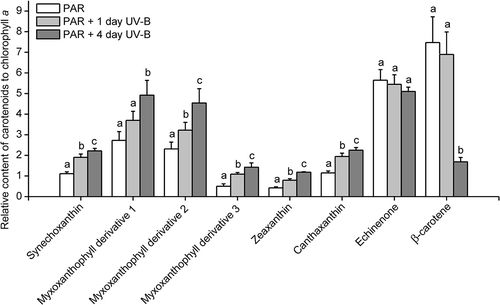
Discussion
In this study, we isolated a new filamentous cyanobacterial strain (Pseudanabaena sp. CCNU1) and characterized its production of the MAA palythine-serine. Pseudanabaena sp. CCNU1 taxonomically belongs to the order Oscillatoriales. A recent review of MAA-producing cyanobacteria included a few Oscillatoriales species, including Arthrospira sp. CU2556, Lyngbya sp. CU2555, Microcoleus chthonoplastes, Oscillatoria spongelidae, and Trichodesmium spp. (Jain et al., Citation2017). In a previous study, palythine-serine was identified as one of multiple MAAs in several coral species (Carignan et al., Citation2009). An earlier investigation of the effects of sulphur-deficient conditions on Anabaena variabilis PCC 7937 revealed an increase in the palythine-serine content, but an even greater increase in the shinorine content (Singh et al., Citation2010). Another study proved that palythine-serine represents a small proportion of the multiple MAA analogues produced by Scytonema cf. crispum (D’Agostino et al., Citation2016). In the current study, considerable amounts of palythine-serine were produced in Pseudanabaena sp. CCNU1 only in response to UV-B radiation (). Thus, Pseudanabaena sp. CCNU1 differs from other cyanobacteria in terms of its MAA production. To the best of our knowledge, this is the first study to reveal the substantial production of a single MAA (palythine-serine) in an organism exposed to UV stress.
More than 40 kinds of MAAs have been detected in diverse organisms (Wada et al., Citation2015; Chrapusta et al., Citation2017), but the investigations on the genes and biochemical pathways involved in their production have mainly focused on the biosynthesis of mycosporine-glycine and shinorine in cyanobacteria (Balskus & Walsh, Citation2010; Gao & Garcia-Pichel, Citation2011a, Citation2011b; Pope et al., Citation2015). Briefly, 4-deoxygadusol can be synthesized by the shikimate and pentose pathways as the central ring of MAAs, after which a condensation reaction with glycine produces mycosporine-glycine, to which serine is subsequently ligated to yield shinorine (Balskus & Walsh, Citation2010; Gao & Garcia-Pichel, Citation2011b; Pope et al., Citation2015). On the basis of structural relationships, palythine-serine is probably synthesized via the successive decarboxylation and demethylation of the glycine unit of shinorine (Singh et al., Citation2010; Carreto & Carignan, Citation2011). However, we did not detect the potential intermediates of mycosporine-glycine and shinorine. Accordingly, a shinorine-independent pathway may exist for the biosynthesis of palythine-serine in Pseudanabaena sp. CCNU1. Palythine-serine production may represent a unique MAA biosynthetic pathway in cyanobacteria. Thus, Pseudanabaena sp. CCNU1 may be an ideal palythine-serine producer, and the associated biosynthetic pathway may have biotechnological applications.
Carotenoids are a common group of pigments belonging to the terpenoid family. In addition to their light-harvesting roles in the photosynthetic apparatus, carotenoids are also well-known photoprotectants that scavenge reactive oxygen species or dissipate excess energy as heat (Domonkos et al., Citation2013). Several studies confirmed that carotenoid contents increase in cyanobacteria exposed to UV-B radiation (Ehling-Schulz et al., Citation1997; Ivanov et al., Citation2000; Mandal et al., Citation2011; Joshi et al., Citation2018). A genetic analysis identified zeaxanthin as an effective antioxidant that can protect cyanobacteria from the deleterious effects of UV-B radiation (Götz et al., Citation1999). In the current study, the zeaxanthin, canthaxanthin and myxoxanthophyll contents concomitantly increased in response to a UV-B treatment, suggesting they help protect Pseudanabaena sp. CCNU1 from UV-B radiation (). Our findings are consistent with the results of earlier investigations in which the canthaxanthin and myxoxanthophyll contents of Nostoc commune and Chlorogloeopsis fritschii PCC 6912 significantly increased following a UV-B treatment (Ehling-Schulz et al., Citation1997; Llewellyn et al., Citation2020). Unfortunately, there are relatively few other reports describing the types of UV-B radiation-induced carotenoids in cyanobacteria. Increased synechoxanthin production in Pseudanabaena sp. CCNU1 due to a UV-B treatment () has not previously reported. Synechoxanthin appears to be produced in Pseudanabaena sp. CCNU1, but not in other Pseudanabaena strains, as well as in other cyanobacterial species, including Synechococcus sp. PCC 7002 and Synechocystis sp. PCC 6803 (Graham & Bryant, Citation2008; Graham et al., Citation2008). Furthermore, alloxanthin, lycopene and α-carotene were undetectable in Pseudanabaena sp. CCNU1, but are produced in two other Pseudanabaena strains (Takaichi & Mochimaru, Citation2007; Takaichi, Citation2013; Paliwal et al., Citation2015; Wojtasiewicz & Stoń-Egiert, Citation2016; Yang et al., Citation2019). These differences in carotenoid profiles suggest Pseudanabaena sp. CCNU1 has unique carotenogenic pathways, and the protective effects of the carotenoids against UV-B radiation may differ among cyanobacterial strains and species.
Pseudanabaena sp. CCNU1 is the first isolated organism revealed to produce a large amount of a single MAA (palythine-serine) to mitigate the adverse effects of UV radiation. The genes and biosynthetic pathways regulating palythine-serine and carotenoid production in Pseudanabaena sp. CCNU1 will be elucidated in future studies.
Supplementary table S1. Comparison of the 16S rDNA of the newly isolated Pseudanabaena sp. CCNU1 and other cyanobacteria. The 16S rDNA accession numbers are provided in parentheses.
Supplementary fig. S1. Energy emission spectrum of the UV-B lamp (a), transmission spectrum of the ultra-white glass filter (b) and weighted UV radiation (c) based on the biological spectral weighting function described by Flint & Caldwell (Citation2003) for UV-B-treated samples.
Supplementary fig. S2. Neighbour-joining phylogenetic tree of cyanobacteria based on the 16S rDNA sequences of Pseudanabaena sp. CCNU1 (from this study) and other species obtained from a BLAST search of the NCBI and GenBank databases. Bootstrap values greater than 50% are provided at the nodes. The main clades are indicated. The Pseudanabaena sp. CCNU1 isolated in this study is boxed.
Author contributions
B.S. Qiu conceived the study. M.C.M. Boukar: isolated the cyanobacterial strain and wrote the manuscript; L.Q. Shen; helped classify the cyanobacterial isolate; K. Wang: detected and characterize the MAAs and carotenoids; Z.C. Zhang: detected and characterize the MAAs and carotenoids, analysed the data and wrote the manuscript; B.S. Qiu; conceived the study and revised the manuscript.
TEJP-2020-0075-File008.pdf
Download PDF (286.8 KB)Disclosure statement
No potential conflict of interest was reported by the author(s).
Supplementary information
The following supplementary material is accessible via the Supplementary Content tab on the article’s online page at https://doi.org/10.1080/09670262.2020.1824019
Additional information
Funding
References
- Balskus, E.P. & Walsh, C.T. (2010). The genetic and molecular basis for sunscreen biosynthesis in cyanobacteria. Science, 329: 1653–1656.
- Cardozo, K.H.M., Guaratini, T., Barros, M.P., Falcao, V.R., Tonon, A.P., Lopes, N.P., Campos, S., Torres, M.A., Souza, A.O., Colepicolo, P. & Pinto, E. (2007). Metabolites from algae with economical impact. Comparative Biochemistry and Physiology Part C Toxicology and Pharmacology, 146: 60–78.
- Carignan, M.O., Cardozo, K.H.M., Oliveira-Silva, D., Colepicolo, P. & Carreto, J.I. (2009). Palythine-threonine, a major novel mycosporine-like amino acid (MAA) isolated from the hermatypic coral Pocillopora capitata. Journal of Photochemistry and Photobiology B, 94: 191–200.
- Carreto, J.I. & Carignan, M.O. (2011). Mycosporine-like amino acids: relevant secondary metabolites. Chemical and ecological aspects. Marine Drugs, 9: 387–446.
- Chen, M. (2014). Chlorophyll modifications and their spectral extension in oxygenic photosynthesis. Annual Review of Biochemistry, 83: 317–340.
- Chen, Z., Jiang, H.B., Gao, K. & Qiu, B.S. (2020). Acclimation to low ultraviolet-B radiation increases photosystem I abundance and cyclic electron transfer with enhanced photosynthesis and growth in the cyanobacterium Nostoc sphaeroides. Environmental Microbiology, 22: 183–197.
- Chrapusta, E., Kaminski, A., Duchnik, K., Bober, B., Adamski, M. & Bialczyk, J. (2017). Mycosporine-like amino acids: potential health and beauty ingredients. Marine Drugs, 15: 326.
- D’Agostino, P.M., Javalkote, V.S., Mazmouz, R., Pickford, R., Puranik, P.R. & Neilan, B.A. (2016). Comparative profiling and discovery of novel glycoslated mycosporine-like amino acids in two strains of cyanobacterium Scytonema cf. crispum. Applied and Environmental Microbiology, 82: 5951–5959.
- Derikvand, P., Llewellyn, C.A. & Purton, S. (2017). Cyanobacterial metabolites as a source of sunscreens and moisturizers: a comparison with current synthetic compounds. European Journal of Phycology, 52: 43–56.
- Domonkos, I., Kis, M., Gombos, Z. & Ughy, B. (2013). Carotenoids, versatile components of oxygenic photosynthesis. Progress in Lipid Research, 52: 539–561.
- Ehling-Schulz, M., Bilger, W. & Scherer, S. (1997). UV-B-induced synthesis of photoprotective pigments and excellular polysaccharides in the terrestrial cyanobacterium Nostoc commune. Journal of Bacteriology, 179: 1940–1945.
- Flint, S. & Caldwell, M. (2003). A biological spectral weighting function for ozone depletion research with higher plants. Physiologia Plantarum, 117: 137–144.
- Gao, Q. & Garcia-Pichel, F. (2011a). Microbial ultraviolet sunscreens. Nature Review Microbiology, 9: 791–802.
- Gao, Q. & Garcia-Pichel, F. (2011b). An ATP-grasp ligase involved in the last biosynthetic step of the iminomycosporine shinorine in Nostoc punctiforme ATCC 29133. Journal of Bacteriology, 193: 5923–5928.
- Götz, T., Windhovel, U., Böger, P. & Sandmann, G. (1999). Protection of photosynthesis against UV-B radiation by carotenoids in transformants of the cyanobacterium Synechococcus PCC 7942. Plant Physiology, 120: 599–604.
- Graham, J.E. & Bryant, D.A. (2008). The biosynthetic pathway for synechoxanthin, an aromatic carotenoid synthesized by the euryhaline, unicellular cyanobacterium Synechococcus sp. strain PCC 7002. Journal of Bacteriology, 190: 7966–7974.
- Graham, J.E., Lecomte, J.T.J. & Bryant, D.A. (2008). Synechoxanthin, an aromatic C40 xanthophyll that is a major carotenoid in the cyanobacterium Synechococcus sp. PCC 7002. Journal of Natural Products, 71: 1647–1650.
- Ivanov, A.G., Miskiewicz, E., Clarke, A.K. & Greenberg, B.M. (2000). Protection of photosystem II against UV-A and UV-B radiation in the cyanobacterium Plectonema boryanum: the role of growth temperature and grow irradiance. Photochemistry and Photobiology, 72: 772–779.
- Jain, S., Prajapat, G., Abrar, M., Ledwani, L., Singh, A. & Agrawal, A. (2017). Cyanobacteria as efficient producers of mycosporine-like amino acids. Journal of Basic Microbiology, 57: 715–727.
- Joshi, D., Mohandass, C. & Dhale, M. (2018). Effects of UV-B radiation and desiccation stress on photoprotective compounds accumulation in marine Leptolyngbya sp. Applied Biochemistry and Biotechnology, 184: 35–47.
- Llewellyn, C.A., Airs, R.L., Farnham, G. & Greig, C. (2020). Synthesis, regulation and degradation of carotenoids under low level UV-B radiation in the filamentous cyanobacterium Chlorogloeopsis fritschii PCC 6912. Frontiers in Microbiology, 11: 163.
- Mandal, S., Rath, J. & Adhikary, S.P. (2011). Adaptation strategies of the sheathed cyanobacterium Lyngbya majuscule to ultraviolet-B. Journal of Photochemistry and Photobiology B, 102: 115–122.
- Paliwal, C., Ghosh, T., Bhayani, K., Maurya, R. & Mishra, S. (2015). Antioxidant, anti-nephrolithe activities and in vitro digestibility studies of three different cyanobacterial pigment extracts. Marine Drugs, 13: 5384–5401.
- Pope, M.A., Spence, E., Seralvo, V., Gacesa, R., Heidelberger, S., Weston, A.J., Dunlap, W.C., Shick, J.M. & Long, P.F. (2015). O-methyltransferase is shared between the pentose phosphate and shikimate pathway and is essential for mycosporine-like amino acid biosynthesis in Anabaena variabilis ATCC 29413. ChemBioChem, 16: 320–327.
- Rosic, N.N. (2019). Mycosporine-like amino acid: making the foundation for organic personalized sunscreens. Marine Drugs, 17: 638.
- Shang, J.L., Zhang, Z.C., Yin, X.Y., Chen, M., Hao, F.H., Wang, K., Feng, J.L., Xu, H.F., Yin, Y.C., Tang, H.R. & Qiu, B.S. (2018). UV-B induced biosynthesis of a novel sunscreen compound in solar radiation and desiccation tolerant cyanobacteria. Environmental Microbiology, 20: 200–213.
- Shick, J.M. & Dunlap, W.C. (2002). Mycosporine-like amino acids and related gadusols: biosynthesis, accumulation, and UV-protective functions in aquatic organisms. Annual Review of Physiology, 64: 223–262.
- Singh, S.P., Klisch, M., Sinha, R.P. & Häder, D.P. (2010). Sulfur deficiency changes mycosporine-like amino acid (MAA) composition of Anabaena variabilis PCC 7937: a possible role of sulfur in MAA bioconversion. Photochemistry and Photobiology, 86: 862–870.
- Takaichi, S. (2013). Tetraterpenes: carotenoids. In Natural Products (Ramawat KG, Mérillon JM, editors). doi: 10.1007/978-3-642-22144-6_141. Springer-Verlag, Berlin.
- Takaichi, S. & Mochimaru, M. (2007). Carotenoids and carotenogenesis in cyanobacteria: unique ketocarotenoids and carotenoid glycosides. Cellular and Molecular Life Sciences, 64: 2607–2619.
- Takaichi, S., Maoka, T. & Masamoto, K. (2001). Myxoxanthophyll in Synechocystis sp. PCC 6803 is myxol 2́ -dimethyl-fucoside, (3R, 2́ S)-myxol 2́ -(2,4-di-O-methyl-α-L- fucoside), not rhamnoside. Plant and Cell Physiology, 42: 756–762.
- Wada, N., Sakamoto, T. & Matsugo, S. (2015). Mycosporine-like amino acids and their derivatives as natural antioxidants. Antioxidants, 4: 603–646.
- Wojtasiewicz, B. & Stoń-Egiert, J. (2016). Bio-optical characterization of selected cyanobacteria strains present in marine and freshwater ecosystems. Journal of Applied Phycology, 28: 2299–2314.
- Yang, Y.W., Yin, Y.C., Li, Z.K., Huang, D., Shang, J.L., Chen, M. & Qiu, B.S. (2019). Orange and red carotenoid proteins are involved in the adaptation of the terrestrial cyanobacterium Nostoc flagelliforme to desiccation. Photosynthesis Research, 140: 103–113.
- Zhang, Z.C., Li, Z.K., Yin, Y.C., Li, Y., Jia, Y., Chen, M. & Qiu, B.S. (2019). Widespread occurrence and unexpected diversity of red-shifted chlorophyll producing cyanobacteria in humid subtropical forest ecosystems. Environmental Microbiology, 21: 1497–1510.