Abstract
The Amt/Mep/Rh family of integral membrane proteins comprises ammonium transporters of bacteria, archaea and eukarya, as well as the Rhesus proteins found in animals. They play a central role in the uptake of reduced nitrogen for biosynthetic purposes, in energy metabolism, or in renal excretion. Recent structural information on two prokaryotic Amt proteins has significantly contributed to our understanding of this class, but basic questions concerning the transport mechanism and the nature of the transported substrate, NH3 or , remain to be answered. Here we review functional and structural studies on Amt proteins and discuss the bioenergetic issues raised by the various mechanistic proposals present in the literature.
Introduction
Nitrogen is an essential part of all biological macromolecules, but more than 99% of this element on earth are found as atmospheric dinitrogen, N2Citation[1]. In spite of its abundance, most organisms are unable to metabolize this molecule, due to the extraordinary kinetic stability of the N–N triple bond (ΔG0' = − 946 kJ×mol−1) Citation[2]. Only a distinct class of microorganisms, the diazotrophs, is able to reductively fix dinitrogen into ammonia (NH3), in a unique reaction catalyzed by the enzyme nitrogenase Citation[2], Citation[3]. A second important source of ammonia is the reductive assimilation of nitrate (), achieved by the successive action of a nitrate and a nitrite reductase in many bacteria and plants. The reason for the particular emphasis on ammonia among all the basic chemical modifications of nitrogen is that only in this reduced state can the element be assimilated into biological molecules Citation[4–6]. This occurs predominantly via either the glutamine synthetase/glutamate synthase pathway (GS/GOGAT) or through glutamate dehydrogenase (GDH), both eventually yielding glutamine, from which other amino acids can be synthesized through transamination reactions. Its exposed role in nitrogen metabolism thus makes ammonia an important nutrient whose availability is often the limiting factor for the production of biomass, requiring for example the extensive use of fertilizers in modern, industrial agriculture Citation[3].
In natural environments, uptake and storage of ammonia are essential for survival, and not surprisingly, organisms have developed various, intricate ways to assure their nitrogen supply. While animals largely rely on the uptake of nitrogen-containing amino acids through their diet, some plants harness the nitrogen-fixing ability of diazotrophic bacteria by providing them with highly specialized organs, the root nodules. Within their protected, anoxic environment with carbon and metabolic energy being provided by the host plant, the symbiotic bacteria use their highly oxygen-sensitive nitrogenase system to produce ammonia that can then be taken up by the plant for its own biosyntheses.
In its free form, ammonia is a hydrophobic gas that can permeate biological membranes with a permeability coefficient in the order of 10−3 cm·s−1, a value close to that of H2O Citation[7–9]. It has initially been suggested that – similar to other metabolically important gases, such as O2, N2 or H2 – a specific transmembrane transport system may not be required. However, NH3 is in protonation equilibrium with the charged ammonium cation () at a pKa of 9.25, such that at a typical physiological pH value of ∼7.5, about 99% will be in the membrane-impermeable, cationic form. During the 1970s and early 1980s, several lines of evidence gave rise to the assumption that ammonia/ammonium uptake into cells should be an active process occurring through dedicated membrane proteins Citation[7], Citation[10], Citation[11]. An increasing number of publications reported the existence of
concentration gradients across cell membranes in various microorganisms Citation[12–15] and evidence for ammonium uptake systems was presented. These would be required at low external concentrations of ammonium, where passive transport would not suffice to satisfy metabolic requirements Citation[14], Citation[16], Citation[17].
In 1994, the first sequences for ammonium transport proteins were presented for MEP1 from Saccharomyces cerevisiaeCitation[18] and Amt-1;1 from Arabidopsis thalianaCitation[19], followed by the first complete prokaryotic Amt sequence from Corynebacterium glutamicumCitation[20]. Today, a large amount of available sequence data outlines a distinct protein family with a high degree of conservation, comprising the ammonium transport proteins (Amt) of bacteria, archaea and plants as well as the methylammonium permeases (Mep) from yeast, and the Rhesus proteins (Rh) of animals.
The Amt/Mep/Rh Family
The nomenclature of proteins within the ammonium transport protein family may seem confusing, as many systems and most published functional assays focus on the transport properties of methylammonium (MA, ), an alternative but presumably non-physiological substrate. Because the detection and precise quantification of ammonia is often difficult, most groups turned to monitoring the accumulation of radioactively labelled 14C-MA, following protocols established by Segel and co-workers Citation[10]. Uptake of MA showed strong, competitive inhibition by
, and it has thus been inferred that the latter is the natural substrate of both Amt and Mep proteins Citation[10–13]. Sequences of both groups are highly similar, and in 1997 the Rhesus blood group proteins were identified as a further line of homologues Citation[21], Citation[22]. In the following we will use the term Amt proteins to refer to the entire family of Amt/Mep/Rh proteins. All genes encode for polypeptides of approximately 400 − 500 amino acids and hydropathy analyses consistently predict 11 − 12 transmembrane helices. They all function as uptake systems for ammonium into the cytoplasm or into a storage vacuole within the plant cell. Of particular interest is the discovery of amt genes in bacteria growing by anaerobic oxidation of ammonia (anammox), such as Kuenenia stuttgartiensisCitation[23] and by nitrification of ammonia to nitrite such as Nitrosomonas europaeaCitation[24]. In both cases, reduced nitrogen is not used for biosyntheses but for energy metabolism.
The Rhesus blood group antigens of mammalian erythrocytes had long been recognized for their immunogenic characteristics and involvement in haemolytic diseases of infants when they were identified as Amt homologues. They are components of the Rh complex, originally proposed to be a heterotetramer of two RhAG glycoproteins and the non-glycosylated RhCE or RhD subunits Citation[25]. In a more recent publication, a trimeric quaternary structure similar to Amt proteins has been proposed Citation[26], but final evidence remains to be presented. RhAG alone has been shown to rescue an ammonium-uptake-deficient phenotype in yeast, while at the same time conferring resistance to MA Citation[22], Citation[27]. The genetic inability to express Rh proteins in erythrocytes is the cause of a syndrome termed Rhnull disease Citation[28]. Its symptoms include various membrane defects and an altered cation transport across the membrane, leading to fragile red blood cells and predisposing the patients for haemolytic anaemia. Moreover, the presence of other Rhesus glycoproteins (e.g., RhBG) in mammalian kidney and liver tissues Citation[29] is consistent with the need to excrete ammonia for acid-base homeostasis Citation[30], Citation[31].
The structure of Amt proteins
It was a significant step forward for research on Amt proteins when Merrick and co-workers showed in 2002 that the AmtB protein of Escherichia coli could be overexpressed and purified from the bacterium in large amounts Citation[32]. They predicted AmtB to form stable trimers in the membrane that would remain intact during the purification procedure and eventually presented a low-resolution model obtained by cryo electron microscopy Citation[33]. In parallel, structural information on two Amt proteins was obtained by X-ray crystallography. AmtB from E. coli was solved independently by two groups Citation[34], Citation[35], followed by Amt-1 from the hyperthermophilic archaeon ArchaeoglobusfulgidusCitation[36]. At resolutions of 1.4 Å Citation[34], 1.54 Å Citation[36] and 1.8 Å Citation[35], these structural models were of exceptional quality for integral membrane proteins and provided a detailed picture of their molecular architecture.
In all three-dimensional models, the tertiary structure shows a highly conserved fold with 11 transmembrane α-helices (). The sequence of AmtB includes a twelfth, N-terminal helix that was processed and removed in the mature protein, such that both AmtB and Amt-1 have an N-out/C-in topology. As seen previously in other membrane transporters such as the ClC chloride channel Citation[37] or lactose permease Citation[38], the Amt monomers have an internal, pseudo-twofold symmetry with its axis in the plane of the membrane that relates helices I-V to helices VI-X. The additional, eleventh helix has a length of more than 48 Å and crosses both symmetry-related halves, presumably adding significantly to the stability of the protein (). The structures of AmtB and Amt-1 are highly similar in their transmembrane parts, but only in Amt-1 the entire protein was ordered in the crystal, including the conserved C-terminal region that is functionally relevant both in plant Amts and in their bacterial and archaeal homologues, where it determines the interaction with the regulatory PII proteins (see below). Recent work on A. thaliana Amt1;1 confirmed the important role of the C-terminal region and showed that it can act as an allosteric switch for transport events between monomers Citation[39].
Figure 1. Stereo representation of the monomer of Amt-1 from A. fulgidus (PDB accession code). Eleven α-helices cross the membrane. The protein is displayed in cartoon representation, with the protein chain coloured from blue at the N-terminus on the extracellular side (top) to red at the C-terminus on the cytoplasmic side (bottom).
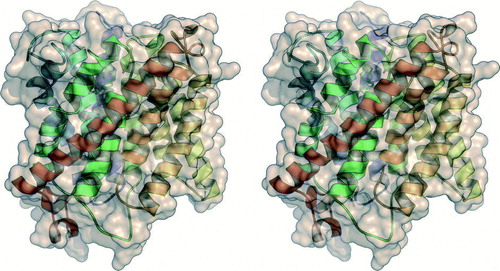
The crystal structures show a putative substrate recruitment site in each monomer on the extracellular side of the trimer (A, 2B). A cavity in the surface of Amt-1 is lined by two conserved residues, Trp 137 and Ser 208, and its bottom is formed by the side chain of Phe 96. A globular electron density peak in this cavity has been modelled as a water molecule in Amt-1, forming a short hydrogen bond of 2.65 Å to the Oγ atom of Ser 208. This position is a promising binding site for , as a positively charged ion here would be able to engage in cation-π interaction with the indole moiety of Trp 137 in addition to the hydrogen bond to Ser 208. Not only could this mode of interaction explain the observed high affinity, but the combination of H-bonding and cation-π interaction would also exclude similarly sized and abundant molecules such as H2O (not a cation) or K+ (no H-bonding interactions). Direct experimental evidence for this binding site has not yet been obtained and an assignment based solely on crystallographic data is impossible, as water and ammonia/ammonium have identical numbers of electrons. The putative binding site shows an electron density peak at identical positions in the presence and absence of
, and we have therefore modelled this peak as water in Amt-1 (A).
Figure 2. Substrate recruitment and transport in Amt-1. (A) A putative recruitment site for is located on the extracellular side of each Amt-1 monomer, where a water molecule was found in the model (red dot). The combination of hydrogen-bonding to Ser 208 and cation-π-interaction with Trp 137 provides selectivity. (B) Trimer of Amt-1 seen from the extracellular side. While each monomer is able to transport substrate, the trimeric arrangement is essential for interaction with the regulatory PII protein (). (C) A channel crosses the Amt-1 monomer, starting immediately below the putative substrate recruitment site at Typ 137 and Ser 208, extracellular side above, cytoplasmic side below. (D) Detail of (C). Substrate is blocked from passing through the channel by the side chains of Phe 96 and Phe 204, but once this obstacle is passed a channel leads directly to the cytoplasmic side of the membrane. It is lined exclusively by hydrophobic residues, with the exception of the conserved histidines His 157 and His 305.
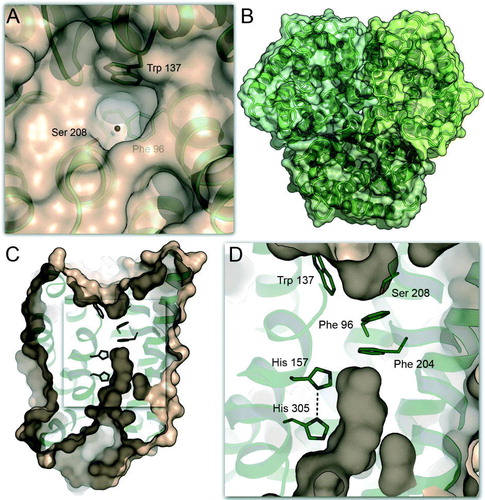
In spite of the highly conserved, trimeric quaternary structure of Amt-1, substrate transport occurs through separate pathways within each monomer, passing in between the two pseudo-symmetry-related halves. Below the extracellular binding site, a distinct channel crosses the protein (C), but is blocked by two conserved residues, Phe 96 and Phe 204, implying that substrate passage is not possible without some degree of structural rearrangement within the protein. The putative binding site is located directly above Phe 96, and immediately below the constriction an exit pathway begins, leading to the cytoplasmic side (C, 2D). Pressurization experiments with the noble gas xenon have shown this part of the channel to be hydrophobic by nature Citation[36], with the exception of two conserved histidine residues, His 157 and His 305. They are arranged with nearly coplanar imidazole rings, hydrogen-bonded through their Nδ atoms. The pseudo-twofold axis of the monomer passes right between these residues, as they are situated in corresponding positions in helices V and X, respectively. First mutational studies have underlined the significance of this motif, although the position of His 157 is found to be replaced by glutamate in some fungal Amt proteins Citation[40]. The observation of this predominantly hydrophobic channel has given rise to the assumption that NH3 rather than the more hydrophilic
cation may be the transported substrate of Amt proteins Citation[34]. Within the channel, three weak electron density peaks have been observed only in the presence of ammonium in one AmtB structure Citation[34], both with and without ammonium in the other Citation[35], and not at all in A. fulgidus Amt-1 Citation[36]. Their nature and relevance thus remains to be confirmed.
Regulation of Amt proteins
Beside their transport function, Amt proteins have been reported to participate in the regulation of nitrogen metabolism by acting as ammonium sensors Citation[41], Citation[42]. In a majority of prokaryotic organisms, the amt gene is found directly associated in an operon with a second gene encoding a protein belonging to the regulatory PII family, GlnB or its homolog GlnK (the respective products of the glnB and glnK genes Citation[43]. In order to avoid ambiguous nomenclature, the name GlnK has been proposed for all Amt-linked PII proteins and will be used in the following Citation[44]. PII proteins have also been found in higher plants, where they are encoded in the nucleus, but located in chloroplasts Citation[45]. Several crystal structures of PII proteins are available Citation[46–52]. In all cases these proteins are stable trimers, whose most prominent feature is a protruding loop in each monomer, the T-loop, which is functionally relevant and also the site of regulation by covalent modifications Citation[44]. In a most recent study, crystal structures of the PII protein GlnK1 from Methanococcus janaschii have been obtained with and without the effectors ATP and 2-oxoglutarate Citation[53]. These structures show a dramatic difference in the conformation of the T-loop in the presence and absence of Mg-ATP. While the entire loop region is extended and mildly disordered in a complex with ADP, the coordination of Mg-ATP between two monomers of the GlnK1 trimer changes the T-loop conformation such that it adopts a more compact position, closer to the protein surface. Only in this conformation is a binding site for 2-oxoglutarate observed on top of the compacted T-loop. A high ADP/ATP ratio signals a low energy level of the cell and it is assumed that under such conditions biosyntheses are down-regulated and uptake of ammonium is not desired. Yildiz et al. therefore propose that GlnK1 binds to its Amt protein in a conformational state similar to the one observed in the presence of ADP Citation[53].
First evidence for a direct association of GlnK to the Amt proteins of E. coli, Azotobacter vinelandiiCitation[54], and C. glutamicumCitation[55] was obtained in response to an ammonium pulse. The result is a direct blockage of Amt-mediated transport when nitrogen is no longer limiting or the accumulation of high levels of ammonium in the cell needs to be avoided Citation[41]. Complex formation was shown to be reversible, with dissociation occurring as the cellular nitrogen levels decrease. Based on the crystal structure of A. fulgidus Amt-1 that included the entire C-terminus and a homology model of A. fulgidus GlnK-1 we proposed a docking model for the Amt/PII complex, in which the regulatory protein binds directly to the cytoplasmic face of the transporter, its T-loops inserting into the substrate channels of Amt-1, blocking them efficiently Citation[36]. The M. jannaschii GlnK1-ADP complex supported this model, suggesting that the extended T-loops would reach even deeper into the transporter trimer Citation[53]. Direct confirmation of this docking mode came through two parallel publications of the E. coli AmtB/GlnK complex, one of which was obtained by co-crystallization of both protein components Citation[56] while in the other the complex was kept intact during purification (A) Citation[57]. A striking feature of the interaction of both proteins is the deep insertion of a conserved arginine residue, Arg 47 in E. coli GlnK, into the substrate channel (B). This residue is fixed by hydrogen bonds, resulting undoubtedly in blockage of substrate transport.
Figure 3. The complex of E. coli AmtB with GlnK. (A) Side view of the complex (PDB accession-# 2NS1) with the AmtB trimer shown in surface representation and GlnK as cartoon. In each trimer, one monomer is shown in colour. (B) Detail of the blocking of the cytoplasmic exit channel of AmtB (green) by GlnK (blue). The side chain of Arg 47 inserts into the channel, and Tyr 51, the site of uridylylation though GlnD, is in close proximity: (not shown).
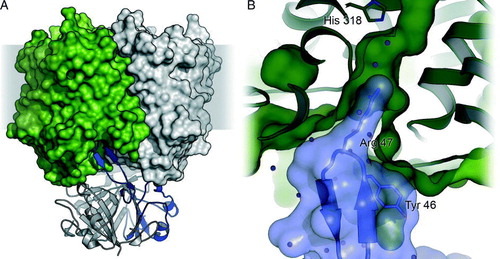
The regulation of nitrogen metabolism has been intensively studied in various species, in particular in E. coli and related enteric bacteria Citation[44], Citation[58–62]. As nitrogen is assimilated to form the amino acid glutamine, the intracellular pools of glutamine and 2-oxoglutarate are proposed to directly reflect the nitrogen supply status of the cell Citation[44], Citation[63]. Regulation of ammonium uptake occurs on the genetic level, through controlling the expression levels of the amt genes Citation[44], Citation[64] and on the protein level, by direct action of PII proteins on the function of the transporters and enzymes involved, as seen for example in the "switch-off" reaction of nitrogen fixation, a PII-controlled ADP-ribosylation of the nitrogenase iron protein that down-regulates the energetically demanding nitrogenase enzyme as soon as ammonium becomes available Citation[65], Citation[66].
Among archaea, only the nitrogen-fixing methanogens have been subject to closer investigation Citation[67]. As in other organisms, PII proteins obviously play a central regulatory role, but due to the absence of all known, covalent modification sites in the T-loop it can be assumed that other mechanisms exist to control the activity of proteins such as the ammonium transport proteins or glutamine synthetase. Genes for Amt proteins have also been found to occur in more than one copy in many organisms, such as in yeast Citation[18], tomato Citation[68], or Methanosarcina acetivoransCitation[69]. The euryarchaeon A. fulgidus possesses three independent amt genes for Amt proteins, designated amt-1, amt-2, and amt-3, each being individually followed by a gene for a PII protein. Such cases suggest a variability in the regulation of expression Citation[70] as well as in substrate affinity Citation[71], Citation[72].
Bioenergetic considerations on ammonium transport
Initial models concerning the mechanism of transmembrane ammonium transport assumed an active process leading to intracellular accumulation of . Kleiner stated that the mere finding of intracellular ammonium accumulation strongly supports active uptake systems, and that the pH gradient (acidic outside, alkaline inside) across the energized, cytoplasmic membranes of bacteria should promote depletion of ammonium rather than its accumulation if permeation was exclusively a passive process Citation[11], Citation[17] (see below). Thus the loss of ammonia due to passive diffusion across the membrane may be a significant problem for many organisms, for which they compensate by expressing Amt proteins as active (re)importers. This was referred to as cyclic retention, a process that would require a secondary active transporter, most likely as cotransport of NH3/H+Citation[7]. Such a transport system would be electrogenic, dependent on the proton-motive force and also dependent on the membrane potential. Indeed, this behaviour was found in Mep proteins of yeast Citation[18], Citation[71] and Amt proteins of A. thalianaCitation[19], Citation[73]. Voltage-clamp experiments on the tomato protein LeAMT1;1 Citation[68] and the RhBG glycoprotein Citation[74] reconstituted in Xenopus laevis oocytes further supported active uptake, albeit without being able to distinguish between a symport of NH3/H+ or uniport of
, due to the identical net charge of both processes.
An alternative mechanistic proposal originates from the work of Kustu and co-workers, who did not observe accumulation of MA in the cytoplasm of E. coliCitation[75] and Salmonella typhimuriumCitation[76]. These in vivo studies indicated the Amt protein to function as a facilitator for NH3 permeation when the external concentration of ammonium is low and led to the suggestion to use the term ammonia channel rather than ammonium transporter. As such, Amt proteins were said to constitute the first characterized class of transmembrane gas channels Citation[76]. Recently this hypothesis gained further support from the first in vitro assays done with purified AmtB reconstituted into proteoliposomes Citation[34]. Upon addition of ammonium to the outside medium, a rise of internal pH was seen, consistent with the notion that ammonia translocation over the membrane is followed by uptake of protons inside the vesicle, converting NH3 into . Together with the observations that the presence of ammonium in the crystallization buffer did not induce any change in the protein structures of either AmtB or Amt-1 and that the substrate channel is predominantly hydrophobic by nature, the view of Amt proteins as passive channels for NH3 seemed to become widely accepted Citation[77].
However, an ammonia facilitator mechanism cannot account for the observed currents in voltage-clamp experiments or the findings of intracellular ammonium accumulation. In addition it poses a very fundamental, bioenergetic problem: Since the action of Amt proteins is mainly required at low substrate concentrations, when the amounts of free NH3 are negligible, the proposed translocation would then require a deprotonation of on the extracellular side and reprotonation in the cytoplasm (A). Effectively, this would result not in passive transport of NH3, but rather in a net antiport of
and H+. In organisms using a proton gradient for energy conservation, the direction of proton transport would be against this gradient, and as a consequence the uptake of ammonia would lead to the generation of a proton motive force. More realistically, the direction of transport would reverse and the proton gradient would cause the removal of ammonia from the cytoplasm. Both possibilities clearly challenge the hypothesis of passive ammonia transport.
Figure 4. Mechanistic scheme of Amt transport. (A) At physiological pH values ammonium will be almost exclusively in the cationic form, such that translocation of NH3 implies extracellular deprotonation and intracellular reprotonation. Uniport of NH3 must therefore be considered a net antiport of versus H+. (B) Electrogenic transport as observed in some plant AMTs and Rhesus proteins is either a net uniport of
or a symport of NH3/H+.
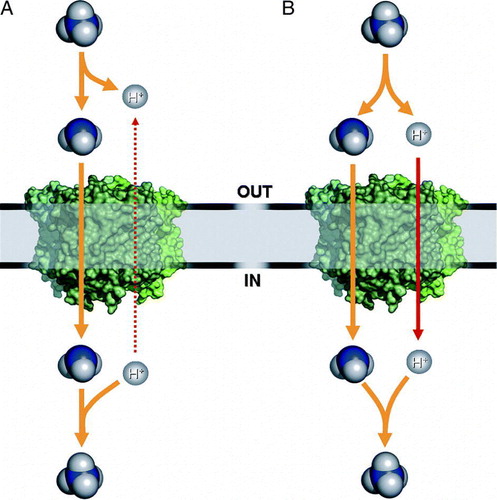
A possible solution to explain both the observed currents in voltage-clamp experiments and the seemingly passive uptake of NH3 into liposomes may be found in a mechanistic model that links the transport of NH3 to a symport of H+. In total this would result in a net uniport of , but after binding of substrate to the transporter, a deprotonation would occur with both ammonia and protons following separate – but coupled – pathways. In the presence of a proton motive force the result would be an electrogenic, secondary active transport while in its absence passive conductance of NH3 may be observed (B).
Conclusions and outlook
Recent years have seen exciting developments towards our understanding of the function of Amt proteins. High-quality structural information has become available and functional data have been collected through a multitude of techniques. Yet the results are inconclusive in respect to some of the most fundamental questions on Amt transport. Based on the crystal structures, theoretical calculations have been presented, but all were following the pre-assumption of passive transport. Ammonium has been predicted to enter the protein in its charged form and get deprotonated upon reaching the histidine pair deep inside the membrane, but the further fate of the lone proton has not yet been investigated Citation[78].
Within a diverse family of transport proteins it is not without precedent to find highly similar proteins that work by very different mechanisms. The ClC family of chloride channels/transporters for example features both active and passive transport systems with nearly identical three-dimensional structures Citation[37], Citation[50], Citation[79] and a similar situation seems possible for Amt proteins considering the fundamental differences in cellular environment found, e.g., in soil bacteria, hepatocytes, or plant root nodules. The question whether facilitated diffusion of NH3 is bioenergetically reasonable is, however, of a fundamental nature. It has been argued that all free ammonium within a cell may immediately be converted to glutamine such that a flowing equilibrium would allow for passive influx even at low external ammonium concentrations Citation[76], but any mechanism coupled to the action of glutamine synthetase would still lead to intracellular consumption and extracellular generation of protons and seems thus energetically unlikely.
Further experiments will be required to closely examine the functionality of Amt proteins in vivo and in vitro and recent structural data have provided an ideal starting point and inspiration for their design.
Acknowledgements
The authors gratefully acknowledge financial support from the European Community 6th framework program (Marie Curie) and the DFG Emmy-Noether-Programme to S.L.A.A. and from the EMBO Young Investigator Programme to O.E.
References
- Schlesinger WH. Biogeochemistry: an analysis of global change. Academic Press, San Diego 1991
- Rees DC, Howard JB. Nitrogenase: standing at the crossroads. Curr Opin Chem Biol 2000; 4: 559–566
- Rees DC, Tezcan FA, Haynes CA, Walton MY, Andrade S, Einsle O, Howard JB. Structural basis of biological nitrogen fixation. Philos Trans R Soc London, A 2005; 363: 971–984
- Crawford NM, Forde BG. Molecular and developmental biology of inorganic nitrogen nutrition. The Arabidopsis book, CR Somerville, EM Meyerowitz. American Society of Plant Biologists, Rockville, MD 2002; 1–25
- Einsle O. Cytochrome c nitrite reductase. Handbook of metalloproteins, A Messerschmidt, R Huber, K Wieghardt, T Poulos. John Wiley & Sons, NewYork 2001; 440–453
- Simon J. Enzymology and bioenergetics of respiratory nitrite ammonification. FEMS Microbiol Rev 2002; 26: 285–309
- Kleiner D. Energy expenditure for cyclic retention of during N2 fixation by Klebsiella pneumoniae. FEBS Lett 1985; 187: 237–239
- Lande MB, Donovan JM, Zeidel ML. The relationship between membrane fluidity and permeabilities to water, solutes, ammonia, and protons. J Gen Physiol 1995; 106: 67–84
- Lide DR. Handbook of chemistry and physics. Chemical Rubber Publishing, Cleveland 2001
- Hackette SL, Skye GE, Burton C, Segel IH. Characterization of an ammonium transport system in filamentous fungi with methylammonium-14C as the substrate. J Biol Chem 1970; 245: 4241–4250
- Kleiner D. The transport of NH3 and across biological membranes. Biochim Biophys Acta 1981; 639: 41–52
- Roon RJ, Even HL, Dunlop P, Larimore FL. Methylamine and ammonia transport in Saccharomyces cerevisiae. J Bacteriol 1975; 122: 502–509
- Kleiner D, Fitzke E. Some properties of a new electrogenic transport system: the ammonium (methylammonium) carrier from Clostridium pasteurianum. Biochim Biophys Acta 1981; 641: 138–147
- Stevenson R, Silver S. Methylammonium uptake by Escherichia coli: evidence for a bacterial transport system. Biochem Biophys Res Commun 1977; 75: 1133–1139
- Strenkoski LF, DeCicco BT. pH-conditional, ammonia assimilation-deficient mutants of Hydrogenomonas eutropha: evidence for the nature of the mutation. J Bacteriol 1971; 105: 296–302
- Jayakumar A, Schulman I, Macneil D, Barnes EM. Role of the Escherichia coli glnALG operon in regulation of ammonium transport. J Bacteriol 1986; 166: 281–284
- Kleiner D. Bacterial ammonium transport. FEMS Microbiol Rev 1985; 32: 87–100
- Marini AM, Vissers S, Urrestarazu A, Andre B. Cloning and expression of the MEP1 gene encoding an ammonium transporter in Saccharomyces cerevisiae. EMBO J 1994; 13: 3456–3463
- Ninnemann O, Jauniaux JC, Frommer WB. Identification of a high affinity transporter from plants. EMBO J 1994; 13: 3464–3471
- Siewe RM, Weil B, Burkovski A, Eikmanns BJ, Eikmanns M, Kramer R. Functional and genetic characterization of the (methyl)ammonium uptake carrier of Corynebacterium glutamicum. J Biol Chem 1996; 271: 5398–5403
- Marini AM, Urrestarazu A, Beauwens R, Andre B. The Rh (rhesus) blood group polypeptides are related to transporters. Trends Biochem Sci 1997; 22: 460–461
- Marini AM, Matassi G, Raynal V, Andre B, Cartron JP, Cherif-Zahar B. The human Rhesus-associated RhAG protein and a kidney homologue promote ammonium transport in yeast. Nat Genet 2000; 26: 341–344
- Strous M, Pelletier E, Mangenot S, Rattei T, Lehner A, Taylor MW, Horn M, Daims H, Bartol-Mavel D, Wincker P, Barbe V, Fonknechten N, Vallenet D, Segurens B, Schenowitz-Truong C, Medigue C, Collingro A, Snel B, Dutilh BE, Op den Camp HJM, van der Drift C, Cirpus I, van de Pas-Schoonen KT, Harhangi HR, van Niftrik L, Schmid M, Keltjens J, van de Vossenberg J, Kartal B, Meier H, Frishman D, Huynen MA, Mewes HW, Weissenbach J, Jetten MSM, Wagner M, Le Paslier D. Deciphering the evolution and metabolism of an anammox bacterium from a community genome. Nature 2006; 440: 790–794
- Schmidt I, Look C, Bock E, Jetten MSM. Ammonium and hydroxylamine uptake and accumulation in Nitrosomonas. Microbiology 2004; 150: 1405–1412
- Avent ND, Reid ME. The Rh blood group system: a review. Blood 2000; 95: 375–387
- Avent ND, Madgett TE, Lee ZE, Head DJ, Maddocks DG, Skinner LH. Molecular biology of Rh proteins and relevance to molecular medicine. Expert Rev Mol Med 2006; 8: 1–20
- Hemker MB, Cheroutre G, van Zwieten R, Maaskant-van Wijk PA, Roos D, Loos JA, van der Schoot CE, von dem Borne AE. The Rh complex exports ammonium from human red blood cells. Braz J Haematol 2003; 122: 333–340
- Huang CH. The human Rh50 glycoprotein gene. Structural organization and associated splicing defect resulting in Rhnull disease. J Biol Chem 1998; 273: 2207–2213
- Liu Z, Peng J, Mo R, Hui C, Huang CH. Rh type B glycoprotein is a new member of the Rh superfamily and a putative ammonia transporter in mammals. J Biol Chem 2001; 276: 1424–1433
- Good DW, Knepper MA. Ammonia transport in the mammalian kidney. Am J Physiol 1985; 248: F459–F471
- Knepper MA. transport in the kidney. Kidney Int Suppl 1991; 33: S95–S102
- Blakey D, Leech A, Thomas GH, Coutts G, Findlay K, Merrick M. Purification of the Escherichia coli ammonium transporter AmtB reveals a trimeric stoichiometry. Biochem J 2002; 364: 527–535
- Conroy MJ, Jamieson SJ, Blakey D, Kaufmann T, Engel A, Fotiadis D, Merrick M, Bullough PA. Electron and atomic force microscopy of the trimeric ammonium transporter AmtB. EMBO Rep 2004; 5: 1153–1158
- Khademi S, O'Connell J, Remis J, Robles-Colmenares Y, Miercke LJ, Stroud RM. Mechanism of ammonia transport by Amt/MEP/Rh: structure of AmtB at 1.35 Å. Science 2004; 305: 1587–1594
- Zheng L, Kostrewa D, Berneche S, Winkler FK, Li XD. The mechanism of ammonia transport based on the crystal structure of AmtB of Escherichia coli. Proc Natl Acad Sci USA 2004; 101: 17090–17095
- Andrade SL, Dickmanns A, Ficner R, Einsle O. Crystal structure of the archaeal ammonium transporter Amt-1 from Archaeoglobus fulgidus. Proc Natl Acad Sci USA 2005; 102: 14994–14999
- Dutzler R, Campbell EB, Cadene M, Chait BT, MacKinnon R. X-ray structure of a ClC chloride channel at 3.0 Å reveals the molecular basis of anion selectivity. Nature 2002; 415: 287–294
- Abramson J, Smirnova I, Kasho V, Verner G, Kaback HR, Iwata S. Structure and mechanism of the lactose permease of Escherichia coli. Science 2003; 301: 610–615
- Loque D, Lalonde S, Looger LL, von Wirén N, Frommer WB. A cytosolic trans-activation domain essential for ammonium uptake. Nature 2007; 446: 195–198
- Javelle A, Lupo D, Zheng L, Li XD, Winkler FK, Merrick M. An unusual twin-his arrangement in the pore of ammonia channels is essential for substrate conductance. J Biol Chem 2006; 281: 39492–39498
- Javelle A, Severi E, Thornton J, Merrick M. Ammonium sensing in Escherichia coli. Role of the ammonium transporter AmtB and AmtB-GlnK complex formation. J Biol Chem 2004; 279: 8530–8538
- Lorenz MC, Heitman J. The MEP2 ammonium permease regulates pseudohyphal differentiation in Saccharomyces cerevisiae. EMBO J 1998; 17: 1236–1247
- Thomas G, Coutts G, Merrick M. The glnKamtB operon. A conserved gene pair in prokaryotes. Trends Genet 2000; 16: 11–14
- Arcondéguy T, Jack R, Merrick M. PII signal transduction proteins, pivotal players in microbial nitrogen control. Microbiol Mol Biol Rev 2001; 65: 80–105
- Hsieh MH, Lam HM, van de Loo FJ, Coruzzi G. A PII-like protein in Arabidopsis: putative role in nitrogen sensing. Proc Natl Acad Sci USA 1998; 95: 13965–13970
- Cheah E, Carr PD, Suffolk PM, Vasudevan SG, Dixon NE, Ollis DL. Structure of the Escherichia coli Signal-Transducing Protein PII. Structure 1994; 2: 981–990
- Xu YB, Cheah E, Carr PD, van Heeswijk WC, Westerhoff HV, Vasudevan SG, Ollis DL. GInK, a PII-homologue: Structure reveals ATP binding site and indicates how the T-loops may be involved in molecular recognition. J Mol Biol 1998; 282: 149–165
- Xu Y, Carr PD, Huber T, Vasudevan SG, Ollis DL. The structure of the PII-ATP complex. Eur J Biochem 2001; 268: 2028–2037
- Benelli EM, Buck M, Polikarpov I, de Souza EM, Cruz LM, Pedrosa FO. Herbaspirillum seropedicae signal transduction protein PII is structurally similar to the enteric GlnK. Eur J Biochem 2002; 269: 3296–3303
- Schwarzenbacher R, von Delft F, Abdubek P, Ambing E, Biorac T, Brinen LS, Canaves JM, Cambell J, Chiu HJ, Dai XP, Deacon AM, DiDonato M, Elsliger MA, Eshagi S, Floyd R, Godzik A, Grittini C, Grzechnik SK, Hampton E, Jaroszewski L, Karlak C, Klock HE, Koesema E, Kovarik JS, Kuhn P, Lesley SA, Levin I, McMullan D, McPhillips TM, Miller MD, Morse A, Moy K, Ouyang J, Page R, Quijano K, Robb A, Spraggon G, Stevens RC, van den Bedem H, Velasquez J, Vincent J, Wang XH, West B, Wolf G, Xu QP, Hodgson KO, Wooley J, Wilson IA. Crystal structure of a putative PII-like signaling protein (TM0021) from Thermotoga maritima at 2.5 Å resolution. Proteins-Struct Funct Bioinformatics 2004; 54: 810–813
- Sakai H, Wang HF, Takemoto-Hori C, Kaminishi T, Yamaguchi H, Kamewari Y, Terada T, Kuramitsu S, Shirouzu M, Yokoyama S. Crystal structures of the signal transducing protein GlnK from Thermus thermophilus HB8. J Struct Biol 2005; 149: 99–110
- Nichols CE, Sainsbury S, Berrow NS, Alderton D, Saunders NJ, Stammers DK, Owens RJ. Structure of the PII signal transduction protein of Neisseria meningitidis at 1.85 Å resolution. Acta Crystallogr F 2006; 62: 494–497
- Yildiz O, Kalthoff C, Raunser S, Kühlbrandt W. Structure of GlnK1 with bound effectors indicates regulatory mechanism for ammonia uptake. EMBO J 2007; 26: 589–599
- Coutts G, Thomas G, Blakey D, Merrick M. Membrane sequestration of the signal transduction protein GlnK by the ammonium transporter AmtB. EMBO J 2002; 21: 536–545
- Strösser J, Lüdke A, Schaffer S, Krämer R, Burkovski A. Regulation of GlnK activity: modification, membrane sequestration and proteolysis as regulatory principles in the network of nitrogen control in Corynebacterium glutamicum. Mol Microbiol 2004; 54: 132–147
- Gruswitz F, O'Connell J, Stroud RM. Inhibitory complex of the transmembrane ammonia channel, AmtB, and the cytosolic regulatory protein, GlnK, at 1.96 Å. Proc Natl Acad Sci USA 2007; 104: 42–47
- Conroy MJ, Durand A, Lupo D, Li XD, Bullough PA, Winkler FK, Merrick M. The crystal structure of the Escherichia coli AmtB-GlnK complex reveals how GlnK regulates the ammonia channel. Proc Natl Acad Sci USA 2007; 104: 1213–1218
- Atkinson MR, Ninfa AJ. Role of the GlnK signal transduction protein in the regulation of nitrogen assimilation in Escherichia coli. Mol Microbiol 1998; 29: 431–447
- Blauwkamp TA, Ninfa AJ. Physiological role of the GlnK signal transduction protein of Escherichia coli: survival of nitrogen starvation. Mol Microbiol 2002; 46: 203–214
- Jack R, De Zamaroczy M, Merrick M. The signal transduction protein GlnK is required for NifL-dependent nitrogen control of nif gene expression in Klebsiella pneumoniae. J Bacteriol 1999; 181: 1156–1162
- Rhee SG, Chock PB, Stadtman ER. Glutamine synthetase from Escherichia coli. Methods Enzymol 1985; 113: 213–241
- Rhee SG, Park SC, Koo JH. The role of adenylyltransferase and uridylyltransferase in the regulation of glutamine synthetase in E. coli. Curr Top Cell Regul, S Shalteil, PB Chock. Academic Press, New York 1985; 221–232
- Kleiner D. Alkali cation transport systems. Prokaryotes, EP Bakker. VRV Press, Boca Raton, FL 1993; 379–395
- Burkovski A. I do it my way: Regulation of ammonium uptake and ammonium assimilation in Corynebacterium glutamicum. Arch Microbiol 2003; 179: 83–88
- Dodsworth JA, Cady NC, Leigh JA. 2-Oxoglutarate and the PII homologues NifI1 and NifI2 regulate nitrogenase activity in cell extracts of Methanococcus maripaludis. Mol Microbiol 2005; 56: 1527–1538
- Yakunin AF, Hallenbeck PC. AmtB is necessary for -induced nitrogenase switch-off and ADP-ribosylation in Rhodobacter capsulatus. J Bacteriol 2002; 184: 4081–4088
- Cabello P, Roldan MD, Moreno-Vivian C. Nitrate reduction and the nitrogen cycle in archaea. Microbiology 2004; 150: 3527–3546
- Ludewig U, von Wirén N, Frommer WB. Uniport of by the root hair plasma membrane ammonium transporter LeAMT1;1. J Biol Chem 2002; 277: 13548–13555
- Galagan JE, Nusbaum C, Roy A, Endrizzi MG, Macdonald P, FitzHugh W, Calvo S, Engels R, Smirnov S, Atnoor D, Brown A, Allen N, Naylor J, Stange-Thomann N, DeArellano K, Johnson R, Linton L, McEwan P, McKernan K, Talamas J, Tirrell A, Ye W, Zimmer A, Barber RD, Cann I, Graham DE, Grahame DA, Guss AM, Hedderich R, Ingram-Smith C, Kuettner HC, Krzycki JA, Leigh JA, Li W, Liu J, Mukhopadhyay B, Reeve JN, Smith K, Springer TA, Umayam LA, White O, White RH, Conway de Macario E, Ferry JG, Jarrell KF, Jing H, Macario AJ, Paulsen I, Pritchett M, Sowers KR, Swanson RV, Zinder SH, Lander E, Metcalf WW, Birren B. The genome of M. acetivorans reveals extensive metabolic and physiological diversity. Genome Res 2002; 12: 532–542
- Lauter FR, Ninnemann O, Bucher M, Riesmeier JW, Frommer WB. Preferential expression of an ammonium transporter and of two putative nitrate transporters in root hairs of tomato. Proc Natl Acad Sci USA 1996; 93: 8139–8144
- Marini AM, Soussi-Boudekou S, Vissers S, Andre B. A family of ammonium transporters in Saccharomyces cerevisiae. Mol Cell Biol 1997; 17: 4282–4293
- Montesinos ML, Muro-Pastor AM, Herrero A, Flores E. Ammonium/methylammonium permeases of a Cyanobacterium. Identification and analysis of three nitrogen-regulated amt genes in Synechocystis sp. PCC 6803. J Biol Chem 1998; 273: 31463–31470
- Gazzarrini S, Lejay L, Gojon A, Ninnemann O, Frommer WB, von Wiren N. Three functional transporters for constitutive, diurnally regulated, and starvation-induced uptake of ammonium into Arabidopsis roots. Plant Cell 1999; 11: 937–948
- Nakhoul NL, Dejong H, Abdulnour-Nakhoul SM, Boulpaep EL, Hering-Smith K, Hamm LL. Characteristics of renal Rhbg as an transporter. Am J Physiol Ren Physiol 2005; 288: F170–F181
- Soupene E, He L, Yan D, Kustu S. Ammonia acquisition in enteric bacteria: physiological role of the ammonium/methylammonium transport B (AmtB) protein. Proc Natl Acad Sci USA 1998; 95: 7030–7034
- Soupene E, Lee H, Kustu S. Ammonium/methylammonium transport (Amt) proteins facilitate diffusion of NH3 bidirectionally. Proc Natl Acad Sci USA 2002; 99: 3926–3931
- Winkler FK. Amt/MEP/Rh proteins conduct ammonia. Pflügers Archiv 2006; 451: 701–707
- Nygaard TP, Rovira C, Peters GH, Jensen MO. Ammonium recruitment and ammonia transport by E. coli ammonia channel AmtB. Biophys J 2006; 91: 4401–4412
- Lobet S, Dutzler R. Ion-binding properties of the ClC chloride selectivity filter. EMBO J 2006; 25: 24–33