Abstract
Rho GTPases are a multifunctional family of proteins that are localized at cellular membranes via an isoprenyl group covalently linked to a C-terminal cysteine. Close to this primary site of membrane anchoring there is often found an additional polybasic region (PBR), which plays a secondary role in membrane binding and targeting of the complex. Here, peptides derived from the PBRs of the Rho family proteins Rac1 (K183KRKRK), TCL (K198KKKKR) and Cdc42 (P182KKSRR) were prepared with hexalysine (K6) and hexaarginine (R6) to study their interactions with multilamellar vesicles of phosphatidylglycerol (DOPG) and headgroup-deuterated dimyristoylphosphatidylcholine (DMPC-d4) using 2H and 31P NMR. The membranes retained their lamellar architecture after peptide binding, but the 2H NMR line shapes for DMPC-d4 indicated that the bound peptides altered the orientation of the choline headgroups, consistent with a change in membrane surface charge. Rac1 and TCL peptides appeared to affect the headgroup orientation similarly to K6, although the perturbations were weaker and unlike those induced by the Cdc42 peptide and R6. Magic-angle spinning 31P NMR spectra of the membranes showed significant and selective broadening of the peak for DMPC after addition of the peptides, with R6 and the Cdc42 peptide having the greatest effect. The selective broadening may be a consequence of the lipids separating into short-lived domains enriched in peptide-bound DOPG and peptide-free DMPC. These results illustrate that a complex relationship exists between the sequence of PBRs and their behaviour at membrane surfaces, which may have implications for the cellular functions and localization of Rho GTPases.
Introduction
The Ras and Rho GTPases are proteins that participate in numerous activities in the cascade of cellular events following external primary stimulation. Rho complexes are involved in nearly every aspect of cell signalling from inflammation to regulation of the cell cycle, F-actin polymerization and cytoskeletal dynamics (Etienne-Manneville & Hall [Citation2002], Van Aelst & D'Souza-Schorey [Citation1997]), and have been implicated in the pathology of various human diseases including cancer. The majority of Rho proteins share a common mechanism of activation during signal transduction (Van Aelst & D'Souza-Schorey 1997). They are inactive in non-stimulated cells because of the presence of bound GDP molecules, but after cell stimulation GDP is exchanged for GTP inducing the activating conformational transitions in the proteins that are necessary for effector binding.
Small GTPases functionally cycle between the cytosol and cellular membranes and appear to have differential preferences for the types of membranes to which they localise. The correct subcellular localization of most Rho GTPases is dependent on the posttranslational attachment of a lipidic farnesyl or geranyl group to the cysteine residue within a CXXX motif at the C-terminus. Proteolytic removal of the last three amino acid residues occurs after prenylation and the free carboxyl group of the cysteine residue becomes methylated. Prenylation and methylation is responsible in part for the targeting and anchoring of these proteins to cell membranes, which is critically important for their biological activity (Silvius [Citation2002]). The confinement of Rho proteins to specific cell membranes increases their effective concentration at the membrane surface and increases the probability of productive collisions with effectors and other proteins.
Many small GTPases in the Ras and Rho families have a second membrane-targeting signal located just upstream of the CXXX motif. This second signal can be a polybasic region (PBR) comprised of multiple lysines or arginines, situated close to the C-terminal site of prenylation. The second signal appears to serve as a recognition element that controls the ability of the small GTPases to associate with membranes, interact with specific proteins, and localize to subcellular compartments (Williams [Citation2003], Lanning et al. [Citation2004]). Basic amino acid side groups are capable of associating with membrane surfaces through electrostatic interactions with the anionic head-groups of acidic phospholipids such as phosphatidylserine (PS), phosphatidylinositol (PI) and phosphatidylglycerol (PG). In addition, several amphipathic proteins and peptides having extended sequences of basic residues are known to segregate the acidic lipid components of membranes into laterally ordered domains (Franzin & MacDonald [Citation2001], Denisov et al. [Citation1998]). Understanding the details of membrane attachment and shuttling requires the elucidation of how, and to what extent, the polybasic regions of Rho GTPases interact with the membrane. There is considerable variation in the charge distribution across the PBRs of Rho family members according to the number and locations of the arginyl and lysyl side-groups. Roy and colleagues ([Citation2000]) showed that the cellular membrane association of K-Ras4B, which has 6 contiguous lysines in the PBR, depends mainly on the net charge within the PBR rather than on the specific order and separation of the lysines. Little is known, however, about how the ratio and positions of arginine and lysine residues affects the membrane interactions of this important region.
This work investigates how differences in the PBRs of three Rho GTPases, Rac1, Cdc42 and TCL, influence the interactions of these regions with membrane surfaces and examines what effects they have upon the phospholipids within the bilayer. Rac1 is involved in cytoskeletal regulation and the closely related proteins Cdc42 and TCL may also play a role in the regulation of intracellular trafficking, with TCL linked to the early endocytic pathway (de Toledo et al. [Citation2003], Cerione, [Citation2004]). It has been recently shown that Rac1 preferentially binds to PS-containing membranes via its polybasic motif whereas Cdc42 only associates with the same membranes when it is isoprenylated (Finkielstein et al. [Citation2006]). The PBRs of Rac1, Cdc42 and TCL differ in their lysine and arginine composition within a hexapeptide stretch close to the C-terminal site of isoprenylation (). To examine the PBRs of Rac1, Cdc42 and TCL in isolation from the remaining protein sequences hexapeptides corresponding to the PBR have been prepared and 31P and 2H nuclear magnetic resonance (NMR) spectroscopy was used to examine their interactions with multilamellar vesicles (MLVs) of acidic dioleoylphosphatidylglycerol (DOPG) mixed with zwitterionic dimyristoylphosphatidylcholine (DMPC) or dioleoylphosphatidylcholine (DOPC). PS is the major anionic lipid found in the cytosolic leaflets of eukaryotic cell membranes, but here DOPG was used as the anionic lipid species because it has favourable NMR properties, its signature peak being well resolved from that of phosphatidylcholine in the 31P spectrum. It is shown that the peptides have different effects upon the structure and organization of the lipid components, which may bear relation to the roles and localities of the parent proteins in the cell.
Materials and methods
Peptide synthesis and purification
Synthesis was carried out by standard solid phase FMOC-Wang based chemistry (Novachem, UK). Peptides were cleaved from the resin using trifluoroacetic acid/aniosole and ether precipitated, spun down and dried. The pellet was resuspended in water and purified by reverse-phase HPLC (Gilson, UK) on a C18 column (Phenonemex). The pure peptide fraction was confirmed by MALDI-TOF mass spectroscopy. A ninhydrin assay (Rosen [Citation1957]) was carried out to determine the peptide concentration against a standard calibration curve.
Preparation of vesicles
MLVs for 2H NMR experiments were prepared by dissolving the lipids DOPG (Sigma UK) and choline α- and β- deuterated DMPC (DMPC-d4) (Avanti Polar Lipids, Inc., USA) in chloroform/methanol to a 2:1 molar ratio with DMPC-d4 in excess, the total lipid content being 10 mg. The sample was dried down to a lipid film under argon and then under high vacuum overnight. The film was resuspended in 50 µl of deuterium depleted buffer (20 mM phosphate, 1mM EDTA, pH 7.4). The lipid mixture was vortexed and subjected to 5 cycles of freeze-thawing. The same procedure was used for the 31P NMR, except that undeuterated lipid was used (5 mg sample) and the buffer was 20 mM Tris buffer with 1 mM EDTA, pH 7.4. The vesicle suspensions were transferred to a 4 mm diameter zirconia sample spinner ready for NMR analysis. Peptides were added to the suspensions in the appropriate buffer to achieve a lipid to peptide molar ratio of 50:1 or 20:1, and the vesicles were again vortexed and freeze-thawed.
NMR
All NMR experiments were performed on a Bruker Avance 400 spectrometer operating at 161.98 MHz for 31P and 61.42 MHz for 2H. Measurements were performed with a solid-state magic-angle spinning (MAS) triple resonance probe for measurements under both non-spinning and MAS conditions. The temperature was maintained at 303 K for experiments on non-spinning samples and 277 K for MAS experiments. The MAS rate was maintained at 4 kHz.
Wide line 2H NMR spectra were collected using the quadrupole echo pulse scheme π/2x-τ1-π/2y-τ2-Acq (Davis et al. [Citation1976]), with t1 set to 22 µs and t2 set to 20 µs. Typically 6 K transients were accumulated to provide the presented spectra. For static 31P NMR, a single Π/2 pulse of 3.75µs was used to generate transverse magnetization, and the free induction decay was acquired with simultaneous proton decoupling at a field of 60 KHz. 12 K transients were collected to obtain the final spectra. For 31P MAS NMR, 1 K transients were accumulated to obtain the final spectra.
Results
Effects on lipid organization
It is now accepted that some basic peptides interact with biomembrane surfaces and induce the formation of domains having a distinct lipid and/or protein composition. Such domains are formed when negatively charged lipids are attracted toward the positive charges of lysine and arginine side groups of peptides residing at the membrane surface. In some cases, the domains can be sufficiently long-lived to be resolved on an NMR interaction time scale (tens of microseconds) (Franzin & MacDonald [Citation2001]). Since Cdc42 and TCL may play a role in the regulation of intracellular trafficking, it is possible that their PBRs influence the organization of specific lipids within cell membranes. We therefore applied a recently devised approach using 31P NMR to investigate whether peptides corresponding to their PBRs could induce lipid domain formation in model membranes. Hexapeptide fragments derived from the polybasic regions of the signalling proteins Rac1 (K183KRKRK), TCL (K198KKKKR) and Cdc42 (P182KKSRR) were prepared with the N-termini acetylated and the C-termini amidated so as to carry the same net formal charge as the corresponding sequences in the parent proteins. Experiments on the three PBR peptides were performed alongside identical measurements of the model peptides hexalysine (K6), which also corresponds to the PBR of K-Ras 4B, and hexaarginine (R6) for comparison.
All six peptides bound with equally high affinity to MLVs of DMPC/DOPG as determined by analysis of lipid/peptide co-sedimentation (data not presented). Magic angle spinning (MAS) NMR was used to obtain high-resolution 31P NMR spectra from membranes of DOPC and DOPG and membranes of DMPC and DOPG, in order to observe peaks from the phosphate groups of the individual lipid components. The association of peptides with the polar head-groups of individual lipid species can be detected from changes in 31P chemical shifts and line widths at half peak height (Bonev et al. [Citation2001], Pinheiro & Watts [Citation1994]). It was shown in our earlier work that by comparing the 31P NMR spectra of DOPC/DOPG and DMPC/DOPG membranes at 4°C, selective line broadening observed after addition of peptides is consistent with lipid reorganization (Madine et al. [Citation2006]). The reasons for the choice of lipids and temperature will be explained later in this section.
Spectra obtained before and after the addition of each of the peptides to a lipid/peptide molar ratio of 20:1 are shown for DOPC/DOPG in and for DMPC/DOPG in . The chemical shifts and peak widths at half height (Δν½) for the two lipids are summarized in and . The chemical shifts for the lipid components of the two membrane samples underwent slight changes upon addition of the peptides. All the peptides invoked a decrease in the chemical shift values for the two lipids in the DOPC/DOPG sample. Similar behaviour of 31P NMR spectra was observed after the addition of pentalysine to DOPC/DOPG membranes (Bonev et al. [Citation2001]) and the slight changes in shift values probably reflect the weakly electrostatic nature of peptide association with the membrane surface. Interestingly, addition of the arginine-containing peptides to the DMPC/DOPG membranes had the opposite effects, increasing the chemical shifts for both lipids. This behaviour may arise from subtle differences in the way these peptides affect membranes containing identical polar head groups but hydrocarbon chains of different length and order of saturation. The bulky guanidinium groups of arginine might, for example, penetrate beneath the membrane surface and have an effect on the order, orientation or dynamics of the lipids to an extent that is modulated by the hydrocarbon chain content of the bilayer.
Figure 2. 31P MAS NMR spectra of MLVs of DOPC/DOPG (2:1 molar ratio) at 4°C. Spectra are shown for the lipid sample alone (a) and after the addition of the 5 basic hexapeptides to a lipid/peptide molar ratio of 20:1 (b–f).
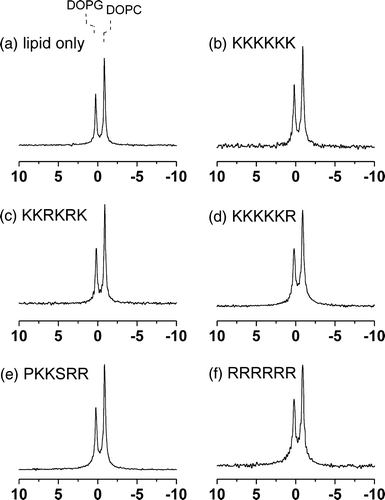
Figure 3. 31P MAS NMR spectra of MLVs of DMPC/DOPG (2:1 molar ratio) at 4°C. Spectra are shown for the lipid sample alone (a) and after the addition of the 5 basic hexapeptides to a lipid/peptide molar ratio of 20:1 (b–f).
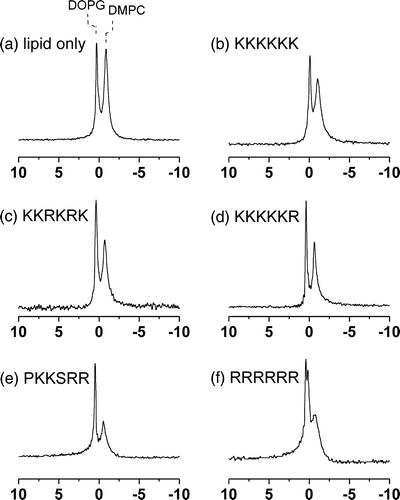
Table I. Summary of 31P NMR isotropic chemical shifts (σi) and line widths at half height (Δν½) at 4°C for MLVs of DOPC/DOPG alone and in the presence of the 5 basic hexapeptides at a lipid/peptide molar ratio of 20:1.
Table II. Summary of 31P NMR isotropic chemical shifts (σi) and line widths at half height (Δν½) at 4°C and apparent chemical shift anisotropy (Δσ) at 30°C for MLVs of DMPC/DOPG alone and in the presence of the 5 basic hexapeptides at a lipid/peptide molar ratio of 20:1.
None of the peptides had a large or selective effect on the widths of the two peaks in the spectra of the DOPC/DOPC membranes ( and ), consistent with the peptides having little or no observable constraint on the dynamics of the lipid molecules. By contrast, the peptides produced varying effects on the line widths for the DMPC/DOPG membranes ( and ). A slight narrowing of the peak for DOPG occurred after the addition of the peptides, but a little or no change in the width of the DMPC resonance occurred in the presence of K6 (b), KKRKRK (c) or KKKKKR (d). After the addition of PKKSRR (e) and R6 (f) the peak for DMPC broadened considerably (by > 40%).
As was implied earlier in this section, the line widths of the DMPC/DOPG and DOPC/DOPG membranes at 4°C provide clues about how the peptides affect the organization of the lipids within the bilayer. The spectra of the mixed membranes after the addition of R6 and PKKSRR are very similar to the spectrum obtained from a mixture of preformed DMPC MLVs and preformed DOPG vesicles at 4°C, which also exhibits a narrow peak for the fluid DOPG but a significantly broader peak for DMPC (, right). In the mixture of preformed vesicles, the saturated chains of the DMPC molecules are well below the melting temperature (∼23°C) and are packed together in a gel phase with restricted segmental motion. These lipids undergo slow or intermediate rates of rotational and lateral diffusion, which consequently broadens the 31P NMR peak. When DMPC and DOPG are mixed homogeneously within the same bilayer in the absence of peptides, both lipids remain fluid at 4°C because the unsaturated chains of DOPG prevent efficient packing of the DMPC chains. Consequently the peaks for both lipids are equally narrow before adding the peptides (, left). The NMR data for the membranes in the presence of the peptides suggest that R6 and PKKSRR, when added to the DMPC/DOPG membranes, sequester DOPG from the bulk lipid membrane leaving behind in essentially gel-phase domains having similar characteristics and NMR properties to pure DMPC. Broadening of the lines does not occur in the spectra of DOPC/DOPG membranes after the addition of these peptides () because both lipids are unsaturated and will remain fluid even if segregated into domains. It should be noted, however, that such segregation of lipids will be transitory at physiological temperatures owing to the highly dynamic nature of the bilayer, with the peptides diffusing across the surface of the membrane.
Figure 4. An illustration of the effect of lipid phase separation on the 31P MAS NMR spectra of DMPC/DOPG membranes. The diagram at the top illustrates the composition of the homogeneously mixed vesicles prepared from DMPC/DOPG in a 2:1 molar ratio (left) and from a mixture of preformed DMPC and preformed DOPG vesicles in the same ratio (right). Spectra corresponding to the two samples are shown underneath each diagram.
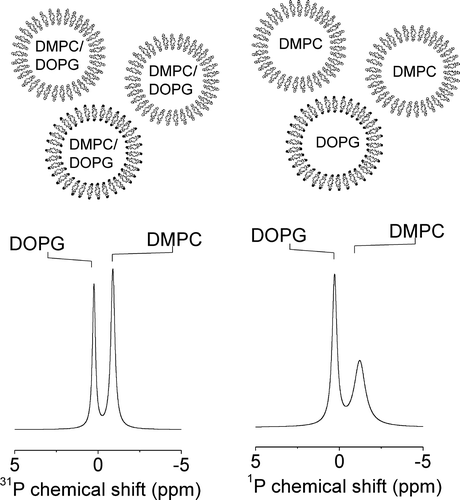
Bilayer integrity
It is possible that the interaction of the peptides with the membranes destroys the integrity of the bilayer and induces non-lamellar structures. Wide line 31P NMR was therefore employed to detect signals from the intrinsic lipid phosphate groups within mixed DMPC/DOPG MLVs in the absence and presence of the 5 hexapeptides. The line shapes provide an indication of the integrity of the lipid bilayer after the addition of the hexapeptide fragments and are diagnostic of any non-bilayer phases that might be adopted following peptide binding. These experiments were carried out at 30°C to avoid any phase separation of the lipid components after adding the peptides. The spectrum of a membrane sample in the absence of peptide is characteristic of lamellar, liquid crystalline lipids (a). Addition of R6, KKKKKR, PKKSRR and KKRKRK to a lipid/peptide molar ratio of 20:1 resulted in the appearance of a minor narrow central component consistent with the emergence of a small population (< 15%) of non-bilayer structures in addition to the predominating lamellar phase lipids. Similar minor components are often observed for pure lipid samples even in the absence of additives and may simply be disturbance arising from the physical agitation of the samples during preparation. The addition of K6 had no effect upon the spectrum, confirming that peptide binding did not induce the formation of non-bilayer structures such as hexagonal HII or cubic phases. Hence the peptides have little or no effect on the integrity of the lamellar structure of the vesicles. The spectra exhibited an increase in the apparent 31P chemical shift anisotropy (CSA) after the addition of the peptides (), which is typical of a charged membrane surface becoming partially or fully neutralized (Denisov et al. [Citation1998]). Apparent CSA values, measured from the outermost limits of the spectra, are given rather than individual CSA values for the two lipid species as the two components could not be resolved in the spectra.
Headgroup perturbations
The abundance of cationic residues in the peptides suggests that they interact with the surface of the membranes rather than penetrate within the lipid bilayer, so wide-line 2H NMR was employed to examine how the peptides affect the lipid head groups of the DMPC/DOPG membranes. The 2H NMR spectrum of headgroup deuterated phosphatidylcholine (PC) is highly sensitive to the reorientation of the choline moiety in adaptation to changes in membrane surface potential (Bonev et al. [Citation2000], Sixl & Watts [Citation1985], Scherer & Seelig [Citation1989]). Here 2H NMR was used to examine the interactions of the 5 hexapeptides with the DMPC/DOPG MLVs by exploiting DMPC-d4 as a reporter molecule. In (a–f) is a series of 2H NMR spectra at 30°C from the MLVs in the absence and presence of the 5 peptides. In the absence of peptide, the spectra exhibit superimposed Pake doublet powder patterns with quadrupole splittings (ΔνQ) of 9.8 kHz for the α-deuterons and 1.1 kHz for the β-deuterons of DMPC-d4. Upon addition of the peptides, first to a lipid/peptide ratio of 50:1 and then to 20:1, the value of ΔνQ for the α-deuterons decreased in a peptide concentration-dependent manner in all cases (). Such changes in the splittings are consistent with the peptides associating with the membrane surface and altering the orientation of the choline headgroups. The value of ΔνQ for the β-deuterons also decreased slightly after the addition of K6, KKKKKR and KKRKRK, whereas PKKSRR and R6 produced an increase in ΔνQ for the β-deuterons (). Of all the peptides, R6 gave rise to the most dramatic changes in splitting values, causing the spectral components for the α- and β-deuterons to converge into an apparently single component spectrum (b). The peptides therefore fall into two groups, classified as K6-like and R6-like according to how they affect the orientation of the choline headgroups. The K6-like peptides probably interact only weakly with the membrane surface and therefore give rise to only small changes in the spectra. The R6-like peptides may interact more intimately with the membranes and have a greater disruptive effect as a consequence of the bulky guanidinium group.
Figure 6. Wide-line 2H NMR spectra of MLVs of DMPC-d4/DOPG (2:1 molar ratio) at 30°C. Spectra are shown for the lipid sample alone (a) and after the addition of the 5 basic hexapeptides to a lipid/peptide molar ratio of 20:1 (b–f). The quadrupole splittings for deuterons at the α- and β-choline positions of DMPC-d4 are denoted by the separation between the dotted lines.
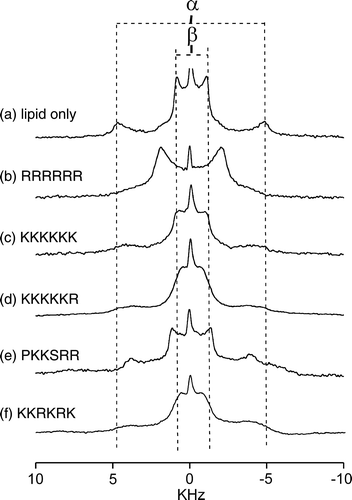
Discussion
Many small GTPases in the Ras and Rho families have a C-terminal PBR comprised of multiple lysines or arginines and there is substantial evidence that the PBR promotes association of these proteins with cellular membranes. For example, Rac1 mutants with deleted PBRs exhibit reduced membrane interactions and a concomitant loss of function and K-Ras-4B also exhibits a diminished association with cell membranes when the 6 contiguous lysines in the PBR are replaced with neutral glutamines (Hancock et al. [Citation1990], Kreck et al. [Citation1996]). A variety of biophysical methods including NMR, surface plasmon resonance, fluorescence digital imaging and electron paramagnetic resonance have been exploited to examine the behaviour of polybasic peptides with model phospholipid bilayers. To our knowledge there have been no systematic studies at the molecular level of how the Lys/Arg ratio within polybasic sequences of Rho GTPases influences their association with cell membranes and modulates the organization of the lipid components.
The associations of peptides derived from Rac1, Cdc42 and TCL with MLVs were examined here and compared with the membrane interactions of the model peptides K6 (also found in K-Ras4B) and R6, which does not feature in a naturally occurring lipid-binding motif identified to date. MLVs represent a convenient model of cell membranes that has been employed in a vast number of studies of basic peptide-membrane interactions using NMR and other techniques (e.g., Heerklotz et al. [Citation2004], Bonev et al. [Citation2000], Franzin & MacDonald [Citation2001]). The presence of multiple layers within each vesicle potentially raises issues about the interpretation of the data that requires clarification. The method of vesicle preparation employed here, involving repetitive freeze-thawing, increases the accessibility of the peptides to each of the layers within the vesicles, but it remains possible that the peptide is not distributed homogeneously within the vesicles, with peptide not exposed to some layers of vesicles. The 2H NMR spectra are not consistent with a non-uniform distribution of the peptides, however, because in such a situation the spectra would then reflect a superposition of line shapes from the layers exposed to the peptide and from the layers not in contact with the peptide (i.e., an essentially ‘lipid-only’ line shape). A lipid-only component would be characterized most prominently by the appearance of a second Pake doublet from the choline β-deuterons in the central region of the peptide. There is no evidence of a lipid-only component in any of the spectra obtained after adding peptide. The second issue regards the behaviour of peptides trapped in the inter-bilayer spaces within the vesicles. Typically, the inter-bilayer head-to-head spacing in MLVs is approximately 25 Å (Kinoshita et al. [Citation1998]) and the maximum length of the peptides is ∼20 Å. Peptides within this space could in principle interact simultaneously with lipid headgroups in two opposing bilayers in a ‘trans’ association, whereas clearly only a ‘cis’-association is possible for peptides outside the vesicles and, indeed, in the cellular environment. Although this possibility cannot be excluded by the current experimental data, it is reasonable to assume that a ‘trans’ association does not occur in the case of such short peptides. Only charged lysine and arginine side-groups at the two ends of a fully extended hexapeptide would be able to interact with two opposing bilayer surfaces, leaving the side-groups in the centre of the peptide unable to pair with negative charges. It is energetically more favourable for the peptides to associate with a single bilayer by lying across the charges surface and allowing all available charges on the peptide to pair with charges carried by the lipid head groups. Moreover, the NMR spectra do not show individual components corresponding to different types of membrane interactions by the external and trapped peptides.
The behaviour of lysine-rich peptides at negatively charged membrane surfaces is well understood. Short polylysine peptides (< 7 residues) associate with membranes through weak electrostatic interactions and are predicted to reside 5 Å away from the membrane surface (Victor & Cafiso [Citation2001]). Longer polylysine peptides associate more closely with the lipid polar head groups at the membrane interface, allowing the side-groups to penetrate deeper into the lipid bilayer (Kleinschmidt & Marsh [Citation1997]). A 2H NMR study of the effects of the long polylysine peptide K100 on mixed neutral/anionic lipid bilayers found that the peptides changed the lipid head group angle and segregated the lipids into domains that were long-lived on the 2H quadrupolar timescale (10-4 s), as characterized by the introduction of a second component into the NMR spectra (Franzin & MacDonald [Citation2001]). In the same work it was shown that pentalysine (K5) does not invoke domain formation, consistent with a weak electrostatic interaction of the much shorter peptide with the membrane surface. It is therefore expected that K6 interacts rather weakly with the surface of DMPC/DOPG membranes, and this prediction is substantiated here by the small changes in 2H quadrupolar splittings and 31P line widths for DMPC upon binding of the peptide. The peptides derived from Rac1 and TCL induce similarly minor changes in the spectra, which suggests that, like K6, they reside some distance away from the polar headgroups where they influence the lipids only weakly. On the other hand, R6 and the Cdc42-derived peptide PKKSRR gave rise to larger changes in the 2H spectra and selective broadening of the 31P peak for DMPC in the 31P MAS NMR spectra. Guanidinium-rich oligo/polymers have a high affinity for cell membranes and several studies of cellular and model membranes have shown that arginine-rich peptides are able to cross lipid bilayers in the presence of various anionic species (Persson et al. [Citation2004], Thorén et al. [Citation2003]). It is suggested here that R6 and PKKSRR penetrate beyond the Gouy-Chapman-like double layer into the polar region of the lipid bilayer where the charged side groups align with the phosphate groups. Such intimate contact could lead to the segregation of the lipids into short-lived domains or substantial perturbation of the choline headgroups by the bulky guanidine moieties of the contiguous arginine residues. By contrast, the abundance of lysine in K6, KKKKKR and KKRKRK may dominate the properties of these peptides and moderate the effects of arginine.
There is substantial evidence to show that the localization, membrane shuttling rate and function of the small RhoGTPases differ from each other. For example, Rac1, Cdc42 and TCL are all localized at intracellular membranes and the latter two proteins have been implicated in intracellular trafficking. Cdc42 is localized predominantly in the Golgi complex in mammalian cells, whereas TCL and Rac1 localise to the plasma membrane and early/sorting endosomes (de Toledo et al. [Citation2003], Cerione [Citation2004]). In the context of membrane attachment, these proteins differ in the polybasic region and the way it is isoprenylated, with both factors possibly affecting the details of the localization and function. Furthermore, the importance of the polybasic regions is evident from the distribution of Rac1 and Rac2; both proteins are identically isoprenylated, yet Rac1 is localized in the plasma membrane whereas the Rac2 is present in the endocytic membrane. Their polybasic C-terminus regions differ, with Rac1 containing six basic residues whereas Rac2 only three. The results obtained here offer an explanation of why these two Rac proteins might be differentially localized in the cell. Furthermore, data presented here could also explain why the RhoGTPases shuttle between the cytosol and membrane at different rates.
In summary, this work has shown that sequential differences in the PBRs of Rho GTPases can modulate the extent to which these regions alone affect the structure and organization of phospholipids in model membranes. It must be recognized that this study was based solely on the model peptides in isolation from their carrier signalling complexes. In reality, it may well be that subtle difference in overall fold and the sequence of PBR flanking regions may play far greater role in determining the mechanism of signalling complex interaction with target membranes or anchored protein complexes in general. Moreover, the role of the PBR as a recognition site for other protein complexes involved in trafficking and other cellular functions cannot be disregarded. Nevertheless, this work suggests that the functions of Rho GTPases in trafficking and sorting, and their membrane localization within the cell, may involve organization of lipid components and the role of the PBR in these processes warrants further investigation at the molecular and cellular level using unilamellar vesicles or planar membranes, for example.
Acknowledgements
The BBSRC is acknowledged for grant JR00UMLI and for funding in support of the NMR facility.
References
- Bonev BB, Watts A, Bokvist M, Grobner G. Electrostatic-lipid interactions of amyloid-beta peptide and pentalysine with membrane surfaces monitored by 31P MAS NMR. Phys Chem Chem Phys 2001; 3: 2904–2910
- Bonev BB, Chan WC, Bycroft BW, Roberts GCK, Watts A. Interaction of the Lantibiotic Nisin with Mixed Lipid Bilayers: A 31P and 2H NMR Study. Biochemistry 2000; 39: 11425–11433
- Cerione RA. Cdc42: new roads to travel. Trends Cell Biol. 2004; 14: 127–132
- Davis JH, Jeffrey KR, Bloom M, Valic MI, Higgs TP. Quadrupolar echo deuteron magnetic resonance spectroscopy in ordered hydrocarbon chains. Chem Phys Lett 1976; 42: 390–394
- de Toledo M, Senic-Matuglia F, Salamero J, Uze G, Comunale F, Fort P, Blangy A. The GTP/GDP cycling of Rho GTPase TCL is and essential regulator of the early endocytic pathway. Mol Biol Cell 2003; 14: 4846–4856
- Denisov G, Wanaski S, Luan P, Glaser M, McLaughlin S. Binding of basic peptides to membranes produces lateral domains enriched in the acidic lipids phosphatidylserine and phosphatidylinositol 4,5-bisphosphate: An electrostatic model and experimental results. Biophys J 1998; 74: 731–744
- Etienne-Manneville S, Hall A. Cdc42 regulates GSK-3 beta and adenomatous polyposis coli to control cell polarity. Nature 2002; 420: 629–635
- Finkielstein CV, Overduin M, Capelluto DGS. Cell migration and signaling specificity is determined by the phosphatidylserine recognition motif of Rac1. J Biol Chem 2006; 281: 27317–27326
- Franzin CM, MacDonald PM. Polylysine-induced deuterium NMR-observable domains in phosphatidylserine/phosphatidylcholine lipid bilayers. Biophys J 2001; 81: 3346–3362
- Hancock JF, Paterson H, Marshall CJ. A polybasic domain or palmitoylation is required in addition to the CAAX motif to localize P21RAS to the plasma membrane. Cell 1990; 63: 133–139
- Heerklotz H, Wieprecht T, Seelig J. Membrane perturbation by the lipopeptide surfactin and detergents as studied by deuterium. J Phys Chem B 2004; 108: 4909–4915
- Kinoshita K, Furuike S, Yamazaki M. Intermembrane distance in multilamellar vesicles of phosphatidylcholine depends on the interaction free energy between solvents and the hydrophilic segments of the membrane surface. Biophys Chem 1998; 74: 237–249
- Kleinschmidt JH, Marsh D. Spin-label electron spin resonance studies on the interactions of lysine peptides with phospholipid membranes. Biophys J 1997; 73: 2546–2555
- Kreck ML, Freeman JL, Abo A, Lambeth JD. Membrane association of Rac is required for high activity of the respiratory burst oxidase. Biochemistry 1996; 35: 15683–15692
- Lanning CC, Daddona JL, Ruiz-Velasco R, Shafer SH, Williams CL. The Rac1 C-terminal polybasic region regulates the nuclear localization and protein degradation of Rac1. J Biol Chem 2004; 279: 44197–44210
- Madine J, Doig AJ, Middleton DA. A study of the regional effects of alpha-synuclein on the organization and stability of phospholipid bilayers. Biochemistry 2006; 45: 5783–5792
- Persson D, Thoren PE, Lincoln P, Norden P. Vesicle membrane interactions of penetratin analogues. Biochemistry 2004; 43: 11045–11055
- Pinheiro TJT, Watts A. Resolution of individual lipids in mixed phospholipid membranes and specific lipid-cytochrome c interactions by magic-angle spinning solid-state phosphorus-31 NMR. Biochemistry 1994; 33: 2459–2467
- Rosen H. A modified ninhydrin colorimetric analysis of amino acids. Arch Biochem Biophys 1957; 67: 1–15
- Roy MO, Leventis R, Silvius JR. Mutational and biochemical analysis of plasma membrane targeting mediated by the farnesylated, polybasic carboxy terminus of K-ras4B. Biochemistry 2000; 39: 8298–307
- Scherer PG, Seelig J. Electric charge effects on phospholipid headgroups – phosphatidylcholine in mixtures with cationic and anionic amphiphiles. Biochemistry 1989; 28: 7720–7728
- Silvius JR. Mechanisms of Ras protein targeting in mammalian cells. J Membr Biol 2002; 190: 83–92
- Sixl F, Watts A. Deuterium and phosphorus nuclear magnetic resonance studies on the binding of polymixin-B to lipid bilayer-water interfaces. Biochemistry 1985; 24: 7906–7910
- Thorén PE, Persson D, Isakson P, Goksor M, Onfelt A, Norden B. Uptake of analogs of penetratin, Tat(48–60) and oligoarginine in live cells. Biochem Biophys Res Commun 2003; 307: 100–107
- Van Aelst L, D'Souza-Schorey C. Rho GTPases and signaling networks. Genes Dev 1997; 11: 2295–2322
- Victor KG, Cafiso DS. Location and dynamics of basic peptides at the membrane interface: Electron paramagnetic resonance spectroscopy of tetramethyl-piperidine-N-oxyl-4-amino-4-carboxylic acid-labeled peptides. Biophys J 2001; 81: 2241–2250
- Williams CL. The polybasic region of RAS and Rho family small GTPases: a regulator of protein interactions and membrane association and a site of nuclear localisation sequences. Cell Signal 2003; 15: 1071–1080