Abstract
Membrane-bound pyrophosphatases (PPases) are involved in the adaption of organisms to stress conditions, which was substantiated by numerous plant transgenic studies with H+-PPase yet devoid of any correlated evidences for other two subfamilies, Na+-PPase and Na+,H+-PPase. Herein, we demonstrate the gene cloning and functional evaluation of the membrane-bound PPase (CmPP) of the human gut microbe Clostridium methylpentosum. The CmPP gene encodes a single polypeptide of 699 amino acids that was predicted as a multi-spanning membrane and K+-dependent Na+,H+-PPase. Heterologous expression of CmPP could significantly enhance the salt tolerance of both Escherichia coli and Saccharomyces cerevisiae, and this effect in yeast could be fortified by N-terminal addition of a vacuole-targeting signal peptide from the H+-PPase of Trypanosoma cruzi. Furthermore, introduction of CmPP could remarkably improve the salt tolerance of tobacco, implying its potential use in constructing salt-resistant transgenic crops. Consequently, the possible mechanisms of CmPP to underlie salt tolerance are discussed.
Introduction
Pyrophosphate (PPi) is proposed as the major energy source for both the early life and the contemporary organisms under conditions of energy stresses (Baykov et al., Citation2013; Holm & Baltscheffsky, Citation2011; Serrano et al., Citation2007). Among those PPi-energizers, a number of membrane-bound pyrophosphatases (PPases) are used as primary pumps of H+ and/or Na+ to generate electrochemical ion gradients for ATP production and substance translocation across membranes (Baykov et al., Citation2013; Gaxiola et al., Citation2007; Serrano et al., Citation2007). Current studies have classified them into three homologous subfamilies: H+-PPase, Na+-PPase, and Na+,H+-PPase, depending on their transported cation specificity. Phylogenetic analyzes indicate that H+-PPase and Na+,H+-PPase evolved independently, with Na+-PPase as the common predecessor (Baykov et al., Citation2013). All membrane PPases form a functional homodimer of the single polypeptide (∼75 kDa), and share the conserved membrane topology (16 ∼ 17 transmembrane α-helixes) and critical amino acid residues, despite some structural details to impart functional divergence (Baykov et al., Citation2013; Tsai et al., Citation2014).
The membrane PPases can be further grouped into K+-dependent and K+-independent families, as determined by the residue (Ala or Lys) at a specific position (Belogurov & Lahti, Citation2002). All Na+-PPases and Na+,H+-PPases uniquely found in prokaryotes are K+-dependent and require Na+ for PPi hydrolysis (Malinen et al., Citation2007; Luoto et al., Citation2011, Citation2013a). Na+ concentration influences their transport activity and/or cation specificity. For instances, Na+-PPases can pump H+ at subphysiological Na+ concentrations (<10 mM) (Luoto et al., Citation2013b). Recently, an accurate phylogenetic map was elegantly constructed to delineate the interface of Na+-PPases and Na+,H+-PPases, and the latter were further identified into two subtypes as “true Na+,H+-PPases” and “Na+-regulated Na+,H+-PPases”, according to the response of their H+-pumping activity to increasing Na+ concentration in the range of 0.5 ∼ 100 mM (Nordbo et al., Citation2016). Interestingly, metagenomic analysis revealed many genes for Na+,H+-PPase or Na+-PPase in the major microbes colonizing the human gut, some of which were virtually verified (Luoto et al., Citation2013a).
Nowadays, the implication of membrane-bound PPases in the life adaption to stress conditions is well evidenced especially for H+-PPase. Introduction of plant H+-PPase makes Escherichia coli cells tolerant to diverse abiotic stresses (Yoon et al., Citation2013) and confers salt tolerance to Saccharomyces cerevisiae strains deficient in Na+/H+ exchanger (Gaxiola et al., Citation1999). Particularly, numerous H+-PPase-overexpressing transgenic plants demonstrate improved tolerance to salt, drought, chilling, nutrition (e.g. nitrogen, phosphorus, potassium) deprivation, and heavy metal environment (Bao et al., Citation2009; Gaxiola et al., Citation2012; Gouiaa & Khoudi, Citation2015; Khoudi et al., Citation2012; Liu et al., Citation2011, Pasapula et al., Citation2011; Paez-Valencia et al., Citation2013; Schilling et al., Citation2014, Citation2017; Wei et al., Citation2011; Yang et al., Citation2007; Zhang et al., Citation2011). Many of those engineered plants even outperform controls with greater yields or biomass under normal and stress conditions. Thus, H+-PPase has emerged as a promising tool to create stress-resistant organisms. Contrastively, Na+-PPase and Na+,H+-PPase have never been reported for uses in constructing stress-resistant organisms. In this study, we presented the gene cloning of the membrane-bound PPase (CmPP) of the human gut microbe Clostridium methylpentosum, a putative candidate of Na+,H+-PPase (Luoto et al., Citation2013a). We also conducted a functional evaluation for the salt tolerance of heterologous CmPP in E. coli, S. cerevisiae, and tobacco.
Materials and methods
Gene amplification of CmPP from the human gut microbial metagenome
According to the known contig sequence of C. methylpentosum DSM 5476 (GenBank Acc. No. ACEC01000124), two pairs of primers () were designed for gene amplification of CmPP. Using the human gut microbial metagenome freshly extracted from feces as the DNA template, the first round of PCR was conducted with DreamTaq DNA polymerase (Thermo Scientific, USA) and primers CmPP-Fw/CmPP-Rv. Then, 0.5 μl aliquot of the product was directly taken as the template for the second round of PCR with the nested primers CmPP-5Nd/CmPP-3Sc.
Table 1. Primers used in this study.
Construction of CmPP vectors
The gel-purified CmPP PCR product was dually digested with NdeI and SacI, then subcloned into plasmid pET32a(+) (Novagen, USA) to generate the prokaryotic expression vector pET(CmPP) that was verified by sequencing.
Subsequently, plasmid pET(CmPP) was sequentially treated with NdeI, Klenow, and XhoI. The excised CmPP fragment was then ligated with plasmid pYES2 (Invitrogen, USA) pretreated with BamHI, Klenow, XhoI in turn, to create the yeast expression vector pYES2(CmPP). Afterwards, the synthetic DNA fragment (109 bp) encoding the putative N-terminal signal peptide (SPtc) of the H+-PPase from the kinetoplastid protist Trypanosoma cruzi (Drake et al., Citation2010; Pérez-Castiñeira et al., Citation2011) was in-frame inserted into plasmid pYES2(CmPP) at the BamHI site just behind the start codon of CmPP to achieve the yeast chimaeric expression vector pYES2(SPtc-CmPP).
Furthermore, the CmPP fragment was re-amplified from plasmid pET(CmPP) by primers CmPP-5Sm/Tt7Dw-Rv (). The purified product was then cut by Sac I and ligated with SmaI/SacI-double digested vector pBI121 to generate the plant binary vector pBI(CmPP).
Dot-plating test and spectrometric curve analysis of E. coli growth under salt stress
E. coli strain BL21(DE3) (Novagen, USA) containing the recombinant vector pET(CmPP) or the empty vector pET32a(+) was grown in LB medium (+100 μg ampicillin ml−1) to an OD600 of 0.4 at 37 °C, then induced overnight at 25 °C with 0.5 mM IPTG (Sigma, USA). Subsequently, the induced bacterial cells were subjected to two trials: (1) after adjusting OD600 to 1.4, 2 μl of each serial dilution (1-, 5-, 10-, 20-, 40-fold) was dot-plated in a row on solid LB medium (containing 100 μg ampicillin ml−1 and 0.5 mM IPTG) with different final concentration of NaCl [0.17 M (CK, the intrinsic NaCl content of LB), 0.7 M, 0.8 M, 0.9 M], and incubated at 37 °C for 1 ∼ 2 d; (2) cells were precipitated by centrifugation at 10,000 rpm, 4 °C for 5 min, resuspended into liquid LB medium with the same additives in trial (1), adjusted with OD600 to 0.6, and cultured at 30 °C. The OD600 value was measured every 1 h.
Dot-plating test and spectrometric curve analysis of S. cerevisiae growth under salt stress
Yeast transformation was done with the LiCl method. Salt-sensitive S. cerevisiae mutant strain ena1- (Gaxiola et al., Citation1999) containing the recombinant vector pYES2(CmPP) or the empty vector pYES2 was grown at 30 °C in YPD medium (1% yeast extract, 2% peptone, 2% dextrose) to an OD600 of 0.4, then precipitated by centrifugation at 10,000 rpm, 4 °C for 5 min, resuspended and induced in YPG medium (1% yeast extract, 2% peptone, 2% galactose) for 24 h at 30 °C. Subsequently, the induced yeast cells were assigned for two trials: (1) After adjusting OD600 to 2, 2 μl of each serial dilution (1-, 5-, 10-, 20-, 40-fold) was dot-plated in a row on solid YPG medium with different concentration of NaCl [0 M (CK), 0.2 M, 0.25 M, 0.3 M], and incubated at 30 °C for 2 ∼ 4 d; (2) a small amount of cells was added into liquid YPG medium with the same salt additives in trial (1), adjusted with OD600 to 0.2, and cultured at 30 °C. The OD600 value was measured every 4 h. Likewise, the yeast dot-plating test for the chimaeric vector pYES2(SPtc-CmPP) in parallel to pYES2(CmPP) and pYES2 was also conducted, but under a wide range of high salt stresses (0.4 ∼ 1.1 M NaCl). Meanwhile, their growth curves at three selected salt conditions (0.3 M, 0.9 M, 1.1 M NaCl) were spectrometrically analyzed.
Generation of CmPP transgenic tobacco
Agrobacterium tumefaciens strain LBA4404 carrying the binary vector pBI(CmPP) was transformed to sterile tobacco explants by leaf disc infiltration. Kanamycin-resistant transgenic tobacco plants were strictly identified by multiplex PCR with reciprocally combinatorial primers derived from the coding region of CmPP and the backbone of vector pBI121, e.g. CmPP-iFw/CmPP-iRv, 35SPro-Fw/CmPP-iRv, and CmPP-iFw/NosTer-Rv ().
Assessment of the salt tolerance of CmPP transgenic tobacco
Three CmPP transgenic lines (CmPP-9, CmPP-11, CmPP-12) as well as the wild-type tobacco were selected for salt treatments and subsequent assays with two replicates as follows. In brief, the small plants with initially identical growth were daily irrigated in the greenhouse with certain amounts of water containing different concentration (0 mM [CK or untreated], 300 mM, 500 mM) of NaCl. After 30 d, samples were taken for several relevant physiological analyzes including: (1) Leaf chlorosis test, by submerging the leaf discs of normally growing (untreated) plants into different concentration (0 mM [CK], 500 mM, 1000 mM) of NaCl solutions for 5 d. (2) Measurements of the total chlorophyll content (mg·g−1 DW), root activity (mg·g−1·h−1 DW), leaf malondialdehyde (MDA) content (μmol·g−1 DW), and leaf electrolyte leakage (%), according to the protocols described by Arnon (Citation1949), Zhong and Huang (Citation2005), Peever and Higgins (Citation1989), and Gibon et al. (Citation1997), respectively.
Heterologous CmPP expression in yeast and tobacco analyzed by semi-quantitative reverse transcription PCR (RT-PCR)
The recombinant S. cerevisiae ena1- strains harboring the empty vector pYES2, the expression vector pYES2(CmPP) or pYES2(SPtc-CmPP) were induced for expression at 30 °C for 24 h as mentioned above, and then their total RNA was prepared using the yeast RNA extraction kit (Omega, USA). By using the random primers and M-MLV RTase (Promega, USA), aliquots of the RNA samples were reversely transcribed as the cDNA templates for PCR identification of CmPP transcriptional expression by primers CmPP-iFw/CmPP-iRv in S. cerevisiae. Meanwhile, the S. cerevisiae 18 S rRNA gene was used as the internal gene expression reference and analyzed with PCR primers Sc18S-iFw/Sc18S-iRv ().
Total RNA from the salt (500 mM NaCI)-treated transgenic tobacco lines (CmPP-9, CmPP-11, CmPP-12) were extracted using the Trizol reagent (Invitrogen, USA). By using the Oligo(dT) primers and M-MLV RTase, aliquots of the RNA samples were reversely transcribed as the cDNA templates for PCR identification of CmPP transcriptional expression by primers CmPP-iFw/CmPP-iRv in tobacco. Meanwhile, the tobacco 18 S rRNA gene was used as the internal gene expression reference and analyzed with PCR primers Nt18S-iFw/Nt18S-iRv ().
The PCR bands of CmPP, yeast 18 S rRNA and tobacco 18 S rRNA (546 bp, 449 bp, 552 bp, respectively) on gels were gray-densitometrically quantified by the program “Quantity One” (Bio-Rad, USA). Subsequently, the values of CmPP were normalized against those of yeast 18 S rRNA or tobacco 18 S rRNA, in which the highest data was further standardized as 100 to obtain the relative expression levels of CmPP in yeast or tobacco for comparison.
Bioinformatic and statistical analysis
Primer design, DNA and protein sequence analysis, and vector construction were assisted by the software Vector NT suite 11.5 (Invitrogen, USA). Protein membrane-topological prediction was done with the online program MEMSAT3 (bioinf.cs.ucl.ac.uk/psipred/) (Buchan et al., Citation2013). Sequence alignments and phylogenetic tree analysis with the neighbor-joining method were performed on the web server of NCBI (http://www.ncbi.nlm.nih.gov). Experimental data statistics and plots were finished with Microsoft Excel.
Results
Gene cloning and bioinformatic analysis of CmPP from C. methylpentosum
Using the extracted metagenome of human gut microbes as template, the first round of PCR for the CmPP gene did not give out a visible product of the expected size (∼2.3 kb). However, the second round of PCR with the nested primers could efficiently amplify the CmPP gene with correct size (∼2.1 kb) and adequate amounts (data not shown).
The CmPP PCR fragment was directly subcloned into plasmid pET32a(+) to achieve its E. coli expression vector pET(CmPP). Sequencing data indicated the amplified CmPP gene contained an ORF (2100 bp) encoding a single polypeptide of 699 aa (amino acids), with the G + C percentage of 59.9%, relatively balanced base dispersion, and no eukaryotic polyadenylation signals (AATAAA). A pairwise alignment of CmPP with its cognate contig (Acc. No. ACEC01000124) revealed 13 nucleotide changes (T↔C or A→G) but only 2 aa alterations (L122S, N294Y) at the uncritical positions (Supplementary Figure S1).
CmPP was topologically predicted as a multi-spanning membrane protein consisting of 16 transmembrane helix segments (S1–S16), with no signal peptide and both short terminals extruding into the side opposite to the cytoplasm (). The protein sequence alignments indicated that the residues of position 474 in CmPP, 475 in C. leptum Na+,H+-PPase, 460 in Carboxydothermus hydrogenoformans H+-PPase, and 537 in Vigna radiate H+-PPase were the common Ala (), implying CmPP as a member of K+-dependent PPase (Belogurov & Lahti, Citation2002; Luoto et al., Citation2011). In addition, our phylogenetic tree analysis (data not shown) was also in concordance with the previous evolutional predictions (Luoto et al., Citation2013a; Nordbo et al., Citation2016). Therein, CmPP was very close to the membrane-integral PPase (Acc. No. ZP_02078667) of the anaerobiotic C. leptum (coexisted with C. methylpentosum in human gut) that has been identified as a Na+-regulated Na+,H+-PPase (Nordbo et al., Citation2016).
Figure 1. The membrane topology of CmPP predicted by the online program MEMSAT3 (A), and the feature residue determination for K+-dependence of CmPP by sequence alignment (B). CmPP contains 16 transmembrane helix segments (S1–S16) with distinct numberings. The feature residue Ala (A) for membrane PPase K+-dependence is boxed.
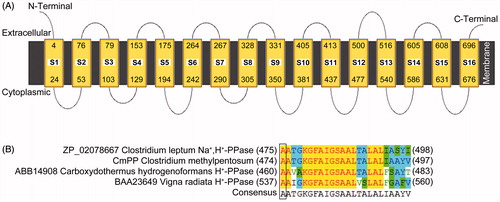
Heterologous expression of CmPP can improve the salt tolerance of E. coli
The function of CmPP was preliminarily evaluated by its heterologous expression in E. coli that is intrinsically devoid of membrane PPases (Luoto et al., Citation2011). From the bacterial dot-plating tests, E. coli strain BL21(DE3) harboring the recombinant vector pET(CmPP) demonstrated a similar colony size upon no salt stress [0.17 M NaCl (CK)] but a significantly better growth status under all appointed salt stresses (0.7 M, 0.8 M, 0.9 M NaCl), as compared with that carrying the empty control vector pET32a(+) (). Furthermore, the spectrometric analysis of the bacterial growth curve also indicated the recombinant E. coli strain of pET(CmPP) grew distinctly faster than the control strain of pET32a(+) under different salt stresses (0.7 M, 0.9 M NaCl) (). Taken together, these results explicitly pointed to a remarkable role of the heterologous CmPP to improve the salt tolerance of E. coli.
Figure 2. Salt tolerance of E. coli could be improved by heterologous expression of CmPP, as judged by comparing the growth of E. coli strain BL21(DE3) harboring the recombinant vector pET(CmPP) or the empty vector pET32a(+) under salt stresses of different NaCl concentrations. (A) Dot-plating test with serial dilutions (1-, 5-, 10-, 20-, 40-fold) for the colony growth of E. coli; (B) Spectrometric analysis for the dynamic growth curve of E. coli. The control (CK) means the intrinsic NaCl concentration (0.17 M) in LB medium. Error bars represent the SD of three separate trials with different clones of each E. coli.
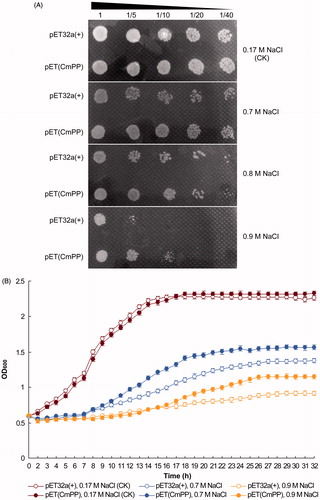
Heterologous expression of CmPP can restore the salt tolerance of S. cerevisiae ena1- strain
The function of CmPP was further investigated in the salt-sensitive mutant strain ena1- of S. cerevisiae (inherently lacking membrane PPases) that is apparently inhibited for growth by 0.2 M NaCl (Gaxiola et al., Citation1999). From the yeast dot-plating tests, the S. cerevisiae ena1- strain carrying the recombinant vector pYES2(CmPP) exhibited a nearly identical colony size upon no salt stress [0 M NaCl (CK)] but a notably better growth status under all chosen salt stresses (0.2 M, 0.25 M, 0.3 M NaCl), as compared with that containing the empty control vector pYES2 (). Moreover, the spectrometric analysis of yeast growth curve also demonstrated that the recombinant ena1- strain of pYES2(CmPP) grew definitely faster than the control strain of pYES2 under any salt stresses (0.2 M, 0.3 M NaCl) (). Conclusively, these results indicated that the heterologously expressed CmPP could restore the salt tolerance of S. cerevisiae ena1- strain.
Figure 3. Salt tolerance of S. cerevisiae could be enhanced by heterologous expression of CmPP, as assessed by comparing the growth of salt-sensitive yeast strain ena1- carrying the recombinant vector pYES2(CmPP) or the empty vector pYES2 under salt stresses of different NaCl concentration. (A) Dot-plating test with serial dilutions (1-, 5-, 10-, 20-, 40-fold) for the colony growth of S. cerevisiae; (B) Spectrometric analysis for the dynamic growth curve of S. cerevisiae. The control (CK) means the intrinsic NaCl concentration (0 M) in YPG medium. Error bars represent the SD of three separate trials with different clones of each yeast strain.
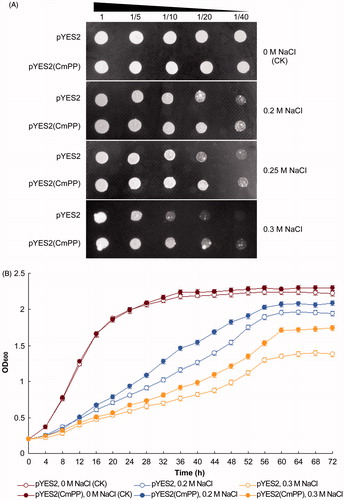
CmPP-mediated salt tolerance in S. cerevisiae can be fortified by N-terminal addition with the signal peptide of T. Cruzi H+-PPase
As reported previously, the signal peptide of T. cruzi H+-PPase, as a N-terminal fusion partner, could not only substantially increase the expression levels of heterologous membrane-bound H+-PPases of different organisms (from bacteria to plants) in S. cerevisiae, but also alter the subcellular distribution of the H+-PPase chimeras, preferentially towards the endomembrane fractions especially of vacuoles (Drake et al., Citation2010; Pérez-Castiñeira et al., Citation2011). Herein, we fused this signal peptide (SPtc) at the N-terminal of CmPP () and constructed the corresponding yeast chimaeric expression vector pYES2(SPtc-CmPP). From the in parallel yeast dot-plating tests, the S. cerevisiae ena1- strain harboring the chimaeric vector pYES2(SPtc-CmPP) showed an extreme tolerance to a wide range of high concentration of NaCl (0.4 ∼ 1.1 M), much better than that found in the recombinant strain of vector pYES2(CmPP). Meanwhile, upon these high salt stresses, the growth of the ena1- strain with the empty vector pYES2 was almost inhibited (). Additionally, when comparing the dynamic growth curves, the recombinant ena1- strain of pYES2((SPtc-CmPP) grew obviously faster than that of pYES2(CmPP) which was in turn with a higher growth rate than the control strain of pYES2 under all three selected salt stresses (0.3 M, 0.9 M, 1.1 M NaCl) (Supplementary Figure S2). Generally, these spectrometric profiles were consistent with the observations from the dot-plating tests (). We also evaluated the transcriptional expression of CmPP in these recombinant ena1- strains after 24 h induction by semi-quantitative RT-PCR, and found no significant variance between the strains of pYES2((SPtc-CmPP) and pYES2(CmPP) (Supplementary Figure S3), suggesting their differential salt tolerance is most likely relevant to the additive SPtc rather than CmPP expression. Overall, these results confirmed CmPP-mediated salt tolerance in S. cerevisiae, and also demonstrated a functional fortification for CmPP by N-terminal addition of a vacuole-targeting signal peptide such as SPtc of T. cruzi H+-PPase.
Figure 4. Fortification of CmPP-mediated salt tolerance in S. cerevisiae by N-terminal addition with a vacuole-targeting signal peptide. (A) The diagram of chimaeric CmPP with the signal peptide (SPtc) of T. cruzi H+-PPase, arrowhead indicates the putative cleavage site of SPtc; (B) Dot-plating test with serial dilutions (1-, 5-, 10-, 20-, 40-fold) to compare the colony growth of salt-sensitive S. cerevisiae strain ena1- harboring the chimaeric vector pYES2(SPtc-CmPP), the expression vector pYES2(CmPP), or the empty vector pYES2 under high salt stresses of different NaCl concentrations.
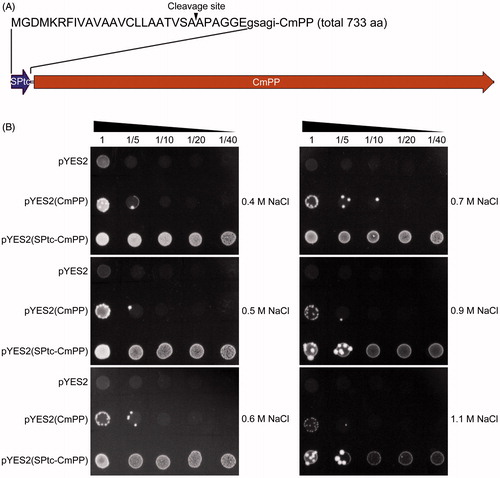
Transgenic introduction of CmPP can further enhance the salt tolerance of tobacco
We compared the salt tolerance of three CmPP transgenic lines (CmPP-9, CmPP-11, CmPP-12) vs. WT tobacco. Leaf discs from their normally growing plants were immerged into NaCl solutions lasting 5 d for a chlorosis test. As shown in , the chlorophyll decay in the leaf discs of all three transgenic lines and WT tobacco was hardly seen under no salt treatment (CK), but obviously displayed under salt treatments and became worse with increased NaCl concentration (500 mM vs. 1000 mM). Nonetheless, the chlorosis degree was remarkably slighter in CmPP transgenic lines than WT tobacco, especially under a more severe treatment of salt (1000 mM NaCl).
Figure 5. Leaf chlorosis comparison of three CmPP transgenic lines (CmPP-9, CmPP-11, CmPP-12) vs. WT tobacco, by submerging their leaf discs into salt solutions (0 mM (CK), 500 mM, 1000 mM NaCl) for 5 d.
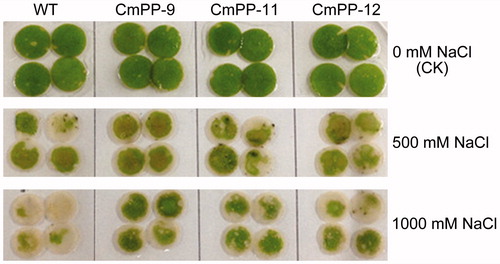
Plant growth is susceptible to high salt that can deteriorate photosynthesis, damage cell membrane and impair root activity. Thus, we further compared all three CmPP transgenic lines (CmPP-9, CmPP-11, CmPP-12) against WT tobacco, in terms of several relevant physiological aspects including the total chlorophyll content (), root activity (), leaf MDA content (), and leaf electrolyte leakage (). Under normal growing condition (without salt trearment, i.e. CK), all these physiological values were similar in both CmPP transgenic lines and WT tobacco. In contrast, decreased chlorophyll content and root activity as well as increased leaf MDA content and electrolyte leakage were found in either CmPP transgenic lines or WT tobacco under salt treatments, and these changes enlarged with higher NaCl stress (300 mM vs. 500 mM). Nevertheless, all these physiological alterations were notably weaker in CmPP transgenic lines than WT tobacco under any salt treatments. We also examined the transcriptional expression of CmPP in these transgenic tobacco lines treated with 500 mM NaCI by semi-quantitative RT-PCR, and found a remarkably higher level of CmPP expression in line CmPP-9 than other two similar lines CmPP-11 and CmPP-12 (), implying the predominant salt tolerance in line CmPP-9 (vs. CmPP-11, CmPP-12) () is probably attributed to a stronger CmPP expression. Collectively, these data suggested that introduction of the CmPP gene could efficiently improve the salt tolerance of tobacco.
Figure 6. Physiological comparison of three CmPP transgenic lines (CmPP-9, CmPP-11, CmPP-12) vs. WT tobacco under salt treatments (0 mM (CK), 300 mM, 500 mM NaCl), in respect of the total chlorophyll content (A), root activity (B), leaf MDA content (C), and leaf electrolyte leakage (D). Error bars represent the SD of three separate trials with different plants of each transgenic line or WT tobacco.
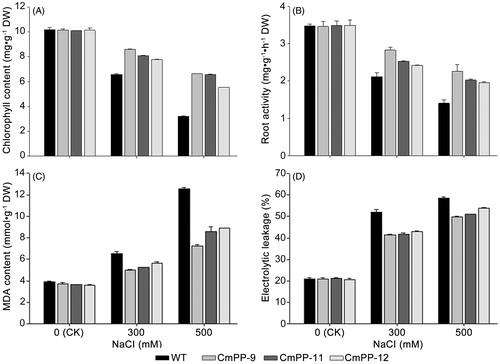
Discussion
The primeval life or the present organisms suffering with stress conditions appear to depend on PPi rather than ATP as the main source of energy, with an important PPi-energizer as the membrane-bound homodimeric PPases composed of H+-PPase, Na+-PPase, and Na+,H+-PPase (Baykov et al., Citation2013; Holm & Baltscheffsky, Citation2011; Serrano et al., Citation2007). The long known H+-PPase with broad distributions has been given with numerous functional characterizations and applications in constructing stress-resistant organisms particularly of plants (Gaxiola et al., Citation2007, Citation2012; Schilling et al., Citation2017), whereas no correlated reports have pointed to Na+-PPase, Na+,H+-PPase that were uniquely found in prokaryotes several years ago or recently (Luoto et al., Citation2011, Citation2013a; Malinen et al., Citation2007). Therefore, to our knowledge, this study seems for the first time to demonstrate a functional evaluation on a prokaryotic membrane-bound PPase with putative Na+-transporting activity.
Nowadays, various environmental microbiome have been increasingly utilized to mine the novel genes involved in stress resistance (Culligan et al., Citation2012; Guazzaroni et al., Citation2013; Kapardar et al., Citation2010). The gut of human and/or other animals is likely a convenient source to acquire the genes of microbial Na+-PPases and Na+,H+-PPases (Luoto et al., Citation2013a). However, probably due to the diversity and complexity of human gut microbiota (Arumugam et al., Citation2011; Eckburg et al., Citation2005; Gill et al., Citation2006), successful amplification of the CmPP gene and those encoding the membrane-bound PPases of other several gut microbes such as Bacteroides vulgatus and C. leptum, generally need two rounds of nested PCR. Furthermore, the human gut metagenome is continent or nationality-specific and influenced by diet, drug and other factors of the intake (Arumugam et al., Citation2011). Therefore, it is plausible that our cloned CmPP gene has a significant divergence in the nucleotide sequence (but with only minor and uncritical changes in amino acids) from the available data deposited in GenBank (Acc. No. ACEC01000124).
Phylogenetic analysis appointed CmPP as one of the closest orthologs of the membrane PPase of the human gut microbe C. leptum (Acc. No. ZP_02078667) that has been identified as a Na+-regulated Na+,H+-PPase (Luoto et al., Citation2013a; Nordbo et al., Citation2016). This subtype of Na+,H+-PPases was characterized with the progressive loss of H+ transport activity at high Na+ concentration (Nordbo et al., Citation2016). Nevertheless, CmPP is believed to at least possess the Na+-transporting activity. This basal feature can be used to explain the improved salt tolerance of E. coli, S. cerevisiae and tobacco imparted by heterologous CmPP (). In E. coli, the heterologously expressed CmPP might be translocated onto the plasma membrane and effectuate as an outward Na+ pump to drive Na+ efflux from the cytosol. This presumption was somewhat supported by the transporting activity analyzes of Na+-PPases or Na+,H+-PPases, using the inverted membrane vesicles (IMV) isolated from their recombinant E. coli cells (Luoto et al., Citation2011, Citation2013a). In S. cerevisiae, a similar action model as that in E. coli is speculated, but the main CmPP-mediated pathway to decrease the cytosolic Na+ level is conceived to be dominated by the vacuole-targeted CmPP that probably acts as an inward Na+-pump to sequester Na+ inside the vacuole lumen. This perception can be further confirmed by the functional promotion on CmPP via the N-terminal addition of a vacuole-targeting signal peptide (SPtc) from T. cruzi H+-PPase (, Drake et al., Citation2010; Pérez-Castiñeira et al., Citation2011). Very likely, this vacuolar route of CmPP working in yeast might be applicable to CmPP transgenic tobacco with increased salt tolerance. Moreover, probably due to an attribute of Na+-regulated Na+,H+-PPase, the tobacco vacuole-targeted CmPP might be unable to exert its H+-pumping activity that indirectly drive Na+ flux into vacuoles and alleviate cytosolic Na+ toxicity under salt stresses, as did the canonical H+-PPases reported in many previous studies (Bao et al., Citation2009; Gouiaa & Khoudi, Citation2015; Liu et al., Citation2011; Pasapula et al., Citation2011; Schilling et al., Citation2014).
In addition, our obtained CmPP gene has the approximate features (e.g. G + C %) of the eukaryotic genes (e.g. those from many common plants) and no cryptic polyadenylation signals (AATAAA), as compared with its AT-rich (> 66%) orthologs from other gut microbes such as Clostridium celatum and Clostridium sp. 7_2_43FAA (Luoto et al., Citation2013a). This advantage not only allowed us a successful gene amplification for CmPP from the complex gut metagenome (Arumugam et al., Citation2011), but also enabled the transgenic CmPP to convey an increased salt tolerance to tobacco ( and ). Rationally, this prokaryotic CmPP gene could be envisioned to extend its application in constructing transgenic crops with improved tolerance to salt stress.
IMBC_1370145_Supplementary_Material.pdf
Download PDF (1.1 MB)Acknowledgements
We thank Prof. Roberto A. Gaxiola (Arizona State University, USA) for a gift of the salt-sensitive S. cerevisiae mutant strain ena1- (L5709). We also thank reviewers for their instructional comments for revision.
Disclosure statement
The authors report no conflicts of interest. The authors alone are responsible for the content and writing of the paper.
Additional information
Funding
References
- Arnon DI. 1949. Copper enzymes in isolated chloroplasts. Polyphenoloxidase in Beta vulgaris. Plant Physiol 24:1–15.
- Arumugam M, Raes J, Pelletier E, Le Paslier D, Yamada T, Mende DR, et al. 2011. Enterotypes of the human gut microbiome. Nature 473:174–180.
- Bao AK, Wang SM, Wu GQ, Xi JJ, Zhang JL, Wang CM. 2009. Overexpression of the Arabidopsis H+-PPase enhanced resistance to salt and drought stress in transgenic alfalfa (Medicago sativa L.). Plant Sci 176:232–240.
- Baykov AA, Malinen AM, Luoto HH, Lahti R. 2013. Pyrophosphate-fueled Na+ and H+ transport in prokaryotes. Microbiol Mol Biol Rev 77:267–276.
- Belogurov GA, Lahti R. 2002. A lysine substitute for K+. A460K mutation eliminates K+ dependence in H+-pyrophosphatase of carboxydothermus hydrogenoformans. J Biol Chem 277:49651–49654.
- Buchan DWA, Minneci F, Nugent TCO, Bryson K, Jones DT. 2013. Scalable web services for the PSIPRED protein analysis workbench. Nucleic Acids Res 41:W349–W357.
- Culligan EP, Marchesi JR, Hill C, Sleator RD. 2012. Mining the human gut microbiome for novel stress resistance genes. Gut Microbes 3:394–397.
- Drake R, Serrano A, Pérez-Castiñeira JR. 2010. N-terminal chimaeras with signal sequences enhance the functional expression and alter the subcellular localization of heterologous membrane-bound inorganic pyrophosphatases in yeast. Biochem J 426:147–157.
- Eckburg PB, Bik EM, Bernstein CN, Purdom E, Dethlefsen L, Sargent M, et al. 2005. Diversity of the human intestinal microbial flora. Science 308:1635–1638.
- Gaxiola RA, Palmgren MG, Schumacher K. 2007. Plant proton pumps. FEBS Lett 581:2204–2214.
- Gaxiola RA, Rao R, Sherman A, Grisafi P, Alper SL, Fink GR. 1999. The Arabidopsis thaliana proton transporters, AtNhx1 and Avp1, can function in cation detoxification in yeast. Proc Natl Acad Sci USA 96:1480–1485.
- Gaxiola RA, Sanchez CA, Paez-Valencia J, Ayre BG, Elser JJ. 2012. Genetic manipulation of a “vacuolar H(+)-PPase: from salt tolerance to yield enhancement under phosphorus-deficient soils”. Plant Physiol 159:3–11.
- Gibon Y, Bessieres MA, Larher F. 1997. Is glycine betaine a non-compatible solute in higher plants that do not accumulate it?. Plant Cell Environ 20:329–340.
- Gill SR, Pop M, Deboy RT, Eckburg PB, Turnbaugh PJ, Samuel BS, et al. 2006. Metagenomic analysis of the human distal gut microbiome. Science 312:1355–1359.
- Gouiaa S, Khoudi H. 2015. Co-expression of vacuolar Na(+)/H(+) antiporter and H(+)-pyrophosphatase with an IRES-mediated dicistronic vector improves salinity tolerance and enhances potassium biofortification of tomato. Phytochemistry 117:537–546.
- Guazzaroni ME, Morgante V, Mirete S, González-Pastor JE. 2013. Novel acid resistance genes from the metagenome of the Tinto River, an extremely acidic environment. Environ Microbiol 15:1088–1102.
- Holm NG, Baltscheffsky H. 2011. Links between hydrothermal environments, pyrophosphate, na(+), and early evolution. Orig Life Evol Biosph 41:483–493.
- Kapardar RK, Ranjan R, Grover A, Puri M, Sharma R. 2010. Identification and characterization of genes conferring salt tolerance to Escherichia coli from pond water metagenome. Bioresour Technol 101:3917–3924.
- Khoudi H, Maatar Y, Gouiaa S, Masmoudi K. 2012. Transgenic tobacco plants expressing ectopically wheat H+-pyrophosphatase (H+-PPase) gene TaVP1 show enhanced accumulation and tolerance to cadmium. J Plant Physiol 169:98–103.
- Liu L, Wang Y, Wang N, Dong YY, Fan XD, Liu XM, et al. 2011. Cloning of a vacuolar H(+)-pyrophosphatase gene from the halophyte Suaeda corniculata whose heterologous overexpression improves salt, saline-alkali and drought tolerance in Arabidopsis. J Integr Plant Biol 53:731–742.
- Luoto HH, Belogurov GA, Baykov AA, Lahti R, Malinen AM. 2011. Na+-translocating membrane pyrophosphatases are widespread in the microbial world and evolutionarily precede H+-translocating pyrophosphatases. J Biol Chem 286:21633–21642.
- Luoto HH, Baykov AA, Lahti R, Malinen AM. 2013a. Membrane-integral pyrophosphatase subfamily capable of translocating both Na+ and H+. Proc Natl Acad Sci USA 110:1255–1260.
- Luoto HH, Nordbo E, Baykov AA, Lahti R, Malinen AM. 2013b. Membrane Na+-pyrophosphatases can transport protons at low sodium concentrations. J Biol Chem 288:35489–35499.
- Malinen AM, Belogurov GA, Baykov AA, Lahti R. 2007. Na+-pyrophosphatase: a novel primary sodium pump. Biochemistry 46:8872–8878.
- Nordbo E, Luoto HH, Baykov AA, Lahti R, Malinen AM. 2016. Two independent evolutionary routes to Na+/H+ cotransport function in membrane pyrophosphatases. Biochem J 473:3099–3111.
- Paez-Valencia J, Sanchez-Lares J, Marsh E, Dorneles LT, Santos MP, Sanchez D, et al. 2013. Enhanced proton translocating pyrophosphatase activity improves nitrogen use efficiency in Romaine lettuce. Plant Physiol 161:1557–1569.
- Pasapula V, Shen G, Kuppu S, Paez-Valencia J, Mendoza M, Hou P, et al. 2011. Expression of an Arabidopsis vacuolar H+-pyrophosphatase gene (AVP1) in cotton improves drought- and salt tolerance and increases fibre yield in the field conditions. Plant Biotechnol J 9:88–99.
- Peever TL, Higgins VJ. 1989. Electrolyte leakage, lipoxygenase, and lipid peroxidation induced in tomato leaf tissue by specific and non-specific elicitors from Cladosporium fluvum. Plant Physiol 90:867–875.
- Pérez-Castiñeira JR, Hernández A, Drake R, Serrano A. 2011. A plant proton-pumping inorganic pyrophosphatase functionally complements the vacuolar ATPase transport activity and confers bafilomycin resistance in yeast. Biochem J 437:269–278.
- Schilling RK, Marschner P, Shavrukov Y, Berger B, Tester M, Roy SJ, Plett DC. 2014. Expression of the Arabidopsis vacuolar H+-pyrophosphatase gene (AVP1) improves the shoot biomass of transgenic barley and increases grain yield in a saline field. Plant Biotechnol J 12:378–386.
- Schilling RK, Tester M, Marschner P, Plett DC, Roy SJ. 2017. AVP1: one protein, many roles. Trends Plant Sci 22:154–162.
- Serrano A, Pérez-Castiñeira JR, Baltscheffsky M, Baltscheffsky H. 2007. H+-PPases: yesterday, today and tomorrow. IUBMB Life 59:76–83.
- Tsai JY, Kellosalo J, Sun YJ, Goldman A. 2014. Proton/sodium pumping pyrophosphatases: the last of the primary ion pumps. Curr Opin Struct Biol 27:38–47.
- Wei A, He C, Li B, Li N, Zhang J. 2011. The pyramid of transgenes TsVP and BetA effectively enhances the drought tolerance of maize plants. Plant Biotechnol J 9:216–229.
- Yang H, Knapp J, Koirala P, Rajagopal D, Peer WA, Silbart LK, et al. 2007. Enhanced phosphorus nutrition in monocots and dicots over-expressing a phosphorus-responsive type I H+-pyrophosphatase. Plant Biotechnol J 5:735–745.
- Yoon HS, Kim SY, Kim IS. 2013. Stress response of plant H+-PPase expressing transgenic Escherichia coli and Saccharomyces cerevisiae: a potentially useful mechanism for the development of stress-tolerant organisms. J Appl Genet 54:129–133.
- Zhang J, Li J, Wang X, Chen J. 2011. OVP1, a vacuolar H+-translocating inorganic pyrophosphatase (V-PPase), overexpression improved rice cold tolerance. Plant Physiol Biochem 49:33–38.
- Zhong XH, Huang NR. 2005. Rice grain chalkiness is negatively correlated with root activity during grain filling. Rice Sci 12:192–196.