Abstract
GLUT11 (SLC2A11) is a class II sugar transport facilitator which exhibits highest similarity with the fructose transporter GLUT5 (about 42%). Here we demonstrate that separate exons 1 (exon 1A, exon 1B, and exon 1C) of the SLC2A11 gene generate mRNAs of three GLUT11 variants (GLUT11-A, GLUT11-B, and GLUT11-C) that differ in the amino acid sequence of their N-termini. All three 5′-flanking regions of exon 1A, exon 1B and exon 1C exhibited promoter activity when expressed as luciferase fusion constructs in COS-7 cells. 5′-RACE-PCR, quantitative real-time PCR, and Northern blot analysis performed with specific probes for exon 1A, 1B and 1C demonstrated that GLUT11-A is expressed in heart, skeletal muscle, and kidney, GLUT11-B in kidney, adipose tissue, and placenta, and GLUT11-C in adipose tissue, heart, skeletal muscle, and pancreas. Surprisingly, mice and rats lack the SLC2A11 gene. When expressed in Xenopus oocytes, all three GLUT11 isoforms transport glucose and fructose but not galactose. There was no apparent difference in the subcellular distribution of the three isoforms expressed in COS-7 cells. Our data indicate that different promoters and splicing of the human SLC2A11 gene generate three GLUT11 isoforms which are expressed in a tissue specific manner but do not appear to differ in their functional characteristics.
Introduction
The facilitative glucose transport into mammalian cells requires specific transmembrane proteins, the GLUT proteins. The GLUT family comprises 14 members and, according to sequence similarities, can be divided into three classes Citation[1]. The first class includes GLUT1–4 and the recently cloned GLUT14 Citation[2]. The fructose transporter GLUT5 and the three novel proteins GLUT7, GLUT9, and GLUT11 belong to the second class. GLUT6, GLUT8, GLUT10, GLUT12, and HMIT, a myoinositol transporter, belong to the third class Citation[1]. All GLUT proteins differ in their tissue distribution and in their substrate specificity. GLUT1–4 and the novel members GLUT6–9, 11, and 12 have been described to transport glucose but exhibit a different affinity for this substrate. GLUT5 is known to transport only fructose Citation[3], HMIT transports myoinositol in a pH dependent manner Citation[4]. Some of the genes (SLC2A) encoding for GLUT proteins appear to arise by duplication. Thus, the genomic organization of SLC2A6 and SLC2A8 is very similar and both genes are located in close proximity suggesting that one of these two genes has evolved by duplication Citation[5]. The comparison of the sequences of SLC2A3 and SLC2A14 also suggested that GLUT14 resulted from duplication of the GLUT3 encoding gene Citation[2]. The third example of gene duplication was noticed for SLC2A5, producing a gene encoding for GLUT7 which exhibits 68% identity with GLUT5 at the amino acid level Citation[1], Citation[2].
GLUT11 has been cloned and characterized by our group Citation[6]. Its closest relative is the fructose transporter GLUT5, sharing about 42% amino acid identity with GLUT11. Transfection of COS-7 cells with GLUT11 cDNA significantly increased the glucose transport activity reconstituted from membrane extracts, and this transport activity could be inhibited by fructose, suggesting that GLUT11 transports both glucose and fructose Citation[6]. We have previously described that in skeletal muscle GLUT11 is predominantly located in slow twitch fibers, in contrast to GLUT4 which is present along the plasma membrane of slow, and to a lesser degree of fast twitch fibers. The expression pattern of GLUT11 was not affected by endurance training, obesity, and type 2 diabetes, suggesting that the localization and the regulation of GLUT11 in skeletal muscle differs from that of GLUT4 Citation[7].
It has recently been described that the SLC2A11 gene produces transcripts that differ in their transcription start and in the N-terminus of the deduced amino acid sequence Citation[8], Citation[9]. In this paper, we characterize these transcripts as well as their expression, and the transport activity, substrate specificity, and subcellular distribution of their translation products.
Materials and methods
Sequencing
All cDNA clones and PCR products were sequenced in both directions by the method of Sanger using the ABI PRISM® 3100-Avant Genetic Analyzer in combination with the BigDye® Terminator v3.1 Cycle Sequencing Kit (Applied Biosystems, Foster City, CA, USA).
Northern blot analysis
Blots generated with poly-A RNA from different human tissues were purchased from Clontech Laboratories (Palo Alto, CA, USA). Probes derived from the GLUT11 cDNA (GenBank accession no. AJ271290) by PCR with specific primers (bp 1–624: forward: 5′-CGG ACC TGC CTC TCA CGC AAT GG-3′, reverse: 5′-CAC CTG TCC CAT CAC GAT CC-3′; bp 952–1608: forward: 5′-GAG CTC TGC GGG AAT GAC TC-3′, reverse: 5′-CTG GCC ACC CCT TTG GGA CTA GAG-3′) and probes derived from the genomic clone AP000350 for the specific detection of GLUT11-A-C (exon 1A: forward: 5′-TCC-TGG ATT CCT CCA TGC AG-3′, reverse: 5′-TCT TCG GAG CGC TCT CAT CTG-3′; exon 1B: forward: 5′-AGA GCC GGA ATT TGC GGG GA-3′, reverse: 5′-TGC GTC CGA GGC CGT AAG G-3′; exon 1C: forward: 5′-AGC GGC TCT TTC ACC ACT GG-3′, reverse: 5′-CAT TCT AGA TCG CAG GAG G-3′) were generated with the Klenow fragment of DNA polymerase I and [α-32P]dCTP by random oligonucleotide priming. The nylon membranes were hybridized at 68°C with ExpressHyb™ hybridization solution, and washed according to the instruction of the manufacturer (Clontech, Palo Alto, CA, USA) at both room temperature and 50°C.
5′-RACE-PCR
RACE-PCR was performed to determine the different 5′-ends of the SLC2A11 gene by the method of Chenchik and Siebert Citation[10] using human skeletal muscle and human pancreas Marathon-Ready™ cDNA in combination with Advantage® 2 Polymerase Mix (Clontech, Palo Alto, CA, USA). First PCR amplification was performed with primers corresponding to the 5′-adapter (5′-CCA TCC TAA TAC GAC TCA CTA TAG GGC-3′) and to a sequence of exon 3 (5′-CCA AGG GAC CTG CAA GCA GTG CTC C-3′) and used as a template for a nested PCR with a second 5′-adapter primer (5′-ACT CAC TAT AGG GCT CGA GCG GC-3′) and another primer specific for exon 3 (5′-GGT GAT CGG GCA GTG GCT CTC CAG TAC G-3′). PCR products were cloned using the TOPO™ TA Cloning® Kit (Invitrogen, Carlsbad, CA, USA) and sequenced.
RNA preparation and first strand cDNA synthesis
RNA was extracted from human abdominal and mamma fat using the RNeasy Mini Kit (Qiagen, Hilden, Germany) and purified from residues of genomic DNA by an on-column digestion with the RNase-free DNase set according to the instruction of the manufacturer (Qiagen, Hilden, Germany). Human pancreas total RNA was purchased from Clontech Laboratories (Palo Alto, CA, USA). cDNA synthesis was performed using the First-Strand cDNA synthesis kit of Amersham Biosciences (Freiburg, Germany) appropriate to manufacturer's instructions using 5 µl of Bulk First-Strand cDNA Reaction Mix and pd(N)6 random hexamers as primer. Quality of cDNA was controlled performing a PCR with human GAPDH primers (forward: 5′-AAG GCT GGG GCT CAT TTG-3′, reverse: 5′-AGG CAT TGC TGA TGA TCT TG-3′).
Real-time quantitative PCR
Real-time quantitative PCR analysis was performed using the Applied Biosystems 7300 Real-Time PCR System (Applied Biosystems, Foster City, CA, USA). The PCR mix (25 µl) was composed of 12.5 µl SYBR® Green PCR Master Mix (Applied Biosystems, Foster City, CA, USA), a cDNA amount corresponding to 25 ng RNA used for cDNA synthesis (each sample in a triplicate), and a final concentration of 100 nM from each primer. The following primers were used: human ribosomal protein L13a (RPL13A) as control: forward: 5′-AAG TTT GCC TAT CTG GGG C-3′, reverse: 5′-TAG CCT CAT GAG CTG TTT CTT C-3′; GLUT11-A: forward: 5′-CGG TTC TCC TAC CCG CAT T-3′, reverse: 5′-ATC CTG CCC TGA ATC AGT CTT C-3′; GLUT11-B: forward: 5′-TGA CCT TGG ACC AGA GCT C-3′, reverse: 5′-CTA AGC CCC AAG CTC ATC TC-3′; GLUT11-C: forward: 5′-GCT CAC CGC TTG CTA ATG-3′, reverse: 5′-CTG AAT CAT TCT AGA TCG CAG G-3′.
Cell culture
COS-7 cells were grown in Dulbecco's modified Eagle's medium containing 10% FCS, HEK-293 cells were grown in DMEM/HAM's F-12 medium with 10% FCS.
Promoter analysis
DNA fragments of the 5′-flanking region of exon 1A, exon 1B and exon 1C of SLC2A11 were generated by PCR (Primer: A1 forward: 5′-TGA CGG TAC CGA CCT TCC CTC CAC TAT TGT CC-3′, A1 reverse: 5′-TGA CAA GCT TGA TCA TAT GGG TAT TCT CC-3′; A2 forward: 5′-TGA CGG TAC CCC TGC CTC AGT CTC CCA AAG TGC-3′, A2 reverse: 5′-TGA CAA GCT TGG AGA GGG AGA GCA GGT TAA TT-3′; B forward: 5′-TGA CGG TAC CAC CAT TAG AAA GTT TAT TGA TG-3′, B reverse: 5′-TGA CAA GCT TGC AAC CCT GCG CGA TTC CCT GC-3′; C forward: 5′-TGA CGG TAC CCA GGT GAG CTC CCA GCG G-3′, C1 forward: 5′-TGA CGG TAC CGG CGA CTC CCC CCC AA-3′, C2 forward: 5′-TGA CGG TAC CAC ACG CTG GGG CGA A-3′, C3 forward: 5′-TGA CGG TAC CTT CTT AAA AAC GCT GAG T-3′, C4 forward: 5′-TGA CGG TAC CAG TCT CAG GCC TTA GTC-3′, C5 forward: 5′-TGA CGG TAC CTC TCA ATA CCC ACT-3′, C6 forward: 5′-TGA CGG TAC CTT GCT CCT CTC AAA CGC TCA-3′, C reverse: 5′-TGA CAA GCT TCC ATT AGC AAG CGG TGA G-3′). The BAC clone KB1125A3 Citation[8] was used as template. Constructs were fused to luciferase cDNA in the vector pGL3-basic (Promega, Madison, WI, USA). These constructs were transiently transfected into COS-7 or HEK-293 cells with the aid of FuGene 6®-reagent (Roche, Mannheim, Germany). 48 h after transfection, cells were lysed, and luciferase activity was analysed with the luciferase assay system from Promega (Madison, WI, USA) according to the instruction of the manufacturer.
Expression of GLUT11-A-C in COS-7 cells
The cDNAs of GLUT11-A-C were subcloned into the mammalian expression vector pCMV, which harbors a simian virus 40 origin and a cytomegalovirus (CMV) promoter. Transfection was performed with calcium phosphate/DNA co-precipitates, and membrane fractions were isolated as described previously Citation[11].
Reconstitution of glucose transport activity from membrane fractions
GLUT proteins were solubilized and reconstituted into lecithin liposomes as described previously Citation[6].
Expression of GLUT11-A-C in Xenopus laevis oocytes
Plasmids containing the cDNAs of GLUT11-A-C were linearized with Nhe I and in vitro transcribed with T7 polymerase mMESSAGE mMACHINE™ (Ambion, Austin, TX, USA). Adult female Xenopus laevis were obtained from Nasco (Fort Atkinson, WI, USA) and housed at 18°C on the 12 h light/dark cycle. Stage V/VI oocytes were harvested from anesthetized frogs and placed in Modified Barth's Media (MBM) (88 mM NaCl, 1 mM KCl, 0.33 mM Ca(NO3)2, 0.41 mM CaCl2, 0.82 mM MgSO4, 2.4 mM NaHCO3, 2.5 mM Na pyruvate, 0.1 mg/ml penicillin, 0.05 mg/ml gentamycin sulphate, 10 mM Hepes at pH 7.5). The follicular layer was removed by treatment for 2 h with 0.02 g/ml type I collagenase (Sigma, St Louis, Missouri, USA). After selection, hypertonic phosphate treatment was applied to all oocytes subjected to microinjection. Prior to mRNA microinjection the oocytes were incubated in MBM at 16–18°C for 24 h to restore their osmolarity. The oocytes were injected with 10–50 nl (∼20 ng) GLUT11 synthetic mRNA transcript and incubated for 3–5 days at 16–18°C prior to functional uptake assays.
Radiotracer flux assay
The influx experiments were performed at 20°C using 5–10 oocytes for each condition and 14C or 3H labelled hexose at a specific activity of 4 µCi/ml. Oocytes were washed with ice cold MBM to stop the incubation and then individual oocytes were placed in vials and dissolved in 0.5 ml 5% SDS for 30 min. Finally, scintillation fluid (5 ml) was added to each vial and radio activity measured using a Beckman LS6500. All experiments were performed 3–7 times and the results were compared to the influx values obtained with water-injected oocytes.
Immunocytochemical detection of GLUT11-A-C overexpressed in COS-7 cells
COS-7 cells were grown on 12 mm glass coverslips and transfected with 0.5 µg GLUT11-A-C cDNA with FuGene®6-Reagent (Roche, Mannheim, Germany). After 48 h, the cells were fixed with acetone, and washed three times with PBS containing 1% BSA. Cells were permeabilized with 0.5% Triton X-100 for 20 min. Then cells were washed three times with PBS/1% BSA and blocked in PBS containing 10% normal goat serum for 1 h and incubated with the preimmuneserum, the anti-GLUT11 antiserum, or the blocked anti-GLUT11 serum (1:2000). Thereafter, cells were washed three times with PBS/1% BSA and incubated for 45 min with FITC-conjugated anti-rabbit antibody (1:200; Dianova, Hamburg, Germany). In one set of experiments we costained the nuclei by incubation of the cells with TO-PRO-3 (Molecular Probes) in a dilution of 1:1000 for 10 min. Cells were mounted on coverslips with Flouromount (Biozol, Eching, Germany) and fluorescence was detected with a confocal laser scanning microscope (Leica TCS NT; Leica Micosystems, Heidelberg, Germay). FITC was excited at 488 nm using an agron/krypton laser. The FITC fluorescence was selected by a 530 nm band-pass filter. Each optical section was averaged four times.
Results
Alternative exons 1 in the SLC2A11 gene generate three different N-termini of GLUT11
A database search performed with the sequence of the cDNA of GLUT11 (GenBank accession no. AJ271290) identified three clusters of ESTs which differ only in their 5′-sequence from the sequence of the known GLUT11. In order to prove that three GLUT11 transcripts arise we performed 5′-RACE-PCR on human RNAs. Sequence analysis of the 5′-RACE-PCR products generated with primers corresponding to the 5′-adapter and to a sequence of exon 3 revealed that in human skeletal muscle mRNAs of GLUT11-A-C exist in comparable amounts whereas in pancreas the mRNA of GLUT11-C is the most abundant isoform (see ). Alignment of the RACE-products with the genomic sequence indicated that three exons 1 of SLC2A11 (exon 1A, exon 1B, exon 1C) exist which are all spliced to the same splice acceptor site at exon 2. The alternative splicing results in three isoforms (GLUT11-A, -B, and -C) which differ only in their N-terminal sequence (see ). As shown in one splicing variant was identified in which exon 1A is spliced to a part of exon 1B and then to exon 2. But since an in-frame stop codon is located upstream of the initiator methionine ATG codon in exon 1B the translation of this mRNA also generates GLUT11-B.
Figure 1. Diagrams of the SLC2A11 gene structure and organization. Exons 1A, 1B, or 1C are spliced to the same splice acceptor site in exon 2 and generate four mRNAs encoding for GLUT11-A-C which differ in their N-terminal sequence. Positions of start (ATG) und stop (*) codons are indicated. Acc. numbers of ESTs encoding for the different isoforms are listed below each diagram.
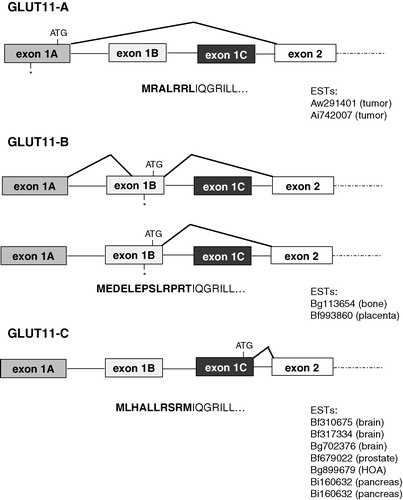
Table I. Tissue specific expression of GLUT11-A-C. (a) Number of 5′-RACE-PCR clones in human skeletal muscle and pancreas with a sequence corresponding to exon 1A, 1B, or 1C. (b) cDNA levels of GLUT11-A, -B and -C in pancreas and adipose tissue. Quantification of cDNA levels of GLUT11-A-C and RPL13A (control) were performed by real-time quantitative PCR. Amplification reactions were performed in triplicates. Results are presented as relative cDNA levels expressed as a percentage and depict a representative experiment that was repeated three times.
Tissue specific expression of GLUT11-A-C
The RACE-PCR experiments suggested a tissue specificity of the expression of GLUT11-A-C. In order to analyse the expression pattern of GLUT11-A-C in more detail, specific probes corresponding to the sequence of exon 1A, 1B, and 1C of SLC2A11 were used for Northern blot analysis. A probe specific for exon 1A gave the strongest signal in kidney, and weaker signals in heart, placenta, and skeletal muscle (see ). The isoform GLUT11-B appears to be expressed mainly in placenta, and to a lower extent in kidney (). GLUT11-C mRNA was detected in pancreas and also in heart and muscle (). The predominant expression of GLUT11-C in pancreas was confirmed in 5′-RACE-PCR experiments, where 26 of 27 sequenced clones corresponded to the sequence of exon 1C, and only one to that of exon 1B (). In addition, real-time quantitative PCR experiments showed that the cDNA level of GLUT11-C in pancreas was more than 30 times higher than that of GLUT11-B, whereas GLUT11-A mRNA was almost undetectable (see ). Similarly, GLUT11-C was the predominant isoform in adipose tissue, whereas GLUT11-B exhibited a moderate and GLUT11-A nearly no expression ().
Figure 2. Northern blot analysis of the tissue distribution of GLUT11-A, -B, and -C. Northern blots with mRNA from the indicated human tissues were hybridized with (a) a GLUT11 cDNA probe specific for exon 1A (50811–51032 bp; genomic clone AP000350), (b) a GLUT11 cDNA probe specific for exon 1B (51340–51574 bp), and (c) a GLUT11 cDNA probe specific for exon 1C (51914–52073 bp).
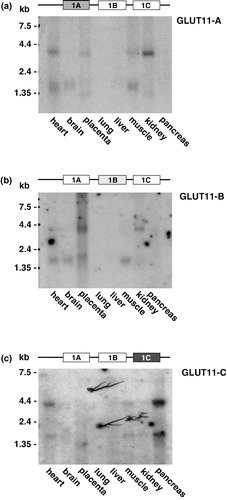
We have previously described a GLUT11 mRNA with a size of 7.0 kb that was exclusively detected in heart and skeletal muscle Citation[6]. However, with specific probes for exons 1A-C we detected a smaller signal with a size of 3.8 kb not only in heart and skeletal muscle but also in placenta, kidney, and pancreas. A BLAST search performed with the GLUT11 sequence (GenBank accession no. AJ271290) and sequence analysis indicated that the full length cDNA probe which we had used in our first characterization Citation[6] contains a small stretch of 25 bp, that is also present in the sequence of α-myosin heavy chain (bp 822–847 in GLUT11 cDNA; AJ271290). We generated two cDNA fragments of GLUT11 lacking the α-myosin heavy chain (α-MHC) motif (bp 1–624 and bp 952–1608) and used a mixture of both fragments as probe for Northern blot analysis. With this probe, we detected the same signal of 3.8 kb as with the exon 1-specific probes in heart, skeletal muscle, placenta, kidney, and pancreas (see ). In addition, we incubated a Northern blot with the mRNA of heart with a probe of the DNA fragment 626–951 bp containing the α-MHC motif and detected a signal of 7.0 kb (). The same signal with a size of 7.0 kb appeared when we used an EST of α-MCH (GenBank accession no. AA445445) as a probe (see ). These results indicate that the signal detected with the full length GLUT11 cDNA corresponds to α-MHC mRNA rather than GLUT11. In addition to the 3.8 kb GLUT11 mRNA we observed a second signal with a size of approximately 1.5 kb (see and ) which we assume to be an aberrant splicing product that cannot encode for a functional glucose transporter with a size of 40–45 kDa. The same signal was detected in Northern blots of different human cancer cell lines whereas no 3.8 kb GLUT11 transcript was visible (data not shown). This observation is consistent with the fact that in cancer cells splicosomal errors often result in the production of aberrant transcripts Citation[12], Citation[13].
Figure 3. Specific expression of GLUT11 in human tissues. (a) Northern blots with mRNA from the indicated human tissues were hybridized as described with a cDNA probe of human GLUT11 (bp 1–624 and bp 952–1608; GenBank accession no. AJ271290). (b) Northern blot with the RNA of mouse heart was hybridized with a probe of the GLUT11 cDNA fragment 626–951 containing the α-MHC motif. (c) Northern blot with the RNA of mouse heart was hybridized with a probe corresponding to an EST of α-MCH (GenBank accession no. AA445445).
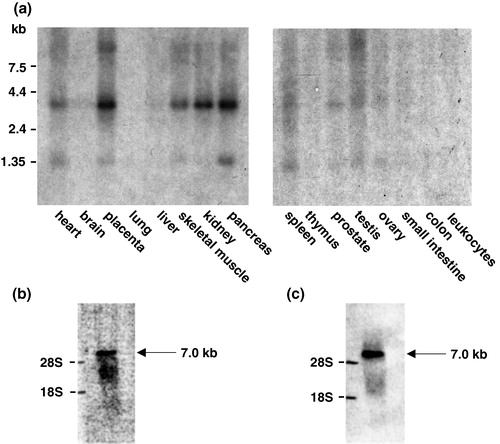
Characterization of the transcriptional control of SLC2A11
In order to determine whether any of the 5′-flanking sequences of exon 1A-C confer basal promoter activity, reporter assays were performed. To narrow down the putative promoter regions, the 5′-flanking sequences of human GLUT11 exon 1A-C were analysed by alignment with the corresponding genomic sequence of the chimpanzee ortholog on chromosome 23. While the complete regions between exon 1A and exon 1B and between exon 1B and exon 1C were highly conserved, the 5′-flanking region of exon 1A exhibited two conserved regions disrupted by a non-homologous transposon-like sequence appearing in several non-coding regions of the human genome (see ). Therefore, four putative promoter fragments designated A1, A2, B, and C () were cloned in front of the luciferase reporter gene. These constructs were transiently transfected into COS-7 cells and luciferase activity was assayed. As shown in the highest luciferase activity was detected for the 5′-flanking region of exon 1C (bp −326 to bp +92), whereas significant but much lower promoter activity was detected with the fragments flanking exon 1A and 1B. The same results were observed after transfection of HEK-293 cells with the promoter constructs (data not shown). In order to test the specificity of the 5′-flanking regions of exon 1A and 1B as promoters their sequence was fused to the luciferase gene in 3′–5′ orientation. As shown in inverted promoters A and B lacked luciferase activity indicating that the sequences upstream of each first exon exhibit promoter activity. The inverted sequence of fragment C showed only 50% of the promoter activity detected with the 5′–3′-orientated sequence ().
Figure 4. Analysis of different SLC2A11 promoters. (a) Comparison of the 5′-flanking regions of human SLC2A11 exon 1A, 1B, and 1C with the corresponding genomic sequence of the chimpanzee ortholog on chromosome 23. Homologous regions are marked as black lines and fragments used for luciferase assays are shown as arrows. The numbers refer to the positions in the gene, where +1 is the transcription initiation site of exon 1C, −680 that of exon 1B, and −1425 that of exon 1A. (b) Analysis of putative GLUT11-A-C promoter regions in 5′–3′ as well as in 3′–5′ orientation (indicated by *). Constructs of the 5′-flanking regions of exons 1A-C were generated by PCR and fused in both directions to the luciferase cDNA in the pGL3-basic vector by blunt-end ligation. COS-7 cells were transfected with these promoter-reporter constructs and luciferase activities were measured 48 h after transfection. Results represent means±SD of triplicate samples (transfections) from a representative experiment that was repeated three times. (c) Deletion analysis of the GLUT11-C promoter. Constructs of the 5′-flanking region of exon 1C were generated by PCR and fused to the luciferase cDNA in the pGL3-basic vector. COS-7 cells were transfected with these promoter-reporter constructs and luciferase activities were measured 48 h after transfection. Results represent means±SD of triplicate samples (transfections) from a representative experiment that was repeated three times.
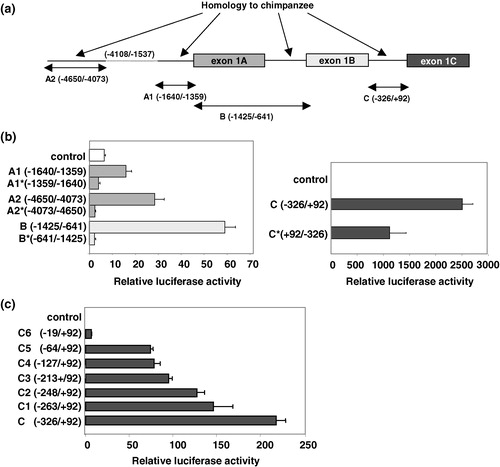
In order to further characterize promoter C we performed a deletion analysis of the 5′-flanking region of exon 1C which contains several putative motifs for ubiquitously expressed transcription factors. As shown in sequential deletion of fragment C resulted in a progressive decrease of promoter activity. Thus, the region between bp −326 and the transcription start of exon 1C harbours critical promoter elements. The luciferase activity of the C-promoter (bp −326/ + 92) was considerably lower in this set of experiments than of that shown in due to a lower transfection efficiency. However, the relative increase induced by the C-promoter is comparable in each experiment, 1142-fold in the experiment shown in and 1185-fold in the experiment shown in .
Reconstituted glucose transport activity of GLUT11-A-C
For the characterization of their glucose transport activity, the cDNAs of GLUT11-A-C were subcloned into the expression vector pCMV and were transiently expressed in COS-7 cells. Plasma membranes were solubilized and proteins reconstituted into lecithin liposomes in order to measure glucose transport activity. As illustrated in , no difference in glucose uptake was detected for the three GLUT11 isoforms. Transfection of COS-7 cells with GLUT11-A-C increased D-glucose transport activity about 2-fold as compared to COS-7 cells transfected with the empty vector. This result suggests that different N-termini do not alter the glucose transport activity.
Figure 5. Sugar transport activity and substrate specificity of GLUT11-A-C. (a) Reconstituted glucose transport activity of GLUT11-A-C. COS-7 cells were transfected with cDNA of GLUT11-A, -B, and -C and membranes from COS-7 cells expressing the GLUT11 isoforms were reconstituted into lecithin liposomes and glucose transport activity was assayed at a D-glucose concentration of 5 mM. Data represent means±S.E. from a representative experiment. Co, control, membranes of cells transfected with the blank vector. (b) Substrate specificity of GLUT11-A-C detected in the Xenopus oocyte system. Xenopus oocytes were injected with the cRNA of GLUT11-A, -B, or -C or water 5 days before the uptake of radiolabeled substrates (100 µM) was measured. Filled bars represent total uptake of the indicated sugars, the open bars uptake detected for water-injected oocytes and the hatched bars represent the net uptake.
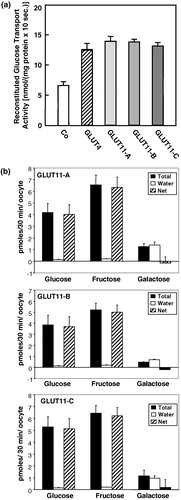
Glucose transport activity of GLUT11-A-C expressed in Xenopus laevis oocytes
We have previously shown that the reconstituted glucose transport activity could be inhibited by fructose indicating that GLUT11 is a bi- or multifunctional transporter Citation[6]. In order to test the ability of GLUT11-A-C to transport fructose and galactose their cDNAs were cloned into the enhanced Xenopus expression vector pGEM-HE, were in vitro transcribed and the RNAs were injected into Xenopus oocytes. shows the uptake of glucose, fructose, and galactose into RNA injected oocytes in comparison to the water-injected controls. All isoforms transported both glucose and fructose but not galactose.
Subcellular distribution of GLUT11-A-C
In order to determine the subcellular localization of GLUT11-A-C COS-7 cells were transfected with the cDNA of GLUT11-A-C. After 48 h cells were fixed and stained for GLUT11 with an antibody raised against the C-terminus of GLUT11. As shown in the anti-GLUT11 antibody detects GLUT11-A specifically, while no signals were detected in untransfected cells or in cells stained with the preimmuneserum or with the blocked serum. The same specificity was obtained for GLUT11-B and -C (data not shown). demonstrates that the three isoforms do not differ in their subcellular localization detected by laser scanning microscopy. Immunoreactive proteins were localized at the cell surface and in an intracellular compartment (). Their subcellular distribution was independent of the presence of serum, suggesting that the three GLUT11 isoforms are constitutively located in the plasma membrane.
Figure 6. Subcellular distribution of GLUT11-A, -B, and -C overexpressed in COS-7 cells. (a) COS-7 cells were transfected with GLUT11-A and stained with preimmuneserum, with antiserum against a C-terminal peptide of GLUT11 (anti-GLUT11 antiserum), or the blocked antiserum and nuclei were co-stained with TO-PRO3. (b) COS-7 cells transfected with GLUT11-A-C were incubated in a serum free medium (upper panel) or in the presence of 10% FCS (lower panel) and stained with the anti-GLUT11 antiserum. In all experiments GLUT11 was detected with a FITC-labelled secondary antibody and visualized by confocal laser scanning microscopy. Thisfigure is reproduced in colour in Molecular Membrane Biology online.
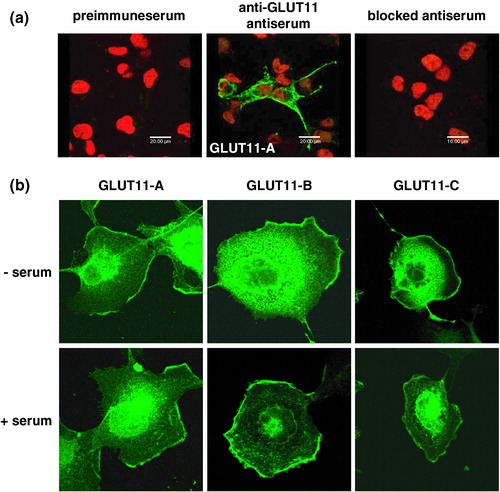
Lack of an orthologue of SLC2A11 in rodents
In spite of considerable effort we failed to clone a mouse cDNA of GLUT11 or detect an mRNA in Northern blots with the human probe. In addition, a murine orthologue was missing in the genomic sequence syntenic to human chromosome 22q11.23 (see ). Thus, in order to prove the absence of SLC2A11 in the mouse genome, we hybridized genomic DNA of different species (‘zoo’ blot) with the human GLUT11 probe. The probe hybridiZed with fragments of human, dog, cow, pig, and rabbit DNA but not with mouse, rat, chicken, frog, and fish DNA, suggesting that the SLC2A11 gene is present in the genome of most mammals except in rodents (see ).
Figure 7. Conservation of SLC2A11 orthologs across vertebrates. (a) Genomic localization of SLC2A11 on human chromosome 22q11.23 and comparison of this region with the syntenic region of mouse chromosome 10. SMARCB1 encodes for integrase interactor 1 protein, DERL3 encodes for Derl-like domain family member 3, and MIF encodes for macrophage migration inhibitory factor. (b) A multiple-species Southern ‘zoo’ blot containing 10 µg of EcoR I-digested genomic DNA samples from representative species from class mammals (human, mouse, rat, dog, cow, and pig), bird (domestic chick, Gallus domesticus), frog (Xenopuslaevis), and from fish (catfish, Parasilurus asolus) was hybridized with a cDNA probe of human GLUT11 (bp 1–624 and bp 952–1608). Ethidium bromide stained gel used for Southern blotting is shown in the lower panel.
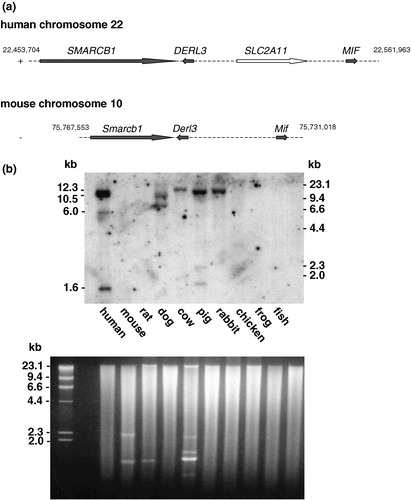
Discussion
Three GLUT11 isoforms (GLUT11-A-C) are generated by alternative promoter usage and splicing. GLUT11-A-C which differ only in their N-terminal sequence are expressed in a tissue specific manner. Northern blot analysis with specific probes corresponding to the sequences of the three exons 1 and quantitative real-time PCR experiments have demonstrated that GLUT11-A is present in heart, skeletal muscle, and kidney, GLUT11-B in placenta, adipose tissue, and kidney, and GLUT11-C in adipose tissue, heart, skeletal muscle, and pancreas (see and ). The SLC2A11 gene is the third gene within the GLUT encoding gene family which generates different isoforms by splicing and in each case the genes generate proteins which differ in their N-termini. Alternative splicing of the SLC2A9 gene results in two isoforms which are also expressed in tissue specific manner and differ in their membrane targeting Citation[14]. In addition, two alternative exons 1 of the SLC2A14 gene encode for two different GLUT14 isoforms which are both exclusively expressed in testis Citation[2].
Analysis of the 5′-flanking regions of exon 1A, 1B, and 1C for promoter activity indicated that the tissue specific expression of GLUT11-A-C is regulated by three different promoters: (1) promoter A is located in the 5′-flanking region of exon 1A and regulates the expression of GLUT11-A and the isoform of GLUT11-B containing exon 1A in its 5′-UTR (see ), (2) promoter B contains exon 1A and the region between exon 1A and exon 1B and regulates the expression of the GLUT11-B isoform not including the sequence of exon 1A in its 5′-UTR, and (3) promoter C is located between exon 1B and exon 1C and regulates the expression of GLUT11-C. Since the activity of promoter A and B was very low compared to the activity of promoter C we tested their specificity and inverted the associated sequences. Indeed, promoter activity only appeared when the promoter sequences were in the right (5′–3′) orientation. Moreover, we tested an additional construct containing fragment A1 fused to fragment A2 but obtained only an additive but no synergistic effect for the fusion product (data not shown). One reason for a low promoter activity could be the absence of transcription factors regulating the expression of GLUT11-A and -B in the cell lines used for promoter studies. Analysis of the 5′-flanking sequence of exon 1A and 1B by the programme MatInspector (www.genomatix.de) revealed putative binding sites for muscle specific transcription factors such as MEF2, MEF3, and TEF. Myocyte enhancer factor 2 (MEF2) is involved in the activation of muscle gene expression Citation[15], Citation[16]. MEF3 motifs are found in many skeletal muscle specific regulatory regions and have been shown to be involved in the transcriptional regulation of the cardiac troponin C gene Citation[17] and the aldolase A muscle-specific promoter Citation[18], Citation[19]. MEF3 has also been shown to bind to and activate the promoter of myogenin which is required for myoblast fusion Citation[20]. Recently, we have described the skeletal muscle and heart specific expression of GLUT11 that was analysed by an immunohistochemical approach with an antibody against the C-terminal peptide of GLUT11 Citation[7]. Together with the results shown in this study it can be speculated that GLUT11-A and/or GLUT11-C are the common isoforms expressed in heart and muscle and that their expression is regulated by muscle specific transcription factors. Interestingly, GLUT4 is also expressed in skeletal muscle and in heart, as well as in adipocytes Citation[21], Citation[22] and a conserved MEF2 site essential for the function of muscle specific enhancer has been mapped to the 5′-flanking region of the SLC2A4 (GLUT4 encoding gene) promoter Citation[23], Citation[24].
Northern blot analysis and real-time PCR experiments also demonstrated that most tissues express two GLUT11 isoforms, heart and skeletal muscle express GLUT11-A and -C, adipose tissue expresses GLUT11-B and -C, and kidney and placenta express GLUT11-A and -B. However, pancreas is the only tissue expressing almost exclusively one isoform, GLUT11-C, a result that was also confirmed by 5′-RACE-PCR (see and ). The selective expression of GLUT11-C in pancreas suggests that the transcription factors regulating expression of GLUT11-A and -B are absent or barely activated in pancreas.
In contrast to promoter A and B, promoter C (5′-flanking region of exon 1C) exhibits high promoter activity in all tested cell lines. This sequence contains putative motifs for ubiquitously expressed transcription factors (two RREB1 and one E2F3 motifs) which are described to be involved in the regulation of proliferation, and could be responsible for the high promoter activity in all tested cell lines. Ras responsive element binding protein 1 (RREB1) is a zinc finger protein that is involved in the differentiation response to Ras and known to be expressed ubiquitously in human tissues Citation[25]. E2F transcription factor 3 (E2F3) has been described to induce both proliferation and apoptosis Citation[26]. Humbert et al. Citation[27] demonstrated that E2F3 is critical for the transcriptional activation of genes that control the rate of proliferation of both primary and tumor cells. The authors identified a number of genes, including B-myb, cyclin A, cdc2, cdc6, and DHFR, whose expression is dependent on the presence of E2F3.
The specific physiological function of the three GLUT11 variants is unknown. We did not detect any difference in the glucose transport activity or in the subcellular localization of GLUT11-A-C. However, it cannot be excluded that the subcellular distribution of GLUT11-A-C detected after overexpression in COS-7 cells may differ from the distribution of the variants in native tissues. Wu et al. Citation[9] described two GLUT11 variants which were designated GLUT11-S and GLUT11-L. GLUT11-S corresponds to GLUT11-A, and GLUT11-L corresponds to GLUT11-B. Overexpression of GLUT11-L in HEK-293 cells also showed transport activity after reconstitution into lecithin liposomes and a predominant localization of the protein in the plasma membrane Citation[9]. Here we demonstrate that all three isoforms transport both glucose and fructose but not galactose indicating that they do not differ in their substrate specificity (see ).
Another main finding in this study is that the gene encoding for GLUT11 is absent in the genome of rodents as for instance ∼99% of human genes possess an ortholog in the mouse genome. Because human beings are phylogenetically closer related to mouse than to pig or dog Citation[28] it is implausible that SCL2A11 emerged after the rodent/primate common ancestor (85 million years ago). It is more reasonable that SLC2A11 was deleted in the genome of rodents and that the function mediated by GLUT11 is not important in rodents or more likely, compensated by another member of the GLUT family. SLC2A11 is not the only glucose transporter encoding gene which is not expressed in rodents. Wu and Freeze Citation[2] described the identification and cloning of SLC2A14, a close relative of SLC2A3 which arose by duplication of the GLUT3 encoding gene. It was described that no ortholog of GLUT14 was found in the mouse genome. However, all other GLUT encoding genes are present in mouse and rat genomes.
What are the specific functions of GLUT11-A-C in human? One speculation is that GLUT11 takes over the function of the lacking GLUT2 in pancreas of Fanconi-Bickel syndrome patients. Fanconi-Bickel syndrome (FBS) patients show characteristic clinical features such as hepatorenal glycogen accumulation, glucose and galactose intolerance, fasting hypoglycemia, and tubular nephropathy. Several mutations of the SLC2A2 gene (missense, nonsense, frameshift, and splice-site mutations) have been identified Citation[29], Citation[30], leading to a lack of GLUT2. Since an insulin treatment is not essential for FBS patients it can be speculated that GLUT11 triggers the insulin secretion which is normally regulated by GLUT2. The pathophysiology of this syndrome differs markedly from the phenotype of the GLUT2 knockout mouse Citation[31]. Mice lacking GLUT2 in β-cells are hyperglycaemic, hypoinsulinaemic, and hyperglucagonaemic and die within the first three weeks of life. Their endocrine pancreas shows a loss of first phase glucose-stimulated insulin secretion Citation[32], Citation[33]. We assume that the pathophysiology of FBS patients differs markedly from the phenotype of GLUT2 null mutants because GLUT11 is absent in mice and cannot compensate for the lacking GLUT2. This paper was first published online on prEview on 19 July 2005.
The authors thank Dr Michael Gaster for kind help with fat tissue preparation. The skillful technical assistance of Brigitte Rischke is gratefully acknowledged. This work was supported by a grant from the Deutsche Forschungsgemeinschaft (Schu750/4–1 and 4–2).
References
- Joost H-G, Thorens B. The extended GLUT-family of sugar/polyol transport facilitators: Nomenclature, sequence characteristics, and potential function of its novel members. Mol Membr Biol 2001; 18: 247–256
- Wu X, Freeze HH. GLUT14, a duplicon of GLUT3, is specifically expressed in testis as alternative splice forms. Genomics 2002; 80: 553–557
- Miyamoto K, Hase K, Takagi T, Fujii T, Taketani Y, Minami H, Oka T, Nakabou Y. Differential responses of intestinal glucose transporter mRNA transcripts to levels of dietary sugars. Biochem J 1993; 295: 211–215
- Uldry M, Ibberson M, Horisberger JD, Chatton JY, Riederer BM, Thorens B. Identification of a mammalian H(+)-myo-inositol symporter expressed predominantly in the brain. EMBO J 2001; 20: 4467–4477
- Scheepers A, Doege H, Joost H-G, Schürmann A. Mouse GLUT8: Genomic organization and regulation of expression in 3T3-L1 adipocytes by glucose. Biochem Biophys Res Commun 2001; 288: 969–974
- Doege H, Bocianski A, Scheepers A, Axer H, Eckel J, Joost H-G, Schürmann A. Characterization of human glucose transporter (GLUT) 11 (encoded by SLC2A11), a novel sugar-transport facilitator specifically expressed in heart and skeletal muscle. Biochem J 2001; 359: 443–449
- Gaster M, Handberg A, Schürmann A, Joost H-G, Beck-Nielsen H, Schoder HD. GLUT11 but not GLUT8 and GLUT12, is expressed in human skeletal muscle in a fiber type-specific pattern. Pflugers Arch 2004; 448: 105–113
- Sasaki T, Minoshima S, Shiohama A, Shintani A, Shimizu A, Asakawa S, Kawasaki K, Shimizu N. Molecular cloning of a member of the facilitative glucose transporter gene family GLUT11 (SLC2A11) and identification of transcription variants. Biochem Biophys Res Commun 2001; 289: 1218–1224
- Wu X, Li W, Sharma V, Godzik A, Freeze HH. Cloning and characterization of glucose transporter 11, a novel sugar transporter that is alternatively spliced in various tissues. Mol Genet Metab 2002; 76: 37–45
- Chenchik A, Siebert P. Studying tissue-specific gene expression with Marathon-Ready cDNAs. CLONTECHniques 1996; XI: 22
- Schurmann A, Monden I, Joost H-G, Keller K. Subcellular distribution and activity of glucose transporter isoforms GLUT1 and GLUT4 transiently expressed in COS-7 cells. Biochim Biophys Acta 1992; 1131: 245–252
- Venables JP. Aberrant and alternative splicing in cancer. Cancer Res 2004; 64: 7647–7654
- Kalnina Z, Zayakin P, Silina K, Line A. Alterations of pre-mRNA splicing in cancer. Genes Chromosomes Cancer 2005; 42: 342–357
- Augustin A, Carayannopoulos MO, Dowd LO, Phay JE, Moley JF, Moley KH. Identification and characterization of human glucose transporter-like protein-9 (GLUT9): Alternative splicing alters trafficking. J Biol Chem 2004; 279: 16229–16236
- Buckingham M, Bajard L, Chang T, Daubas P, Hadchouel J, Meilhac S, Montarras D, Rocancourt D, Relaix F. The formation of skeletal muscle: from somite to limb. J Anat 2003; 202: 59–68
- Naya FS, Olson E. MEF2: A transcriptional target for signaling pathways controlling skeletal muscle growth and differentiation. Curr Opin Cell Biol 1999; 11: 683–688
- Parmacek MS, Ip HS, Jung F, Shen T, Martin JF, Vora AJ, Olson EN, Leiden JM. A novel myogenic regulatory circuit controls slow/cardiac troponin C gene transcription in skeletal muscle. Mol Cell Biol 1994; 14: 1870–1885
- Hidaka K, Yamamoto I, Arai Y, Mukai T. The MEF-3 motif is required for MEF-2-mediated skeletal muscle-specific induction of the rat aldolase A gene. Mol Cell Biol 1993; 13: 6469–6478
- Salminen M, Lopez S, Maire P, Kahn A, Daegelen D. Fast-muscle-specific DNA-protein interactions occurring in vivo at the human aldolase A M promoter are necessary for correct promoter activity in transgenic mice. Mol Cell Biol 1996; 16: 76–85
- Spitz F, Demignon J, Porteu A, Kahn A, Concordet JP, Daegelen D, Maire P. Expression of myogenin during embryogenesis is controlled by Six/sine oculis homeoproteins through a conserved MEF3 binding site. Proc Natl Acad Sci USA 1998; 95: 14220–14225
- James DE, Strube M, Mueckler M. Molecular cloning and characterization of an insulin regulatable glucose transporter. Nature 1989; 338: 83–87
- Birnbaum MJ. Identification of a novel gene encoding an insulin-responsive glucose transporter protein. Cell 1989; 57: 305–315
- Liu ML, Olson AL, Edgington NP, Moye-Rowley WS, Pessin JE. Myocyte enhancer factor 2 (MEF2) binding site is essential for C2C12 myotube-specific expression of the rat GLUT4/muscle-adipose facilitative glucose transporter gene. J Biol Chem 1994; 269: 28514–28521
- Thai MV, Guruswamy S, Cao KT, Pessin JE, Olson AL. Myocyte enhancer factor 2 (MEF2)-binding site is required for GLUT4 gene expression in transgenic mice. Regulation of MEF2 DNA binding activity in insulin-deficient diabetes. J Biol Chem 1998; 273: 14285–14292
- Thiagalingam A, De Bustros A, Borges M, Jasti R, Compton D, Diamond L, Mabry M, Ball DW, Baylin SB, Nelkin BD. RREB-1, a novel zinc finger protein, is involved in the differentiation response to Ras in human medullary thyroid carcinomas. Mol Cell Biol 1996; 16: 5335–5345
- Ginsberg D. E2F1 pathways to apoptosis. FEBS Lett 2002; 529: 122–125
- Humbert PO, Verona R, Trimarchi JM, Rogers C, Dandapani S, Lees JA. E2f3 is critical for normal cellular proliferation. Genes Dev 2000; 14: 690–703
- Murphy WJ, Eizirik E, O'Brien SJ, Madsen O, Scally M, Douady CJ, Teeling E, Ryder OA, Stanhope MJ, de Jong WW, Springer MS. Resolution of the early placental mammal radiation using baysian phylogenetics. Science 2001; 294: 2348–2351
- Santer R, Groth S, Kinner M, Dombrowski A, Berry GT, Brodehl J, Leonard JV, Moses S, Norgren S, Skovby F, Schneppenheim R, Steinmann B, Schaub J. The mutation spectrum of the facilitative glucose transporter gene SLC2A2 (GLUT2) in patients with Fanconi-Bickel syndrome. Hum Genet 2002; 110: 21–29
- Santer R, Steinmann B, Schaub J. Fanconi-Bickel syndrome – a congenital defect of facilitative glucose transport. Curr Mol Med 2002; 2: 213–227
- Thorens B, Guillam MT, Beermann F, Burcelin R, Jaquet M. Transgenic reexpression of GLUT1 or GLUT2 in pancreatic beta cells rescues GLUT2-null mice from early death and restores normal glucose-stimulated insulin secretion. J Biol Chem 2000; 275: 23751–23758
- Guillam MT, Dupraz P, Thorens B. Glucose uptake, utilization, and signaling in GLUT2-null islets. Diabetes 2000; 49: 1485–1491
- Thorens B. GLUT2 in pancreatic and extra-pancreatic gluco-detection. Mol Membr Biol 2001; 18: 265–273