Abstract
Cyt2Aa1 is a haemolytic membrane pore forming toxin produced by Bacillus thuringiensis subsp. kyushuensis. To investigate membrane pore formation by this toxin, second-site revertants of an inactive mutant toxin Cyt2Aa1-I150A were generated by random mutagenesis using error-prone PCR. The decrease in side chain length caused by the replacement of isoleucine by alanine at position 150 in the αD-β4 loop results in the loss of important van der Waals contacts that exist in the native protein between I150 and K199 and L203 on αE. 28 independent revertants of I150A were obtained and their relative toxicity can be explained by the position of the residue in the structure and the effect of the mutation on side-chain interactions. Analysis of these revertants revealed that residues on αA, αB, αC, αD and the loops between αA and αB, αD and β5, β6 and β7 are important in pore formation. These residues are on the surface of the molecule suggesting that they may participate in membrane binding and toxin oligomerization. Changing the properties of the amino acid side-chains of these residues could affect the conformational changes required to transform the water-soluble toxin into the membrane insertion competent state.
Introduction
Bacillus thuringiensis (Bt) insecticidal protein toxins are divided into two families (Cry and Cyt) with unrelated amino acid sequences Citation[1], Citation[2]. Although the X-ray crystallographic structures of Cry and Cyt toxins are entirely different Citation[3], Citation[4], the mechanism of action of these two families is thought to be the same. Both Cry and Cyt toxins form small pores approximately 1–2 nm in diameter in the larval midgut epithelial cell membranes leading to colloid-osmotic lysis Citation[5]. Cyt2Aa1 which is the subject of this study is produced as a 29 kDa protoxin which is processed at both ends by gut proteases to generate the active 21–23 kDa toxin Citation[6].
Cyt2Aa1 exists as an inactive protoxin dimer linked by intertwined N-terminal strands in a continuous 12-stranded β-sheet Citation[4]. The high sequence similarity between members of the Cyt toxin family (see ) indicates that they have the same structural fold as Cyt2Aa1 Citation[4].
Figure 1. Multiple sequence alignment of amino acids from Cyt δ-endotoxins. The alignment was performed using Clustal X. The number on the right of each sequence indicates the residue number at the end of each line. The symbols (*), (:) and (.) represent identical, highly conserved and less conserved amino acids, respectively. The graph under the sequences indicates the degree of amino acid identity for all toxins (from 0% at the bottom to 100% at the top). The secondary structures of Cyt2Aa1 are shown on top of the sequence. The ruler number under the sequences is shown for indication of the alignment position only. The residues in Cyt2Aa1 which were selected for mutagenesis are marked by |. This Figure is reproduced in colour in Molecular Membrane Biology online.
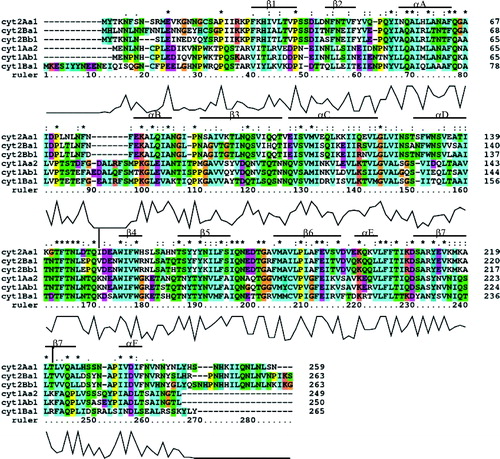
Protease processing by insect gut enzymes cleaves the intertwined N-terminal β1 strands responsible for dimerization to release the active toxin as a monomer and simultaneously eliminate several structural barriers to pore formation Citation[4], Citation[6]. Activated toxin molecules can form pores in cell membranes Citation[7], phospholipid vesicles and bilayers Citation[8–10]. Lytic pore formation is believed to require toxin monomers to assemble into an oligomeric complex Citation[4], Citation[11], Citation[12].
The Cyt toxin pore is not likely to be formed by the six α-helices in the structure, as they are too short to span the 30 Å width of the lipid bilayer Citation[4]. In contrast, strands β5, β6 and β7 are sufficiently long to span the bilayer. Over these three strands the sheet shows an amphiphilic or hydrophobic character and therefore they could oligomerise to form a β-barrel pore Citation[4].
The aim of this study was to investigate structure-function relationships associated with membrane pore formation by Cyt2Aa1. A non-haemolytic mutant of Cyt2Aa1 was generated and used as a template for reversion mutagenesis. Analysis of the revertant proteins should provide information about residues that are important in pore formation. This method proved valuable in the study of Staphylococcal α-hemolysin Citation[13]. Cyt2Aa1 was chosen in preference to other Cyt δ-endotoxins because the availability of its crystal structure Citation[4] enables the position of mutated residues to be mapped on the structure of the molecule.
Materials and methods
Plasmids and oligonucleotides
Plasmid pMSV1Cyt2Aa1 (wild type) is based on pALTER (Promega) and carries the full-length cyt2Aa1 gene from Bt subsp. kyushuensisCitation[14]. pUC18 was described by Yanisch-Perron et al. Citation[15]. Two oligonucleotides were designed to change the sequence of Cyt2Aa1 from isoleucine at position 150 and from threonine at position 221 to alanine (I150A and T221A). To facilitate identification of the mutants, the oligonucleotide for I150A was designed so as to introduce a new Hae III restriction site and the T221A oligonucleotide was designed to abolish a Hind III site. DNA sequences of oligonucleotides for I150A and T221A were 5′-TTTAGACACTCAGGCCGATGAAGCATG-3′ and 5′-TAAAATGAAAGCTCTTGCTTTAGTTCAAG-3′, respectively. Oligonucleotides for error-prone PCR were universal M13 forward and reverse sequencing primers. All oligonucleotides were produced by the Oligonucleotide Synthesis Facility, Department of Biochemistry, University of Cambridge, UK.
Site-directed mutagenesis and cloning
Site-directed mutagenesis was performed using the Altered Sites II In Vitro Mutagenesis System (Promega) as described by the manufacturer. Single-stranded template was prepared from pMSV1Cyt2Aa1 using R408 helper phage. Mutagenesis reactions were used to transform the mismatch repair deficient E. coli strain ES1301 mutS by electroporation Citation[16]. Transformed cells were grown overnight in 5 ml LB (Luria-Bertani) medium containing 100 µg/ml ampicillin. Plasmid DNA was then isolated and re-transformed into E. coli JM109 or TG1 cells. Transformants were analysed for introduced mutations by restriction endonuclease digestion and DNA sequencing. Wild type and mutant genes were also sub-cloned into plasmid pUC18 for high efficient expression in E. coli.
Error-prone PCR
Error-prone PCR was performed as described by Panchal and Bayley Citation[13]. The template for PCR was the cyt2Aa1-I150A gene in plasmid pUC18. The 3′- and 5′- universal primers were as described above. Random mutations were introduced using a large number of PCR cycles (50) and a high concentration of dNTPs (0.4 mM each). PCR reactions were carried out in standard PCR buffer (Pharmacia) plus 1.0 ng of plasmid template, 0.8 mM of each primer, 3.0 mM MgCl2 and 2.5 units of Taq DNA polymerase (Pharmacia) in 50 µl reaction volume for 50 cycles at 94°C, 30 sec; 50°C, 30 sec; 72°C, 2 min (10 min for the last cycle). PCR products were then cloned into plasmid pUC18 and transformed into E. coli JM109 or TG1.
Screening for revertants
The screening method was modified from the method described by Panchal and Bayley Citation[13]. Small colonies that appeared after 8 h growth at 37°C were replicated onto nitrocellulose membranes (Gelman Sciences), which were then placed colony side up onto a second LB-ampicillin plate previously spread with 50 µl of 0.1 M IPTG and 50 µl of 2% X-gal, and incubated overnight at 37oC. The replicate colonies were lysed by removing the nitrocellulose membranes and placing them (colony side up) for 1 h on Whatman paper (no. 3) soaked with 0.1 M NaHCO3, 1% Triton X-100 (v/v) containing 2 mg/ml lysozyme. The toxin inclusions were solubilized and processed by removing the membranes and placing them on Whatman paper (no. 3) soaked with 50 mM Na2CO3 pH 10.5 containing 0.1 mg/ml proteinase K for 1 h at 37°C. The membranes were then washed with PBS buffer (8 mM Na2HPO4, 1.5 mM KH2PO4, 140 mM NaCl, 2.7 mM KCl pH 7.4) plus 1 mg/ml BSA by gently floating the membranes (colony side up) on the surface of the wash buffer for 15 min. The membranes were then inverted onto blood agar plates prepared by adding 2.5 ml of washed human blood to 100 ml of tryptone soya agar (Oxoid) held at 50°C. Colonies showing distinct zones of haemolysis within 24 h at room temperature were individually picked from the master plates for further analysis.
Protein preparation and processing
Cyt2Aa1 inclusions were released from E. coli cells by sonication as described previously Citation[17]. Prior to use, the inclusions were solubilized at 1–2 mg/ml at 37°C for 1 h in 50 mM Na2CO3 pH 10.5 with occasional shaking. Solubilized toxin was separated from insoluble materials by centrifugation at 12,000 g for 5 min and stored at −20°C in 1 ml aliquots. Concentrations of solubilized toxin were determined by the method of Bradford Citation[18] using the BioRad protein assay kit and BSA as standard. For proteolytic processing, the solubilized material was mixed with 0.1–10% (w/w) proteinase K and incubated at 37°C for 10 min to 3 h.
Circular dichroism analysis
Protein samples in 50 mM Na2CO3 pH 10.5 in a 0.02 cm cylindrical quartz cuvette were analysed using a Jasco J-715 spectropolarimeter at 25oC. The instrument was calibrated with camphorsulfonic acid (CSA) and continuously purged with nitrogen gas. Scanning was set to accumulate for 4 averaged spectra in the 180–320 nm region and subtracted with baseline spectra from buffer. All spectra were analysed and compared at the same protein concentration.
Intrinsic fluorescence spectroscopy
Cyt toxin fluorescence spectra were obtained by emission scanning of protein solution in a 10 mm path length square quartz cell. The protein samples were excited at 280 nm using a Jasco FP-6500 spectrofluorometer. The emission spectra were recorded from 300–500 nm with medium scanning speed using 3 nm of excitation and emission slit width. All spectra were subtracted with baseline spectra from buffer.
In vitro haemolysis assay
Haemolysis assays in microtitre plates were as described by Thomas and Ellar Citation[19] except that a 4% human blood (National Blood Transfusion Service) suspension in haemolysis buffer (50% PBS, 2.25% glucose, 0.05% gelatine) was used. Processed toxin samples were diluted in two-fold serial dilutions with haemolysis buffer. 0.1 ml aliquots of the diluted toxin samples were mixed with 0.1 ml of diluted blood cells and left at room temperature. The haemolytic end-point was recorded after 24 h of incubation.
Mosquito larvicidal assay
Mosquito larvicidal assays were performed as described by Koni and Ellar Citation[6] with modification. Solubilized toxin samples in 50 mM Na2CO3 pH 10.5 were precipitated by adding 0.1 volume of 11% (w/v) citric acid to lower the pH to approximately 4.5 and the samples stored at −20oC for at least 2 h until required. The material was then used directly without further manipulation. The assays were carried out 3 times in duplicate using 10 larvae of 3-day-old Aedes aegypti (reared from eggs supplied by Zeneca Agrochemical, UK and the Institute of Molecular Biology and Genetics, Mahidol University, Thailand) in 1.5 ml microtubes containing 0.9 ml of water in which the larvae were reared and 0.1 ml of precipitated toxin samples. The final concentration of toxin in each tube was a 2-fold serial dilution from 250 µg/ml to 0.25 µg/ml. The negative control contained 90 µl of 50 mM Na2CO3 pH 10.5 and 10 µl of 11% citric acid. Mortality was recorded after 24 h at room temperature.
Results
Expression and activity of primary mutants
Ward et al. Citation[20] demonstrated that replacing K154 or K225 with alanine in the closely related toxin Cyt1Aa () caused a loss of activity without affecting expression. Therefore, it was decided to substitute alanine at the equivalent residues I150 and T221 in Cyt2Aa1. Both mutant proteins expressed at levels similar to that of the wild type and were soluble in 50 mM Na2CO3 pH 10.5 without the addition of a reducing agent suggesting that the mutations did not affect dimerization and crystal packing. No differences between solubilized mutant proteins and wild type protein were detectable on SDS-PAGE (data not shown). Proteinase K treatment of the mutant proteins gave identical products to the wild type (data not shown) indicating that the processing sites in the mutants had not changed. The haemolytic activity of Cyt2Aa1-T221A was similar to the wild type, but Cyt2Aa1-I150A was not haemolytic even at very high concentrations (250 µg/ml; ). Finally analysis of both proteins using Circular Dichroism (CD) and intrinsic fluorescence revealed no significant differences in structure between the inactive mutant and the wild type (data not shown).
Table I. Activity of Cyt2Aa1-wt, Cyt2Aa1-T221A, Cyt2Aa1-I150A and revertants of Cyt2Aa1-I150A. The revertants are indicated in square bracket [].
The Cyt2Aa1-I150A mutant was therefore selected as the template for reversion mutagenesis. The equivalent mutation in Cyt1Aa (K154A) was one of 6 mutations showing low toxicity and reduced phospholipid binding, but no change in expression levels Citation[20]. All these mutations mapped to loops lying along the edge of the β-sheet adjacent to the helix pair αC-αD Citation[4]. Because alanine replaced a charged residue in 4 of these mutants and because these charged residues are not conserved between Cyt1Aa1 and Cyt2Aa1, Li et al. Citation[4] proposed that this region is required to remain exposed to the aqueous phase when β5, β6 and β7 insert into the membrane. In the remaining two low toxicity mutants, alanine was replaced with proline suggesting that main chain flexibility as well as the appropriate hydrophobicity is needed in this region. In the model for toxin insertion proposed by Li et al. Citation[4] these properties were important in allowing helix pair αC-αD to peel away from the β-sheet to lie on the membrane surface while the sheet itself rearranges to form the oligomeric trans-membrane pore. On the basis of these suggestions the loss of toxicity when the highly hydrophobic isoleucine is replaced by alanine in Cyt2Aa1-I150A could result from a disruption of the hydrophobicity which positions the surface-located αC-αD helix pair adjacent to the edge of the inserted β-sheet. Because monomer interaction following membrane insertion is crucial to the assembly of the oligomeric pore it is important to keep in mind the possibility that interference with monomer recognition could also result in loss of toxicity. If the activity of the Cyt2Aa1-I150A mutant could be recovered by substitution at other residue positions in the molecule, the location of these substitutions could therefore reveal regions important in the steps of membrane recognition, toxin insertion and pore formation.
Reversion mutagenesis
Reversion mutagenesis of the inactive Cyt2Aa1-I150A was performed using error-prone PCR. Random mutations were introduced into PCR products by using Taq polymerase which lacks 3′-5′ exonuclease activities Citation[21] and has an error rate of 2×10−4 errors/base Citation[22]. This error rate was further increased by using a large number of PCR cycles and a high concentration of dNTPs. PCR products were then cloned into pUC18, expressed in E. coli and colonies screened on blood agar plates. This screen is a powerful method to identify a few haemolytic revertants among thousands of non-haemolytic colonies Citation[13]. In the work reported here, 40 positive colonies were obtained after screening approximately 10,000 colonies. This reversion frequency of 0.4% is very similar to that reported for α-haemolysin (0.5%) Citation[13]. DNA sequencing of the positive clones revealed 28 different revertants. Of these, 13 revertants were single mutations, 12 were double mutations, 2 were triple mutations and 1 was a quadruple mutation (see ). Four single revertants were found repeatedly: [V105A] (7 colonies), [N170S] (4 colonies), [A150V] (3 colonies) and [T148I] (2 colonies). The positions of revertant residues in the primary sequence and in the toxin structure are shown in , respectively.
Figure 2. Amino acid sequence and structure of wild type Cyt2Aa1. (A) The position of the original inactive mutant (I150A) is marked by * and the positions of revertant residues are shown in bold. Proteinase K processing sites are indicated by arrows. (B) 3-D structure of Cyt2Aa1 from different views showing the positions of wild type residues that were replaced in the revertants. The images were produced by MolScript v2.0.2 Citation[33]. This Figure is reproduced in colour in Molecular Membrane Biology online.
![Figure 2. Amino acid sequence and structure of wild type Cyt2Aa1. (A) The position of the original inactive mutant (I150A) is marked by * and the positions of revertant residues are shown in bold. Proteinase K processing sites are indicated by arrows. (B) 3-D structure of Cyt2Aa1 from different views showing the positions of wild type residues that were replaced in the revertants. The images were produced by MolScript v2.0.2 Citation[33]. This Figure is reproduced in colour in Molecular Membrane Biology online.](/cms/asset/6dfb89c2-8158-4d50-b0aa-2a890c0f7be7/imbc_a_116602_uf0002_b.jpg)
Solubilization and proteolytic processing of revertant toxins
All revertant proteins expressed in E. coli at the same level as the wild type and formed typical inclusion bodies that could be solubilized in 50 mM Na2CO3 pH 10.5 without the need for a reducing agent (data not shown). This result was not unexpected since none of the revertants has a residue changed to cysteine. Cyt2Aa1 contains a single cysteine residue located near the N-terminus which does not appear to play an important role in crystal packing Citation[4]. After solubilization, all revertant proteins showed SDS-PAGE profiles similar to the wild type (data not shown).
Most revertants gave similar products to the wild type when treated with proteinase K to simulate protoxin activation (data not shown). However, 9 revertants: [F77S], [I83T], [N170S], [N8S,F47S], [I68M,S166G], [D69A,I171T], [K79R,I83T], [Q119R,T180A,I207T] and [D39G,S108P,I171T, N259D] were unusually sensitive to proteinase K digestion and were almost completely digested by 0.5% (w/w) proteinase K at 37oC for 1 h. These proteins were only partially digested by 0.1% (w/w) proteinase K under the same conditions (data not shown). Most of the mutated residues in the proteinase K-sensitive revertants were located on the surface of the molecule (see ). The remaining two mutated residues in this group (N170S and I171T) are located in the middle of β5. Interestingly 8 of these 9 protease sensitive revertants which all showed much reduced mosquitocidal and haemolytic activities compared to the wild type toxin, have a residue mutated to serine or threonine. The protease sensitivity of the relatively toxic revertant [I68M,S166G] is likely to be the result of I68M because the active form of revertant [S166G] is not sensitive to proteinase K digestion.
Activity of the revertants
The results of haemolysis assays (see ) show that none of the revertant toxins was as active as the wild type, except [A150V]. Larvicidal assays using Aedes aegypti generally showed very similar results to the haemolytic assays, but there were some revertants (e.g., [T148I] and [A185V,F205L]) with significantly less haemolytic activity but comparable larvicidal activity to the wild type ().
The revertants could be divided into three groups according to their activity. The first group, [N74D], [F77L], [V105A], [T148I], [S166G], [V195A], [S9F,N74D], [V109A,A185V], [V125A,A185T], [S166G,I171V], and [A185V,F205L], had relatively high activity similar to wild type. A second group, [T129A], [F47L,N259H], [I68M,S166G], and [F77L,S210N], had moderate activity, while a third group, containing the remaining revertants all had low activity. The revertant [D39G,S108P,I171T,N259D] may be classified into the moderate or high activity group, although its activity is very difficult to quantitate because of its protease sensitivity.
Positions of revertant residues in the structure of molecule
Two revertants ([A150V] and [T148I]) contain mutations close to or at the position occupied by A150 in the inactive primary mutant. Both of these return a hydrophobic residue to the loop at the edge of the β-sheet adjacent to the helix pair αC-αD from which isoleucine was removed in the primary inactive mutant. The fact that both revertants showed very high activity () indicated that hydrophobicity is required in this segment.
Seven of the revertants ([N74D], [F77S], [F77L], [S9F,N74D], [F77L,S210N], [I68M,S166G], [D69A,I171T]) contained mutations in the loop separating helices αA-αB. (Revertant [F77L] appears to be more active than revertants [F77S] and the double revertant [F77L,S210N], but the intrinsic activities of these three mutants are difficult to measure because their low activities are undoubtedly partly due to their increased protease sensitivity.) In the protoxin, residues in this loop play a role in stabilizing the packing of helix pair αA-αB against the β-sheet Citation[4]. It was suggested above that an impairment of the hinge function of the loop linking helix αD to the β-sheet causes the loss of activity in the Cyt2Aa1-I150A mutant. The location of these seven revertants suggests that mutations in the helix αA-αB loop can compensate for this. Since the report by Du et al. Citation[23] indicates that helices αA and αB are exposed on the membrane surface, it is possible that disruption to the connectivity between helix pair αA-αB and the β-sheet may compensate for the change in the connectivity between helix pair αC-αD and the sheet that has occurred in the Cyt2Aa1-I150A mutant. The two revertants located in β2 ([N8S,F47S], [F47L,N259H], or the adjacent β2-αA loop ([K14R,V49A,S130N], [Q50R]) may compensate by a similar mechanism.
Three revertants ([T129A], [V125A,A185T] and [K14R,V49A,S130N]) have replacements in the loop joining helices C and D. This may indicate that disruption to the connectivity between this region and the sheet can compensate for the inactivating I150A change in the segment between helix αD and the sheet. It is noteworthy that the addition of the A185T replacement in the double revertant [V125A,A185T] dramatically increases the activity. If as suggested by Li et al. Citation[4] and Du et al. Citation[23] β5, β6 and β7 from several monomers span the membrane in the oligomeric pore, these strands would have alternate residues facing the membrane lipid or the channel lumen. A185 in β6 faces the membrane lipid Citation[4] and a change to a more hydrophobic residue may favour sheet insertion. This may be a partial explanation for the activity of revertant [A185V,F205L]. However A185 is hydrogen bonded to I175 in β5 and a strengthening of this interaction in A185T may also promote β5, β6 and β7 insertion. The hydrophobicity of the residue at position 205 in the loop between helix αE and β7 is highly conserved (see ) and any restoration of activity caused by the F205L change should be due to other reasons.
Four revertants ([V109A,A185V], [V111I,K218R], [Q119R,T180A,I207T], [D39G,S108P,I171T,N259D]) are found with replacements in helix αC and one in helix αB ([I83T]). The important intra-molecular salt bridge between E192 and K218 Citation[4] is preserved in [V111I,K218R] and therefore restoration of activity is attributable to the V111I change. The finding that these helices are not membrane-protected Citation[23] suggests that in addition to their importance in connectivity to the sheet, their surface location may also be important in the monomer recognition that precedes oligomerization. This is supported by studies using synthetic peptides Citation[24], Citation[25], reporting that αA and αC had the ability to bind the membrane and self-associate. Thus changes to both the amphipathic character and sequence in the surface exposed helices may also be compensatory.
The revertants [S166G] and [S166G,I171V] show similar levels of activity, suggesting that the conservative change from isoleucine to valine in the double revertant may have no effect on the toxin activity. [S166G] is located at the N-terminal end of β5 where it forms a hydrogen bond with H158 in β4. Du et al. Citation[23] have shown that β2 remains on the membrane surface after insertion of β5. Therefore one suggestion to explain restoration of activity in this revertant would be a decrease in β2–β5 connectivity caused by substitution of glycine for serine. A similar explanation could be proposed for restoration of activity in the mutant [N170S] since N170 forms a hydrogen bond with N44 in β2. (The increased protease sensitivity of N170S makes it difficult to compare its intrinsic activity with that of S166G.).
The revertant [E178G] is located in the β5–β6 loop which is proposed to be oriented towards the cell interior during sheet insertion Citation[4]. Although 5 of the loop residues are highly conserved () the lack of conservation at this position suggests that the negative charge on E178 is not important during insertion. The ability of a glycine substitution at this position to compensate for the distal I150A substitution may suggest that loop flexibility favours membrane insertion.
Discussion
The α/β architecture of the Cyt2Aa1 toxin monomer is unusual in that the two outer layers sandwiching the β-sheet consist of helical hairpins that can lift upwards and away from the sheet without unraveling it Citation[4]. Helices αA, αB, αC and αD are amphipathic (see ) with hydrophobic residues packed against the β-sheet. The model for membrane insertion and pore formation proposes that upon membrane contact, helix pair αC-αD swings away from the sheet to lie on the membrane surface allowing β5, β6 and β7 to move into the lipid bilayer with the loop between β5 and β6 oriented towards the cell interior. Subsequent association of these inserted strands from several monomers creates an oligomeric pore Citation[4]. The appearance of new proteolytic sites upon toxin binding to the membrane and the location of these sites Citation[23] are consistent with the model. In a variation on their proposal Li et al. Citation[4] suggested that helix hairpin αA-αB might remain packed against the sheet during insertion rather than remaining on the surface. This appears to be ruled out by the recent finding that only the segment containing β4, β5, β6, αE and β7 remains tightly associated with the membrane after proteolysis of membrane bound Cyt2Aa toxin Citation[23].
Figure 3. Helical wheels of helices αA, αB, αC and αD. All helices show amphipathic character. Residues on the hydrophobic face could interact with the lipid bilayer of the membrane while residues on the hydrophylic side may interact with residues from other monomers to form the oligomer. This Figure is reproduced in colour in Molecular Membrane Biology online.
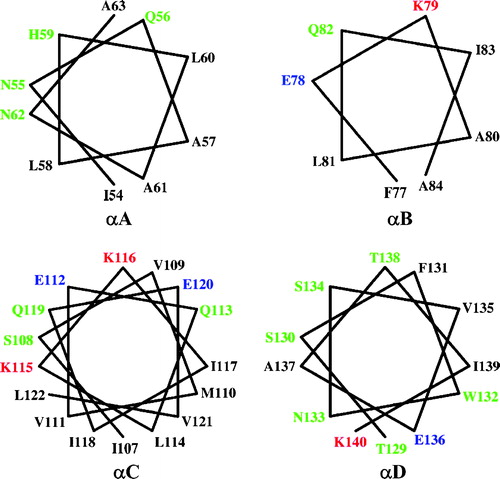
As measured by their solubility, protease sensitivity, expression levels and reaction with wild-type antibody (data not shown), the non-toxic Cyt2Aa1-I150A and the wild type toxin were identical. In addition no significant structural differences between the proteins were detectable using Circular Dichroism (CD) and intrinsic fluorescence. The dramatic difference in toxicity between the two proteins despite this similarity in properties and structure, suggests that replacement of isoleucine by alanine may affect the conformational changes required for transforming the water-soluble toxin into the membrane-bound pore form. Li et al. Citation[4] proposed a key role in membrane insertion for the hinge region between helix pair αC-αD and the β-sheet both in terms of surface-seeking ability and flexibility. Because residue 150 is located in this region, the loss of activity in the Cyt2Aa1-I150A mutant may originate in changes in one or both of these properties. By reversion mutagenesis we have located residues proximal and distal from this mutation that can restore toxin activity in this mutant. Comparative analysis of these revertants may therefore help us to understand the insertion mechanism.
Proteolytic activation of the Cyt2Aa1 protoxin removes 33–37 amino acids at the N-terminus and 22-31 amino acids at the C-terminus Citation[6] to generate either the fully-activated (S38 to S228) or the partially activated toxin (T34 to F237 or S38 to F237 or T34 to S228). Mutation of residues upstream or downstream of these regions should have no effect on toxin activity. Therefore, residues N8S, S9F, K14R, N259H and N259D contained in revertants [N8S,F47S], [S9F,N74D], [K14R,V49A,S130N], [F47L,N259H] and [D39G,S108P,I171T,N259D] are not considered to be responsible for restoring the activity of these revertants.
Combining our results with the findings of Du et al. Citation[23] lead us to refine the model proposed by Li et al. Citation[4] to describe the events taking place during pore formation (see ). The refined model proposes that upon membrane contact both helix hairpins αA-αB?, αC-αD swing away from the sheet. These helices together with αF, β2, β3, β4 and the loops between them locate on the membrane surface with β5, β6 and β7 inserting into the membrane. After insertion, this places alanine in the primary inactive Cyt2Aa1-I150A mutant on the membrane surface.
Figure 4. Schematic diagram of a possible model for insertion of Cyt2Aa1 into the cell membrane. The helices αA, αB, αC and αD bi nd the membrane and form an oligomer with other protomers. The regions between αD and β5 and between β6 and β7 act as hinges during the conformational changes following membrane binding. Sheets β5, β6 and β7 insert into the membrane and form an oligomer with similar sheets from other protomers to form a β-barrel pore. Residues in helices and sheets are coloured according to their hydrophobicity (red for hydrophobic, yellow for polar or charged and green for tyrosine). Sheet β3 which links helices αB and αC is not shown. The positions of the primary inactive mutant (I150A) and the high activity revertants are shown in ball and stick as the original residues in the wild type. I175, A182 and A225 are the residues at the C or N termini of β5, β6 and β7, respectively.
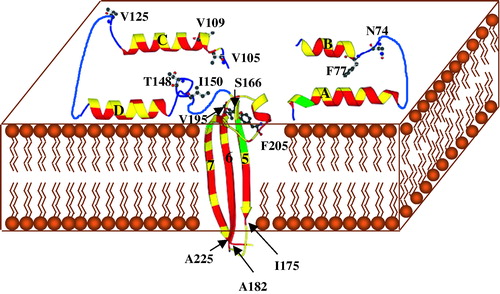
Experiments to investigate the effect of temperature on pore formation of Cyt2Aa1 and studies on membrane insertion and pore formation of acrylodan-labelled mutants of Cyt2Aa1 Citation[17], Citation[26] show that the toxin binds and inserts into the membrane as a monomer. Oligomerization occurs when toxin molecules bind in close proximity to each other and the pores are formed when oligomers reach a critical number. Cyt2Aa thus exists in three different configurations (water-soluble monomer, membrane inserted monomer and membrane inserted oligomer) in any or all of which a residue may have an important role. Toxicity may therefore be restored in a revertant because of compensatory changes in the steps of membrane insertion or oligomerization or both. Using the refined model, it could be suggested that in addition to a possible impairment of the hinge region between helix pair αC-αD and the sheet, the loss of toxicity in Cyt2Aa1-I150A could be due to the reduction of binding between the toxin molecule and the membrane surface.
If the monomer-monomer recognition that precedes pore formation involves recognition/association between the surface exposed elements, then clearly toxicity may be affected by a broad range of residue changes in the elements. For example αA, αB, and αC are amphipathic (see ) and the model proposes that residues on the hydrophobic face interact with the lipid bilayer while residues on the other side of the helix are available to recognize residues from other monomers to form the oligomer. V109 is the only hydrophobic residue located on the hydrophilic face of helix C () which is known to be capable of self-association in a membrane Citation[24], Citation[25]. Substitution of V109 by alanine at this position in revertant [V109A,A185V] may therefore further stabilize the surface location of this element and/or increase its ability to oligomerize with other monomers. Many of the other high activity revertants that map to the loops or helices exposed on the surface in the model e.g., N74D, F77L, V105A, V109A, V125A, and T148I, may compensate by similar mechanisms.
The model shows β4 to be on the membrane surface rather than inserted in the bilayer. Proteolysis of membrane-bound Cyt2Aa1 occurred at a number of cleavage sites in the αD-β4 loop to leave β4 associated with β5, β6 and β7 in the membrane protected fragment Citation[23]. However proteolysis of membrane bound Cyt1Aa revealed cleavage sites in β4 including one at the C-terminal end. This indicates that in Cyt1Aa, β4 is membrane bound but not inserted and we suggest this is also likely to be true for Cyt2Aa1.
Li et al. Citation[4] proposed that β5, β6 and β7 from several toxins form a pore based on a β-barrel structure. Experiments using polarity sensitive acrylodan-labeled toxin have confirmed that the pore is oligomeric with each monomer contributing three strands (β5, β6, β7) Citation[17]. Strands β5 and β7 show a pattern of alternating hydrophobic and hydrophilic residues similar to that of the known membrane spanning regions of α-hemolysin Citation[27] and anthrax toxin protective antigen Citation[28]. Strand β6 is highly hydrophobic and the pattern is less obvious than in β5 and β7. The amino acid sequences of β5, β6 and β7 are not conserved but the alternating hydrophobic and hydrophilic pattern is highly conserved in all Cyt δ-endotoxins ().
The crystal structures of the transmembrane domains of several proteins have been determined. In many of these structures, bands of aromatic residues (Tyr, Phe and Trp) lie at the hydrophobic/hydrophilic interface of the lipid bilayer and are considered to be a general characteristic of transmembrane proteins such as α-hemolysin Citation[27], OmpA Citation[29] and porin from Rhodobacter capsulatusCitation[30]. Although the three β-barrel structures are diverse in terms of subunit stoichiometry and architecture, each shows the band of aromatic residues (Tyr, Phe and Trp) at the hydrophobic/hydrophilic interface of the lipid bilayer. These residues have intermediate polarity and are considered to interact favorably with the membrane at the hydrophobic/hydrophilic interface. In Cyt2Aa1, there are three tyrosine residues near the N-terminus of β5 and β7 (Y167, Y169 in β5 and Y213 in β7) which are conserved in all Cyt δ-endotoxins (). After the Cyt toxin monomers have assembled and formed a pore in the membrane, these residues could form a band around the β-barrel at a point that the model proposes to be the point of entry of β5 and β7 (). This band would be very similar in position to the band in α-hemolysin.
The recent solution of the crystal structure of a fungal pore forming toxin, volvatoxin A2 (VVA2), has shown it to be almost identical to Cyt2Aa1 Citation[31]. Electron microscopy of this toxin suggested that it could form an oligomer with 18 subunits Citation[31]. Investigation of truncated fragments of VVA2 suggested that the N-terminal fragment domain which consists mostly of α-helices is responsible for oligomerization and the C-terminal fragment domain consists of three β-sheets inserted into the membrane Citation[32]. In Cyt2Aa1, sheets β5, β6 and β7 were reported to insert into the membrane and are protected from protease digestion Citation[23]. Experiments using synthetic peptides corresponding to helices αA and αC Citation[25] demonstrated that these helices could form oligomers. We therefore propose that Cyt2Aa1 forms a β-barrel transmembrane pore by inserting β5, β6 and β7 from 18 toxin molecules into the membrane with helices αA and αC playing a role in the oligomerization step.
We thank Dr Jade Li for valuable suggestions and discussion and Dr Paul Davis for assistance with graphics and multiple sequence alignment and Zeneca Agrochemicals, UK and the Institute of Molecular Biology and Genetics, Mahidol University, Thailand, for supplying mosquito eggs. Support to B.P. from the Thailand National Center for Genetic Engineering and Biotechnology is gratefully acknowledged.
References
- Schnepf HE, Crickmore N, Van Rie J, Lereclus D, Baum J, Feitelson J, Zeigler DR, Dean DH. Bacillus thuringiensis and its pesticidal proteins. Microbiol Mol Biol Rev 1998; 62: 775–806
- Crickmore N, Zeigler DR, Feitelson J, Schnepf E, Van Rie J, Lereclus D, Baum J, Dean DH. Revision of the nomenclature for the Bacillus thuringiensis pesticidal crystal proteins. Microbiol Mol Biol Rev 1998; 62: 807–813
- Li J, Carroll J, Ellar DJ. Crystal structure of insecticidal δ-endotoxin from Bacillus thuringiensis at 2.5Å resolution. Nature 1991; 353: 815–821
- Li J, Koni PA, Ellar DJ. Structure of the mosquitocidal δ-endotoxin CytB from Bacillus thuringiensis sp. kyushuensis and implications for membrane pore formation. J Mol Biol 1996; 257: 129–152
- Knowles BH, Ellar DJ. Colloid-osmotic lysis is a general feature of the mechanism of action of Bacillus thuringiensis δ-endotoxins with different specificity. Biochim Biophys Acta 1987; 924: 509–518
- Koni PA, Ellar DJ. Biochemical characterisation of Bacillus thuringiensis cytolytic delta-endotoxins. Microbiol 1994; 140: 1869–1880
- Chow E, Singh FJP, Gill SS. Binding and aggregation of the 25 kilodalton toxin of Bacillus thuringiensis subsp. israelensis to insect cell membranes and alteration by monoclonal antibodies and amino acid modifiers. Appl Environ Microbiol 1989; 55: 2779–2788
- Drobniewski FA, Ellar DJ. Purification and properties of a 28-kilodalton hemolytic and mosquitocidal protein toxin of Bacillus thuringiensis subsp. darmstadiensis 73-E10-2. J Bacteriol 1989; 171: 3060–3067
- Knowles BH, Blatt MR, Tester M, Horsnell JM, Carroll J, Menestrina G, Ellar DJ. A cytolytic δ-endotoxin from Bacillus thuringiensis var. israelensis forms cation-selective channels in planar lipid bilayers. FEBS Lett 1989; 244: 259–262
- Knowles BH, White PJ, Nicholls CN, Ellar DJ. A broad spectrum cytolytic toxin from Bacillus thuringiensis var. kyushuensis. Proc R Soc Lond 1992; 248: 1–7
- Maddrell SHP, Lane NJ, Harrison JB, Overton JA, Moreton RB. The initial stages in the action of an insecticidal δ-endotoxin of Bacillus thuringiensis var. israelensis on the epithelial cells of the malpighian tubules of the insect, Rhodnius prolixus. J Cell Sci 1988; 90: 131–144
- Maddrell SHP, Overton JA, Ellar DJ, Knowles BH. Action of activated 27000 Mr toxin from Bacillus thuringiensis var. israelensis on malpighian tubules of the insect, Rhodnius prolixus. J Cell Sci 1989; 94: 601–608
- Panchal RG, Bayley H. Interactions between residues in Staphylococcal α-hemolysin revealed by reversion mutagenesis. J Biol Chem 1995; 270: 23072–23076
- Koni PA, Ellar DJ. Cloning and characterization of a novel Bacillus thuringiensis cytolytic delta-endotoxin. J Mol Biol 1993; 229: 319–327
- Yanisch-Perron C, Vieira J, Messing J. Improved M13 phage cloning vectors and host strains: Nucleotide sequences of the M13MP18 and pUC19 vectors. Gene 1985; 33: 103–119
- Dower WJ, Miller JF, Ragsdale CW. High efficiency transformation of E. coli by high voltage electroporation. Nucleic Acids Res 1988; 16: 6127–6145
- Promdonkoy B, Ellar DJ. Membrane pore architecture of a cytolytic toxin from Bacillus thuringiensis. Biochem J 2000; 350: 275–282
- Bradford MM. A rapid and sensitive method for the quantitation of microgram quantities of protein utilizing the principle of protein-dye binding. Anal Biochem 1976; 72: 248–254
- Thomas WE, Ellar DJ. Bacillus thuringiensis var. israelensis crystal δ-endotoxin: Effects on insect and mammalian cells in vitro and in vivo. J Cell Sci 1983; 60: 181–197
- Ward ES, Ellar DJ, Chilcott CN. Single amino acid changes in the Bacillus thuringiensis var. israelensis δ-endotoxin affect the toxicity and expression of the protein. J Mol Biol 1988; 202: 527–535
- Tindall KR, Kunkel TA. Fidelity of DNA synthesis by the Thermus aquaticus DNA polymerase. Biochemistry 1988; 27: 6008–6013
- Cha RS, Thilly WG. Specificity, efficiency, and fidelity of PCR. PCR Methods Applic 1993; 3: 18–29
- Du J, Knowles BH, Li J, Ellar DJ. Biochemical characterization of Bacillus thuringiensis cytolytic toxins in association with a phospholipid bilayer. Biochem J 1999; 338: 185–193
- Gazit E, Shai Y. Structural characterization, membrane interaction, and specific assembly within phospholipid membranes of hydrophobic segments from Bacillus thuringiensis var. israelensis cytolytic toxin. Biochemistry 1993; 32: 12363–12371
- Gazit E, Burshtein N, Ellar DJ, Sawyer T, Shai Y. Bacillus thuringiensis cytolytic toxin associates specifically with its synthetic helices A and C in the membrane bound state: Implication for the assembly of oligomeric transmembrane pores. Biochemistry 1997; 36: 15546–15554
- Promdonkoy B, Ellar DJ. Investigation of the pore forming mechanism of a cytolytic δ-endotoxin from Bacillus thuringiensis. Biochem J 2003; 374: 255–259
- Song L, Hobaugh MR, Shustak C, Cheley S, Bayley H, Gouaux JE. Structure of Staphylococcal α-hemolysin, a heptameric transmembrane pore. Science 1996; 274: 1859–1866
- Petosa C, Collier RJ, Klimpel KR, Leppla SH, Liddington RC. Crystal structure of the anthrax toxin protective antigen. Nature 1997; 385: 833–838
- Pautsch A, Schulz GE. Structure of the outer membrane protein A transmembrane domain. Nature Struct Biol 1998; 5: 1013–1017
- Weiss MS, Schulz GE. Structure of porin refined at 1.8 Å resolution. J Mol Biol 1992; 227: 493–509
- Lin SC, Lo YC, Lin JY, Liaw YC. Crystal structures and electron micrographs of fungal volvatoxin A2. J Mol Biol 2004; 343: 477–491
- Weng YP, Lin YP, Hsu CI, Lin JY. Functional domains of a pore-forming cardiotoxic protein, volvatoxin A2. J Biol Chem 2004; 279: 6805–6814
- Kraulis P. MolScript: A program to produce both detailed and schematic plots of protein structures. J Appl Crystallog 1991; 24: 946–950