Abstract
Glycoconjugates are utilized by eukaryotic organisms ranging from yeast to humans for the cell surface expression of a wide variety of proteins and lipids. These glycoconjugates are expressed as enzymes or receptors and serve a diversity of functions, including cell signaling and cell survival. In parasitic protozoans, glycoconjugates play roles in infectivity, survival, virulence and immune evasion. Among the alternate glycoconjugate structures that have been identified, glycosylphosphatidylinositols (GPIs) represent a universal structure for the anchorage of proteins, lipids, and phosphosaccharides to cellular membranes. Biosynthesis of the GPI is a multi-step process that culminates in the attachment of the assembled GPI to a precursor protein. This final step in the transfer of the GPI to a protein is catalyzed by GPI8 of the putative transamidase complex (TAM). GPI8 functions dually to perform the proteolytic cleavage of the C-terminal signal sequence of the precursor protein, followed by the formation of an amide bond between the protein and the ethanolamine phosphate of the GPI. This review summarizes the current aggregate of biochemical, gene-disruption and active site mutagenesis studies, which provide evidence that GPI8 is responsible for the protein-GPI anchoring reaction. We describe recently published studies that have identified other potential components of the TAM complex and that have elucidated their likely role in protein-GPI attachment. Further, we discuss the biochemical, molecular and functional differences between protozoan and mammalian GPI8 and the protein-GPI anchoring machinery. Finally, we will present the implications of these studies for the development of anti-parasite drug therapies.
Overview of glycoconjugates and their significance
Glycosylphosphatidylinositols (GPIs) attach a diverse group of macromolecules to the plasma membrane of eukaryotes Citation[1]. In higher eukaryotes, GPIs are found on a minor group of proteins. In parasitic protozoans, the majority of the cell surface proteins are anchored by GPIs. A combination of molecular, biochemical, and mutagenesis approaches has demonstrated that the GPI-anchor consists of a basic core glycan structure that is highly conserved among all eukaryotic organisms (reviewed in Citation[1–3]). The structural differences are represented in the extensive variability in the carbohydrate side chain modifications and lipids among the divergent eukaryotic organisms, as well as between species, strains and/or developmental stages of lower eukaryotes Citation[4], Citation[5]. In addition to GPI-anchored proteins, parasitic protozoans also express GPI-related complex glycophospholipids Citation[6]. Thus, GPI-anchored macromolecules are classified into three main groups, i.e., protein anchoring GPIs, glycoinositol-phospholipids (GIPLs) and GPI-phosphosaccharides Citation[3].
Parasitic protozoans of the Kinetoplastida order, i.e., Trypanosoma brucei species, Trypanosoma cruzi, and Leishmania species, are the causative agents of important human and livestock diseases. Numerous studies support the conclusion that glycoconjugates play critical roles in trypanosomes attachment to host cells, as well as in invasion, differentiation and replication. Examples of GPI-anchored proteins in these protozoans include variant surface glycoprotein (VSG) and procyclic acidic repetitive protein (PARP) of Trypanosoma brucei; several proteins expressed in the infective trypomastigote (1G7, SSP-3, mucins, members of trans-sialidase superfamily) and intracellular amastigote (SSP-4, ASP-1, ASP-2) stages of T. cruzi; and the GP63 and PSA-2 of Leishmania. Other unique GPI-macromolecules that are abundantly expressed in trypanosomes include GIPLs, lipophosphoglycan (LPG), and proteophosphoglycan (PPG) of Leishmania, and GIPLs, phosphoglycan (PG), and lipopeptidophosphoglycan (LPPG) of T. cruzi. A detailed discussion of the biological significance of GPI-glycoconjugates in protozoans, as well as in yeast and mammalian cells, can be found in several reviews Citation[3], Citation[4], Citation[6–17].
Several investigators have utilized genetic approaches to evaluate the overall importance of glycoconjugates in parasitic trypanosomes. In early studies, phenotypic GPI mutants of T. cruzi and Leishmania were generated by episomal over-expression of T. brucei GPI-phospholipase C (GPI-PLC) Citation[18–20]. In both T. cruzi and Leishmania, GPI-PLC-mediated cleavage of GPI intermediates resulted in a depletion of GPI-anchored proteins as well as GIPLs. The GPI-PLC-expressing T. cruzi and Leishmania grew well in axenic cultures as epimastigotes and promastigotes, respectively. However, these mutants exhibited an inability to replicate as intracellular amastigotes and a failure to maintain active infection in animal models of Chagas disease and Leishmaniasis, respectively. The loss of virulence in GPI-PLC expressing T. cruzi and Leishmania suggested the importance of GPIs for completion of their life cycle. Yet GPI-PLC also acts on inositolphospholipids (PIs) Citation[21], which are important second messengers in signal transduction-mediated cellular functions, including nuclear regulatory pathways, in eukaryotic organisms Citation[22–24]. In Leishmania expressing GPI-PLC, PIs were not affected while the potential contribution of PI depletion to the observed phenotype was not examined in T. cruzi. Furthermore, given a general depletion of GPI precursors, these studies did not distinguish between the relative significance of GIPLs versus protein-GPIs in parasite replication and survival. Thus, focus has been placed on identification and functional characterization of the genes involved in GPI biosynthesis and/or attachment (see ).
Figure 1. Biosynthesis of glycosylphosphatidylinositol (GPI)-glycoconjugates. Shown is the GPI biosynthesis pathway in eukaryotes. Steps that are unique to the respective organism are indicated as follows: yyeast; mmammalian cells (reviewed in Citation[134]). GIPLs and the GPI-phosphosaccharides, e.g., lipophosphoglycan (LPG) or lipophosphopeptidoglycan (LPPG), are formed from distinct intermediates in the GPI biosynthesis pathway, designated, respectively as PI2 and M1 (Manα1-4GlcN-PI) Citation[135]. Specific enzymatic reactions: (1) GlcNAc transferase complex, addition of GlcNAc to PI; (2) GlcNAc-PI-de-N-acetylase, removal of N-acetyl group from GlcNAc; (2A) inositol-acyltransferase, addition of acyl group to GlcN-PI at position 2y,m; (3) GPI-α1-4mannosyltransferase (GPI-α1-4MT), transfer of mannose to position 4 of GlcN; (F) Flippase, translocation of GPI intermediate from the cytoplasmic to the luminal face of endoplasmic reticulum; (3A) Ethanolamine phosphotransferase (EtNPT-I), addition of EtNP to the first mannosey,m; (4) GPI-α1-6MT, transfer of mannose to position 6 of the first mannose; (5) and (5A) GPI-α1-2MT, transfer of mannose to position 2 of the second and thirdy mannose, respectively; (6) EtNPT-III, transfer of EtNP to the third mannose at position 6; (6A) EtNPT-II, transfer of EtNP to the second mannose at position 6y; (7) Transamidase complex, cleavage of C-terminal GPI addition signal sequence (ss) of the precursor protein and attachment of GPI. This Figure is reproduced in color in Molecular Membrane Biology online.
![Figure 1. Biosynthesis of glycosylphosphatidylinositol (GPI)-glycoconjugates. Shown is the GPI biosynthesis pathway in eukaryotes. Steps that are unique to the respective organism are indicated as follows: yyeast; mmammalian cells (reviewed in Citation[134]). GIPLs and the GPI-phosphosaccharides, e.g., lipophosphoglycan (LPG) or lipophosphopeptidoglycan (LPPG), are formed from distinct intermediates in the GPI biosynthesis pathway, designated, respectively as PI2 and M1 (Manα1-4GlcN-PI) Citation[135]. Specific enzymatic reactions: (1) GlcNAc transferase complex, addition of GlcNAc to PI; (2) GlcNAc-PI-de-N-acetylase, removal of N-acetyl group from GlcNAc; (2A) inositol-acyltransferase, addition of acyl group to GlcN-PI at position 2y,m; (3) GPI-α1-4mannosyltransferase (GPI-α1-4MT), transfer of mannose to position 4 of GlcN; (F) Flippase, translocation of GPI intermediate from the cytoplasmic to the luminal face of endoplasmic reticulum; (3A) Ethanolamine phosphotransferase (EtNPT-I), addition of EtNP to the first mannosey,m; (4) GPI-α1-6MT, transfer of mannose to position 6 of the first mannose; (5) and (5A) GPI-α1-2MT, transfer of mannose to position 2 of the second and thirdy mannose, respectively; (6) EtNPT-III, transfer of EtNP to the third mannose at position 6; (6A) EtNPT-II, transfer of EtNP to the second mannose at position 6y; (7) Transamidase complex, cleavage of C-terminal GPI addition signal sequence (ss) of the precursor protein and attachment of GPI. This Figure is reproduced in color in Molecular Membrane Biology online.](/cms/asset/bdc60b6e-b242-45e3-a6e2-e3ac9d34fa10/imbc_a_160132_f0001_b.jpg)
With the elucidation of the GPI biosynthesis pathway(s), the specific importance of GIPLs in comparison to protein-GPIs to growth and virulence of parasitic protozoans could be delineated. Studies of several L. mexicana and L. major mutants with partial or complete defects in expression of protein-GPIs Citation[18], Citation[20], Citation[25–28], LPG Citation[11], Citation[12], Citation[29–36], or the depletion of a combination of protein-GPIs, LPG and/or GIPLs Citation[26], Citation[37–39] present a complex portrait of the requirement for distinct classes of GPI-macromolecules in development and virulence of these parasites. Neither Leishmania species depend upon protein-GPIs for axenic growth as promastigotes Citation[18], Citation[26], Citation[27]. In L. major, mutants with selective loss of protein-GPIs, e.g., reduced expression of GP63, or complete loss of LPG expression were less virulent in mice Citation[11], Citation[12], Citation[25], Citation[34]. However, loss of combined LPG/GIPL expression in L. major did not ultimately affect amastigote survival, as disease development occurred, albeit in a delayed fashion Citation[39]. In L. mexicana, mutants with defects in only one class of GPI-macromolecules were capable of infecting macrophages in vitro remained virulent in mice Citation[27], Citation[32], Citation[33]; and mutants in which all classes of GPI-macromolecules were affected did not exhibit reduced macrophage infectivity or virulence Citation[38]. Thus, LPG appears to be more important to virulence in L. major than in L. mexicana (reviewed in Citation[14]) and the amenability of the protein-GPI pathway as a chemotherapeutic target will likely be dependent upon the Leishmania species. In T. brucei, protein-GPIs were found to be essential to survival of bloodstream forms and were required for procyclics to establish infection in tsetse flies Citation[40], Citation[41]. To date, however, no genetic mutants of the T. cruzi protein-GPI, GPI-phosphosaccharide and/or GIPL biosynthetic pathways have been developed. In comparison to the effects of glycoconjugate deficiency in these trypanosomatids, mammalian cell mutants defective in GPI biosynthesis or protein-GPI anchoring machinery are viable Citation[42–47], although an acquired genetic deficiency in humans Citation[48] or disruption of specific GPI-anchored proteins in mice and/or cell lines results in developmental anomalies and selective defects in hematopoietic cells Citation[49–52]. The results of the genetic studies outlined above have important implications, as enzymes involved in GPI biosynthesis and/or protein-GPI anchoring might serve as potential targets for chemotherapeutic interventions of parasitic diseases.
Characteristics of proteins anchored by GPI
Newly synthesized proteins that are destined to be GPI anchored have two signal peptides (see ). First, the precursor protein contains an N-terminal signal peptide that directs the translocation of the protein across the endoplasmic reticulum (ER) membrane Citation[53–55]. Second, this protein contains a C-terminal GPI anchor addition signal sequence that is cleaved and replaced by the GPI anchor Citation[56]. The analysis of the primary sequences and the signal sequence mutagenesis of proteins that receive a GPI have been used to determine the characteristic features of the GPI anchor addition signal sequence in different organisms Citation[57–64]. These studies have revealed that a GPI addition signal consists of a stretch of three amino acids (ω, ω + 1, ω + 2), followed by a hydrophilic spacer segment of 10–12 amino acids, and a terminal hydrophobic segment of 12–20 amino acids Citation[65]. The ω residue on the N-terminal side of the cleavage point and GPI attachment is restricted to six amino acids with small side chains, the ω + 2 is restricted to relatively small amino acids, whereas ω + 1 accepts every amino acid except proline and tryptophan Citation[59], Citation[62]. The length of the spacer region and the extent of hydrophobicity in the hydrophobic region govern the efficiency of protein-GPI anchoring. These features have been used to create computer algorithms, e.g., DGPI, to predict the presence of C-terminal GPI addition signal in protein sequences Citation[66].
Figure 2. Characteristic features of the proteins anchored by GPI. Precursor proteins destined to receive a GPI contain two signal sequences, an N-terminal signal sequence and a C-terminal signal sequence, which respectively direct the translocation of the protein to the endoplasmic reticulum (ER) and the anchoring of the GPI to the protein. The C-terminal signal sequence is composed of cleavage attachment (C/A) site of three amino acids, referred to as ω (omega), ω + 1, ω + 2; a short hydrophilic spacer element (designated ---); and a series of hydrophobic amino acids (hydrophobic domain). Cleavage occurs between the ω and ω + 1 site and is followed by GPI attachment Citation[76].
![Figure 2. Characteristic features of the proteins anchored by GPI. Precursor proteins destined to receive a GPI contain two signal sequences, an N-terminal signal sequence and a C-terminal signal sequence, which respectively direct the translocation of the protein to the endoplasmic reticulum (ER) and the anchoring of the GPI to the protein. The C-terminal signal sequence is composed of cleavage attachment (C/A) site of three amino acids, referred to as ω (omega), ω + 1, ω + 2; a short hydrophilic spacer element (designated ---); and a series of hydrophobic amino acids (hydrophobic domain). Cleavage occurs between the ω and ω + 1 site and is followed by GPI attachment Citation[76].](/cms/asset/15b0d4b9-0b4f-4ba9-87d2-b1de9cd2d029/imbc_a_160132_f0002_b.gif)
Recombination and mutagenesis studies have indicated that variability exists in the acceptable GPI addition signal sequence between organisms. The GPI addition signal sequence of T. brucei VSG did not function in mammalian cells. This difference was proposed to be due mainly to the amino acids at the ω and ω + 2 sites, since the amino acids present at these sites in parasite proteins are larger than those found in mammalian proteins. VSG, in which ω and ω + 2 amino acids were replaced by those found in decay accelerating factor (DAF), was appropriately processed, anchored to a GPI and displayed on the mammalian cell surface Citation[67], Citation[68]. These studies have suggested that there are differences in the active site of the enzyme involved in GPI transfer to proteins between higher eukaryotes and protozoans Citation[64], Citation[67]. If such is the case, it should be possible to design specific inhibitors of the protozoan protein-GPI anchoring machinery that do not affect mammalian activity and thereby serve as a selective therapeutic drug to treat parasitic diseases.
Biochemical characterization of the protein-GPI anchoring reaction
The early steps in the assembly of GPIs have been demonstrated to occur on the cytoplasmic face of the ER Citation[69–71]. A flippase enzyme is believed to accomplish the change in orientation of GPI intermediates from the cytosolic to the lumenal face of the ER membrane Citation[72–74] followed by assembly of precursor GPI. The subsequent transfer of GPI to protein occurs on the luminal face of the ER where precursor proteins are translocated Citation[55], Citation[70], Citation[75]. The final step of protein-GPI assembly, i.e., the attachment of the preformed GPI to a precursor protein involves: (1) the proteolytic cleavage of the C-terminal GPI addition signal sequence of precursor protein, and (2) the formation of a covalent bond between a carboxyl group of the protein and the amino group of ethanolamine phosphate of the GPI Citation[76]. Based upon biochemical evidence, the two functionalities, endoproteolysis and amidation, are accomplished by a single enzyme, which was later identified to be encoded by GPI8.
Several approaches have been used for the biochemical characterization of the protein-GPI attachment reaction in different organisms. Reporter assays have been utilized to monitor the steps involved in GPI-anchoring using precursor proteins, either endogenous protein substrates Citation[77–80] or reporter proteins such as placental alkaline phosphatase (PLAP), in yeast and mammalian cells Citation[59], Citation[78] or VSG117 in T. brucei Citation[80]. An in vitro translation assay was developed as a convenient system to assess the post- (or co-) translational processing and GPI-anchoring of the precursor protein in rough microsomal membranes (RMs) of yeast Citation[79] and mammalian cells Citation[81]. The kinetics of precursor protein (prepro-α factor in yeast and prepro-mini-PLAP in mammalian cells) processing to a GPI-anchored mature form was observed by pulse-chase/immunoprecipitation and SDS-PAGE. These studies were the first to show that enzymatic cleavage of the N-terminal ER targeting signal sequence and of the C-terminal GPI anchor addition signal sequence, as well as protein-GPI attachment, occur in microsomes. The inability to detect processed PLAP (i.e., PLAP from which both signal sequences have been cleaved but to which GPI is not attached) in these assays led to a suggestion that removal of the C-terminal GPI addition signal sequence and addition of GPI occur either very rapidly or simultaneously, and thus, were most likely catalyzed by a single enzyme Citation[60], Citation[82]. This idea was sustained by the results of assays utilizing RMs from GPI-anchoring defective mutants; only the N-terminal signal sequence was processed, and the pro-PLAP form accumulated, suggesting that the same enzyme performs proteolytic cleavage of the C-terminal GPI addition signal sequence, as well as the linkage of the GPI to protein Citation[44].
In T. brucei, a microsomal assay using procyclic forms was developed to study the processing and GPI-anchoring of a reporter precursor protein, the non-endogenous VSG variant, VSG117. The fate of the VSG reporter protein could be monitored by metabolic labeling, followed by immunoprecipitation with anti-VSG117 antibody. The immunoprecipitated antigen-antibody complex was resolved by SDS-PAGE and visualized by fluorimetry. Along with the observations similar to those made with yeast and mammalian microsomes, the GPI was incorporated into VSG117 in the absence of an energy source Citation[80], as previously described for endogenous VSG Citation[77]. The activation of a protein carboxyl group and its condensation with an appropriate nucleophile in the absence of an energy source is, generally, accomplished by a transamidase Citation[83–85]. It was, therefore, suggested that addition of a GPI to protein most likely involves a nucleophilic attack by the ethanolamine group of the GPI on an activated carbonyl on the ω residue of the pro-protein Citation[86]. Subsequently, hydrazine and hydroxylamine, artificial substrates that can act as nucleophilic acceptors in transpeptidase and transamidase reactions, respectively, were added as co-reactants in the cell-free assay system Citation[80], Citation[86], Citation[87]. Incubation of T. brucei RMs with hydrazine resulted in VSG117 release that was optimal at 37°C, suggestive of enzyme-mediated replacement of GPI by hydrazine. Monitoring of this reaction following a complete inhibition of GPI anchor biosynthesis by mannosamine demonstrated that hydrazine was incorporated into VSG, acting directly as a nucleophilic amine to form a hydrazide with the activated VSG-carbonyl intermediate Citation[80], Citation[88]. Similarly, hydrazine and hydroxylamine competed with GPIs in the prepro-mini-PLAP assay with mammalian cell RMs, leading to the formation of mini-PLAP-hydrazide and -hydroxamate derivatives, respectively Citation[86]. The incorporation of hydrazine and hydroxylamine was enzyme catalyzed, as addition of heated or disaggregated RMs, detergent extraction of RMs, or incubation without RMs did not result in GPI attachment to PLAP and VSG Citation[80], Citation[86]. Collectively, these studies provided evidence that protein-GPI anchoring is an enzyme-catalyzed endoproteolysis/amidation reaction in yeast, mammalian cells and trypanosomes.
Identification of the gene (GPI8) involved in protein-GPI anchoring reaction
Both reverse and forward genetic approaches were utilized in different eukaryotic organisms to identify GPI8, the gene that encodes the enzyme responsible for protein-GPI anchoring. The first indication that GPI8 is essential in a protein-GPI anchoring reaction was provided by studies of the yeast Saccharomyces cerevisiae. Several temperature-sensitive (ts), mutant yeast strains, defective in their ability to express GPI-anchored proteins on their surface, were generated via chemical mutagenesis Citation[89]. By transforming the ts mutants with a yeast chromosomal DNA library, the complementing gene that restored the surface expression of GAS1, was isolated, sequenced and named yGPI8. Deletion of yGPI8 in a parental wild-type strain proved to be lethal Citation[90], as would be hypothesized from studies indicating that GPI-anchored proteins are essential in yeast Citation[91–95]. The human homologue of yGPI8, hGPI8, was able to rescue the growth of these ts yeast mutants Citation[90].
Unlike in yeast, mammalian cells appear to be less dependent in vitro upon protein-GPIs. Thus, viable mutants defective in GPI-anchoring were derived by anti-DAF-based negative fluorescent cell sorting of N-methyl-N'-nitro-N-nitrosoguanidine pretreated human lymphoblast cells Citation[43]. Analysis of the putative GPI precursors in the mutant lines led to the identification of class K mutants that exhibited markedly increased amounts of mature GPIs, but were deficient in a step preliminary to or associated with protein transfer of assembled GPIs Citation[43], Citation[90]. The wild-type hGPI8 and the chimeric yGpi8 in which C-terminal residues were replaced by hGPI8 peptide, but not the wild-type yGPI8, complemented the class K mutants, restoring the surface expression of GPI-anchored DAF Citation[45]. These studies led to a conclusion that: (i) GPI8 plays a role in protein anchoring of GPI, and (ii) hGPI8 is a functional homologue of yGPI8. However, yGPI8 lacks certain features of the human homologue.
In L. mexicana, T. brucei, and T. cruzi, the GPI8 genes were identified by a forward genetics approach. A L. mexicana GPI8 null mutant (LmΔGPI8), generated by targeted disruption of GPI8 alleles, was shown to be deficient in surface expression of the major GPI-anchored protein, GP63, that was secreted in unanchored form Citation[27], Citation[96]. Expression of the non-protein-linked glycoconjugates (LPG and GIPLs) was unaffected. This mutant was viable in the promastigote stage and was infective to animals, which showed that GPI-anchored proteins are not essential for L. mexicana development. Disruption of GPI8 in T. brucei (TbΔGPI8) resulted in a loss of GPI-anchored proteins (e.g., procyclin) from the cell surface of procyclic forms and lead to the accumulation of precursor GPIs. The ΔGPI8 procyclics, while viable in culture, were unable to establish infections in the tsetse mid-gut, confirming that GPI-anchored proteins are essential for insect-parasite interactions Citation[41]. In T. brucei bloodstream forms, the double-stranded RNA interference (dsRNAi) approach was used for functional studies. In this system, TbGPI8 mRNA depletion was achieved by tetracycline induction of dual T7 promoters driving the production of dsRNA. GPI8-depleted bloodstream forms of T. brucei showed an accumulation of precursor GPIs, unanchored VSG and severe growth deficiency associated with multiple nuclei and kinetoplasts, indicative of cytokinesis arrest. Similar growth arrest phenotype was observed in bloodstream form of T. brucei mutants defective in other GPI-biosynthesis genes (e.g., GPI10 Citation[40]). These results indicate that GPIs are essential for the viability of bloodstream-form trypanosomes and imply that the GPI pathway is a valid drug target in T. brucei Citation[27], Citation[41].
A search of public genomic databases revealed that GPI8 homologues exist in apicomplexan parasites (Plasmodium falciparum, Toxoplasma gondii), plants (Arapidopsis thalania), and other eukaryotes (Caenorhabditis elegans, Drosophila melanogaster) Citation[97]. These genes have not, as yet, been functionally characterized. Further investigations of their activity will likely increase our understanding of the protein-GPI anchoring mechanism, substrate specificity as well as the overall function and essentiality of GPI-anchored proteins in other organisms.
GPI8 protein: its role in protein-GPI anchoring reaction
The yeast and human GPI8s contain a hydrophobic N-terminal signal sequence, a luminally-oriented middle segment, and a C-terminal transmembrane sequence Citation[45], Citation[90]. The L. mexicana, T. brucei and T. cruzi GPI8s exhibit ∼31% sequence homology to yeast and human GPI8s. An N-terminal ER targeting signal sequence is present; however, protozoan GPI8s lack the predicted C-terminal transmembrane helical domain found in yeast and human GPI8s. The comparative features of GPI8s in different organisms are summarized in . These protein predictions suggest that GPI8s may comprise two general groups: (1) type I transmembrane proteins, e.g., yeast and human GPI8s, and (2) soluble proteins, e.g., L. mexicana, T. brucei and T. cruzi GPI8s.
Table I. Characteristics of GPI8s.
On the basis of amino acid homology and motifs, GPI8s are classified among the CD clan of cysteine proteases, and are further subdivided into the C13 family, based upon their mechanism of peptide bond hydrolysis Citation[98–100]. This family includes peptidases found in legumes Citation[101–104], mammals Citation[105], Citation[106] and nematodes, e.g., Schistosoma mansoni Citation[107]. The asparaginyl endopeptidases Citation[108], originally identified in leguminous plants, and so named legumains, have been shown to participate in the proteolytic processing of concanavalin A and of other pro-proteins present in their seeds Citation[109–111]. More recently, mammalian homologues of legumain have been identified in lysosomes Citation[105], Citation[106] and may function in the proteolytic processing of bacterial antigens, and possibly, of pro-enzymes Citation[112]. A group of homologues in Schistosoma species, cathepsin B, D, and L and hemoglobinase, cooperate in the degradation of hemoglobin Citation[107]. The presence of conserved residues in the predicted active site of these proteases dictates their catalytic activity (). Endoproteolysis, the cleavage of a protein within the peptide chain, by cysteine proteases is believed to occur via general acid: base catalysis. In this mechanism, the conserved histidine (His) and cysteine (Cys) residues defining the catalytic diad of the active site of C13 peptidases function in concert to cleave the peptide bond. First, His acts as a general base (proton acceptor), deprotonating the sulfhydryl group of cysteine protease. Secondly, the sulfur of the Cys's thiol residue mounts a nucleophilic attack on the carbonyl carbon of the peptide substrate Citation[98]. The transfer of acyl from the cysteine protease to a water molecule, which serves as a nucleophile, generates the free acid on the cleaved peptide. In the mechanism proposed for GPI-protein anchoring, the transfer of an acyl group from the thio-carbonyl to the nucleophilic amine of the GPI's ethanolamine results in the formation of an amide bond between the protein and GPI and the release of the free C-terminal signal sequence Citation[87].
Figure 3. The postulated catalytic mechanism for GPI-anchor attachment by GPI8. Cleavage of the C-terminal signal sequence of the precursor protein is believed to occur in an endoproteolytic reaction in which the peptide bond is cleaved between the ω and ω + 1 site within the protein chain, followed by GPI attachment to the protein in an amidation reaction. Cysteine proteases (enzyme, Enz) perform peptide bond hydrolysis via a general acid: base catalysis, as follows: (1) The sulfhydryl (SH) group of the cysteine (Cys) residue is deprotonated by the histidine (His) residue, which acts as a ‘general base’ or proton acceptor; (2) Nucleophilic attack of the Cys's sulfur on the carbonyl carbon results in the cleavage of the peptide and the formation of an acyl-enzyme intermediate. The transfer of acyl to water (H2O) that serves as a nucleophile releases the Enz and formation of carboxylic acid on the cleaved peptide. When GPI serves as the nucleophile, an amide bond (*) is formed between the nitrogen in the ethanolamine phosphate (EtNP) of the GPI and the carbonyl group of the protein, completing the attachment of a GPI to the protein. Alkyl groups of the protein are represented as R; R1 corresponds to the portion of the precursor protein that is GPI-anchored; R2 corresponds to the peptide or, in the case of protein-GPI anchoring, the C-terminal signal sequence that is released. (Diagram created in ChemSketch 5.0, Advanced Chemistry Development Inc.).
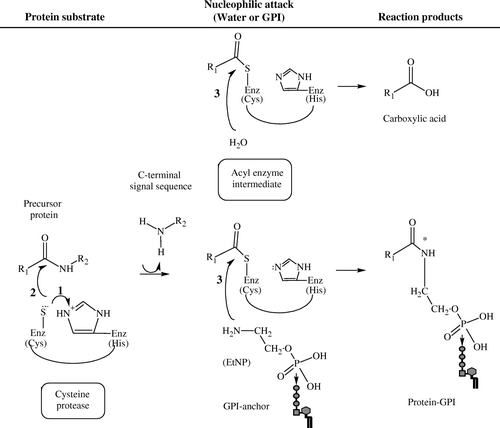
The enzymatic properties of GPI8 were confirmed in the reporter protein-GPI anchoring assay systems. GPI anchoring was lost in T. brucei membranes depleted of soluble ER contents by incubation under high pH conditions Citation[88]. Reconstitution of T. brucei RMs with recombinant LmGPI8 restored the production of VSG-GPI. Upon incubation with hydrazide, reconstituted RMs produced VSG-hydrazide. These results suggested that TbGPI8 was a soluble protein and LmGPI8 functions as a homologue of TbGPI8 to perform protein-GPI anchoring. Further, this activity was sensitive to treatment of either recombinant LmGPI8 or of T. brucei RMs with iodoacetamide, indicative of the importance of disulfide bond formation or of catalysis by sulfhydryl residue(s) in the anchoring reaction Citation[88]. Others showed the physical association between mammalian GPI8 and precursor protein to be anchored by GPI. In vitro translation of a prepro-mini-PLAP variant in mammalian RMs in the presence of N ε-(5-azido-2-nitrobenzoyl)-Lys-tRNA resulted in the incorporation of photo-reactive lysine residues. Upon photolysis, the pro-mini-PLAP cross-linked to ER proteins in its immediate vicinity. The demonstration of a photo-adduct between recombinant epitope-tagged (FLAG)-GPI8 and pro-PLAP provided the first direct evidence of an interaction between a precursor protein substrate and the GPI8 Citation[113]. A similar conclusion that GPI8 associated with mini-PLAP was obtained using semi-permeabilized cells treated with the cross-linking agent bismaleimidohexane. While this assay resulted in the cross-linking of PLAP to GPI8 in the parental K562 line, no association was detected in GPI8-deficient class K mutants, providing further strength for the evidence that GPI8 is a necessary component of the protein-GPI anchoring reaction Citation[114]. Finally, enzymatic cleavage of a fluorogenic peptide, acetyl-S-V-L-N-aminomethyl-coumarine, that mimics GPI anchor addition signal sequence by T. brucei lysates and recombinant TbGPI8 provided evidence that GPI8 is indeed directly involved in the proteolytic processing of the GPI addition signal sequence Citation[115].
Taken together, the biochemical studies performed to-date have established that GPI8 catalyzes the endoproteolysis and amidation steps involved in transfer of GPI to proteins. The two steps most likely occur in concert as a transamidation reaction, leading to the suggestion that GPI8-encoded protein is a transamidase Citation[76]. However, in vivo evidence of GPI8 transamidase activity awaits future studies.
Active-site studies of GPI8
At present, no 3D structure has been constructed for any member of the C13 family to facilitate a molecular model for understanding the catalytic and other domains of GPI8. Alternatively, functional studies of purified enzymes as well as site-directed mutagenesis of conserved residues in the putative active site have been used to characterize this group of cysteine proteases. Mutation of the conserved Cys and His residues in legumain resulted in a loss of protease activity, establishing them as critical to the active site of this protein Citation[101]. The role of GPI8 in protein-GPI anchoring based upon mutation of putative active-site residues has been examined for yGPI8 Citation[116], hGPI8 Citation[117] and LmGPI8 Citation[96]. Among potential active-site residues present in yGPI8, two histidine and two cysteine residues were conserved in a majority of C13 family members, including the GPI8s. The experimental studies demonstrating the functional importance of the residues, highlighted in , in GPI anchoring are detailed below.
Figure 4. CLUSTAL W (1.82) multiple sequence alignment of GPI8 in T. cruzi, T. brucei, L. mexicana, S. cerevisiae, H. sapiens, T. gondii and P. falciparum and of legumain in Canavalia ensiformis. Accession numbers: TbGPI8, AJ308106; LmGPI8, AJ242865; yGPI8, P49018; hGPI8, Q92643; TgGPI8, AJ507036; PfGPI8, AJ401201; Legumain, JX0344. Arrows indicate the conserved cysteine (C) and histidine (H) residues. The postulated active site residues for GPI8, based on mutagenesis studies of legumain (plant endopeptidase), are indicated by •. Specific residues that have been examined by mutagenesis and overexpression in the respective organisms are underlined.
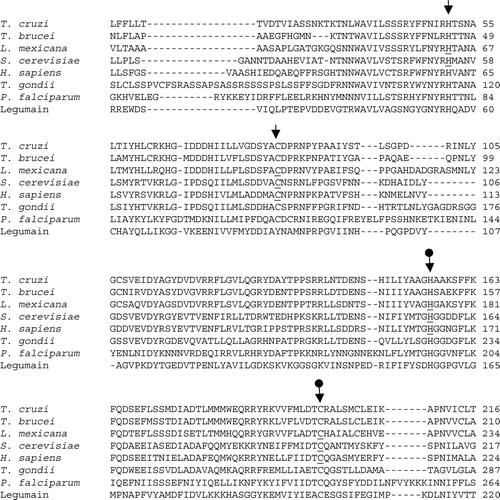
Two approaches were applied to examine the potential active-site histidine (H) and cysteine (C) residues in yGPI8 Citation[116]. The conserved residues in yGPI8 were individually mutated to alanine (A), and these alleles were investigated for their ability to: (i) restore viability and/or protein-GPI attachment in conditional, ts ygpi8 mutants or yGPI8 deletion mutants (yΔGPI8), or (ii) produce dominant-negative phenotypic effects on wild-type (parental) yeast strains, e.g., growth impairment, lethality, and/or GPI-anchoring defects Citation[90]. Using the first approach, expression of the yGpi8C199A and yGpi8H57A did not re-establish the viability of yΔGPI8 mutants or growth of the ts ygpi8 mutants at 37°C. Using the second approach, expression of these alleles resulted in an accumulation of the precursor GPI lipid CP2, delayed maturation of GAS1 protein, and arrested cell growth. yGpi8C85A and yGpi8H54A complemented the growth defect of yΔGPI8 Citation[116].
The corresponding conserved amino acids, Cys92, His164, and Cys206, were investigated as potential active-site residues in hGPI8. The expression of hGpi8H164A and hGpi8C206A did not functionally complement the class K mutants to restore the protein transfer of GPIs and cell surface expression of CD59, unlike hGpi8C92A. The in vitro PLAP assay demonstrated that hGpi8H164A and hGpi8C206A alleles were unable to process pro-mini-PLAP to GPI- or hydrazide-linked forms, unlike in class K mutant cells complemented with hGPI8 Citation[117].
Similar to yeast and mammalian cells, putative active-site residues are conserved in Leishmania, T. brucei, and T. cruzi. L. mexicana null mutants (LmΔGPI8) transfected with LmGPI8 or LmGpi8H63A exhibited decreased secretion and increased cell surface expression of GP63, whereas cells complemented with LmGpi8H174A or LmGpi8C216G secreted high levels of GP63. Similar observations were made when LmGpi8C216G allele was expressed in wild-type L. mexicana; with increased concentrations of selective drug, surface expression of GP63 was diminished in a dose-dependent fashion. The LmΔGPI8/LmGpi8C94G parasites exhibited an intermediate phenotype, with GP63 being processed and transported to the cell surface as well as secreted into the medium, suggesting that Cys94 residue may be necessary for optimal folding of the GPI8 and/or is directly involved in its interaction with other proteins of the complex Citation[27], Citation[96]. Mutation of His54 in yGPI8 Citation[116] and Cys92 in hGPI8 Citation[117] also led to a partial loss of protein-GPI anchoring activity, suggesting that these residues directly or indirectly affect protein-protein interactions.
Other components of protein-GPI anchoring machinery
While GPI8 is the catalytic component involved in protein-GPI anchoring reaction, it functions in cooperation with other essential proteins as a multi-subunit complex, named the transamidase (TAM) complex. Genetic and biochemical approaches have identified, in addition to GPI8, two protein subunits of this complex, namely GAA1 and GPI16/PIG-T, which are common to all eukaryotic organisms that have been studied to date. GPI17/PIG-S and CDC91/PIG-U are detected in yeast and mammalian TAM, but are absent from trypanosomatids, which, alternatively, possess TTA1 and TTA2. PIG-T is a type I transmembrane protein, and GAA1, GPI17/PIG-S and CDC91/PIG-U have multiple transmembrane domains (). We discuss the characteristics of the TAM components in yeast, mammalian cells and trypanosomes.
Figure 5. The transamidase (TAM) complex. A model of GPI8 interaction with other proteins in the TAM complex is presented for (A) yeast and human cells, and (B) T. brucei. Structures are not drawn to scale. Identification and functional analysis of the indicated proteins is described in the text Citation[124]. Adapted by permission from Macmillan Publishers Ltd: EMBO Journal 20(15):4088–4098, copyright 2001.
![Figure 5. The transamidase (TAM) complex. A model of GPI8 interaction with other proteins in the TAM complex is presented for (A) yeast and human cells, and (B) T. brucei. Structures are not drawn to scale. Identification and functional analysis of the indicated proteins is described in the text Citation[124]. Adapted by permission from Macmillan Publishers Ltd: EMBO Journal 20(15):4088–4098, copyright 2001.](/cms/asset/53d4edaa-989c-4a91-9723-09da78e0ccdf/imbc_a_160132_f0005_b.gif)
Table II. Other components of GPI-anchoring machinery.
GAA1
In yeast, functional complementation of the GPI-anchoring deficient end2 mutant with a cDNA expression library which resulted in restoration of GAS1 maturation and anchoring to GPI, led to the identification of yGAA1, named for its role in GPI-anchor attachment Citation[93]. GAA1s in other organisms were identified based upon homology to yGAA1. Human GAA1 is 25% identical and 57% homologous to yeast protein and 21% identical and 38% similar to T. brucei GAA1. In yeast and human cells, GAA1 is predicted to encode a ∼68-kDa protein, with an uncleaved, cytoplasmically oriented N-terminal signal sequence, and a large, hydrophilic, lumenal domain, followed by several transmembrane domains (six in yeast and seven in human cells). A C-terminal KEKQS ER retention sequence and two putative, N-linked glycosylation sequences (one confirmed by site-directed mutagenesis/endoglycosidase treatment) are identified in yGAA1. Immunofluorescence staining showed co-localization of GAA1 with an authentic ER resident protein WBP1. Targeted disruption of yGAA1 resulted in a lethal phenotype associated with accumulation of GPI precursor CP2 and GAS1 protein; this phenotype was rescued by episomal expression of GAA1 Citation[93].
In mammalian cells, over-expression of anti-sense GAA1 or disruption of GAA1 resulted in decreased expression of the GPI-anchored reporter proteins (CD8-DAF and Thy-1) and an accumulation of mature GPI anchors Citation[117], leading to the conclusion that protein and GPI biosynthesis are not altered by GAA1 deficiency. Microsomal membranes of GAA1-defective cells were capable of cleaving the N-terminal signal peptide to form pro-mini-PLAP. However, the mini-PLAP-GPI or the mini-PLAP-hydrazide forms were not detected Citation[118], Citation[119], a phenomenon characteristic of defects in protein-GPI anchoring activity.
The T. brucei GAA1 is noted to encode a smaller (461 amino acids) protein, as compared to human and yeast GAA1 (621 amino acid) proteins. However, like human and yeast GAA1s, TbGAA1 contains several transmembrane domains. Disruption of TbGAA1 resulted in a loss of cell surface expression of the major GPI-anchored protein, procyclin, which was restored upon the episomal expression of TbGAA1 Citation[120]. Together, these results provide evidence that GAA1 plays an essential role in the anchoring of proteins to GPI in all organisms studied to date.
GAA1 is not homologous to any known proteins in the global databases to suggest its specific function. In effort to characterize the functional role of GAA1 in protein-GPI anchoring machinery, recombinant FLAG-GAA1 was co-expressed with GST-GPI8 in CHO cells. Co-precipitation of the FLAG-GAA1 and GST-GPI8 by either of the epitope-specific antibodies suggested the interaction of these proteins. A similar observation was made in TbΔGAA1 mutants expressing FLAG-GAA1; GPI8, TTA1, and GAA1 were co-precipitated by anti-FLAG antibody, thus providing evidence for the association of GAA1 with other components of the TAM complex in trypanosomes Citation[120]. To determine which segment of GAA1 is essential for this interaction, a truncated Gaa1 lacking the C-terminal transmembrane segment was expressed in ΔGAA1 CHO cells. Truncated Gaa1 interacted with other subunits (GPI8, PIG-S and PIG-T) and a pro-protein substrate, but failed to co-precipitate a GPI precursor and formed a non-functional TAM complex. In subsequent experiments, a large lumenal domain between 1st and 2nd transmembrane domains was shown to be sufficient for GAA1 interaction with TAM subunits Citation[121]. A proline residue in the C-terminal transmembrane domain of GAA1 was identified to serve as a dynamic hinge, providing a structural basis for GPI interaction with TAM Citation[122], and leading to the suggestion that GAA1 may play a role in recruiting GPI to the TAM complex. In vivo demonstration of this GAA1 interaction has not as yet been published.
GPI16/PIG-T and GPI17/PIG-S
To identify additional components of the yeast TAM complex, Fraering et al. Citation[123] expressed GST-tagged yGPI8 in ΔGPI8 yeast mutants. The multi-protein complex containing yGPI8-GST was extracted from microsomal membranes in digitonin, purified via GST-sepharose affinity chromatography and resolved by two-dimensional, blue-native gel electrophoresis. Mass spectrophotometric analysis of tryptic digests of the proteins co-precipitated with yGPI8 identified GAA1, GPI16 (earlier named YHR188c) and GPI17 Citation[123]. A similar approach led to the identification of PIG-S and PIG-T in class K mutant cells expressing FLAG-GST-hGPI8. The two proteins were co-precipitated with the TAM complex, and later identified to be homologues of GPI17 and GPI16, respectively Citation[124].
GPI16 is predicted to encode a type I membrane protein with two N-glycosylation sites, an N-terminal ER targeting signal sequence, large lumenal sequence, and a single C-terminal transmembrane domain. GPI16 homologues identified in several organisms predict a similar size (531–639 amino acids) and a similar hydrophobicity profile. Only the yGPI16s have a small C-terminal cytosolic extension with an ER retrieval motif, but the proteins of other species may be retained in the ER by other signals or interaction with GAA1 or GPI8. GPI17 and its mammalian homologue PIG-S encode N-glycosylated protein (534–555 amino acids) with a cytoplasmically oriented N-terminal hydrophobic signal sequence, and a large luminal domain. A putative transmembrane domain is predicted at the C-terminus of PIG-S and PIG-T proteins.
Traditional biochemical and molecular approaches were employed for functional characterization of GPI16 and GPI17. Triton X-100 treatment of yeast microsomal membranes released GPI16 in soluble fractions. The sub-cellular fractionation of GPI16 with ER proteins (GPI8 and WBP1), sensitivity of GPI16 to protease-mediated digestion in detergent-treated yeast microsomes, and a decrease in molecular mass by endoglycosidase H treatment provide evidence for GPI16 being an integral ER membrane glycoprotein, with the bulk of the protein oriented toward the lumen of the ER. Similar biochemical approaches concluded GPI17 (∼63-kDa) is an N-glycosylated integral membrane glycoprotein with its N-terminal hydrophobic signal sequence not cleaved. Depletion of either GPI16 or GPI17 resulted in an accumulation of precursor GPI lipid CP2 and unanchored pro-proteins (e.g., GAS1 and CWP1) Citation[123], Citation[125], a phenomenon characteristic of GPI-anchoring-deficient yeast mutants. Deletion of GPI16 or GPI17 was lethal Citation[126]. Both GPI16 and GPI17 were essential for GPI8 stability. While GPI16 was unstable in absence of GPI8, GPI17 was destabilized upon GAA1 depletion Citation[123].
Like yeast, deletion of PIG-S or PIG-T in F9 embryonal carcinoma cells resulted in a loss of cell surface expression of the GPI-anchored protein Thy-1, which was restored by the episomal expression of the respective gene. ΔPIG-S and ΔPIG-T microsomes were unable to process pro-mini-PLAP, i.e., they failed to develop the GPI-anchored or hydrazide-protein forms, and accumulated mature GPIs. Co-immunoprecipitation experiments suggested that PIG-S is a stable subunit of the TAM complex, independent of the expression levels of the other components, while PIG-T was critical for stable expression and association with GAA1 and hGPI8 Citation[124]. Others provided direct evidence of a disulfide linkage between PIG-T and GPI8; GST-tagged cysteine-to-serine (S) mutants of GPI8, mutated in conserved Cys92 and Cys206 (active-site residues) were expressed in GPI8-deficient class K mutant cells. Immunoprecipitation under native conditions, followed by western blotting, identified that GPI8C92S mutant was incapable of interacting with PIG-T. Similarly, PIG-TC182S, but not PIG-TC139S, mutant failed to interact with GPI8, leading to a conclusion that Cys92 of GPI8 and Cys182 of PIG-T are involved in the formation of the disulfide bond. Notably disulfide linkage was not essential for the generation of the tetrameric complex, since the PIG-TC182S or GPI8C92S were co-precipitated with other components of the TAM complex. However, PIG-TC182S and GPI8C92S alleles only partially restored the surface expression of GPI-anchored proteins in the respective deletion mutant (ΔPIG-T and ΔGPI8) cells. In microsomal membranes of the ΔPIG-T/ PIG-TC182S and ΔGPI8/GPI8C92S cells, only trace amounts of GPI-anchoring activity assessed by a mini-PLAP assay was restored. These studies concluded that the disulfide linkage between GPI8 and PIG-T is important, but not essential, for normal GPI transfer activity Citation[127].
Despite identification of GPI17 in affinity-purified TAM complexes Citation[123], others have shown that a majority of GPI17 is not associated with a yeast TAM complex Citation[125]. No stable stochiometric interaction of GPI17 with the GPI8-GAA1-GPI16 complex was noted in yeast detergent extracts, leading to the suggestion that GPI17 may exert its GPI anchoring role without interacting in a consistent manner with other TAM components Citation[125]. A similar conclusion was reached in F9 cells, as in the absence of PIG-S, a stable GPI8-GAA1-PIG-T complex is formed Citation[124].
In trypanosomes, immunoprecipitation with anti-FLAG antibody from TbGPI8-FLAG-expressing parasites enabled identification of a partial amino-acid sequence of a ∼70 kDa protein that was 13% identical and 25% similar to GPI16 Citation[120]. No functional analysis of TbGP16 has been reported to date.
CDC91/PIG-U
Yeast CDC91 (394 amino acids) exhibits 28% identity to PIG-U of mammalian cells, but has not yet been functionally characterized. PIG-U was identified by a novel selection approach. Chemically mutagenized CHO cells were isolated by their resistance to cytolysis by aerolysin, a GPI-anchored protein-binding toxin. One aerolysin-resistant clonal line exhibited reduced surface staining with fluorescent aerolysin and with antibodies to DAF and CD59 proteins. Microsomes isolated from this CHO line, though capable of synthesizing mature GPIs, were unable to form GPI-anchored mini-PLAP. Transfection of hGPI8, hGAA1, PIG-S and PIG-T did not restore GPI anchoring in this CHO mutant line. These results indicated that this mutant was defective in a new TAM complex gene, which was designated class U. Transfection of the class U mutant with a rat cDNA expression library, followed by restoration of aerolysin sensitivity and DAF surface expression, led to the identification of the complementing gene, thereby named PIG-U Citation[128].
PIG-U encodes a highly hydrophobic protein (435 amino acids) with nine potential transmembrane domains. A sequence of 21 amino acids spanning residues 269–289 in PIG-U is highly similar to that in a corresponding region in CDC91 and consists of a 17-residue motif present in fatty acid elongases. Transfection of PIG-U in class U mutants restored the cell surface expression of the GPI-anchored proteins CD59 and DAF. Subsequently, co-immunoprecipitation experiments showed that FLAG-tagged PIG-U associates with other TAM subunits. PIG-U is not essential for the formation of the TAM complex, as the high-molecular weight complexes of GPI8, GAA1, PIG-T and PIG-S were purified from class U mutants. However, the PIG-U-deficient TAM complex is non-functional and lacks protein-GPI anchoring activity Citation[128].
Yeast CDC91, like PIG-U, retains the predicted characteristics of a highly hydrophobic protein with multiple transmembrane domains. Transfection of CDC91 into class U mutants partially restored protein-GPI anchoring, suggesting that it is a functional homologue Citation[128]. When the conserved aromatic amino acids in the elongase motif were mutated to leucine, the mutant recombinant protein failed to restore protein-GPI in PIG-U mutants. It was postulated, but not demonstrated experimentally, that PIG-U is involved in the recognition of long-chain fatty acids in GPI Citation[128].
TTA1 and TTA2
T. brucei procyclics expressing GPI8-FLAG-GST were subjected to Nonidet P-40 treatment, and affinity-purified complexes containing GPI8 were resolved by SDS-PAGE. N-terminal sequencing of the co-purified 40- and 35-kDa proteins led to the identification of two new subunits of the TAM complex, named trypanosomatid transamidase 1 (TTA1) and 2 (TTA2), respectively. TTA1 and TTA2 sequences were identified in the T. brucei genome, and the predicted proteins contained 2 and 6 transmembrane domains, respectively. Targeted disruption of TTA1 or TTA2 in T. brucei procyclic forms resulted in a loss of the surface expression of the GPI-anchored protein, procyclin, and slower growth, which was normalized by transfection of corresponding cDNA Citation[120]. The biosynthesis of GPI precursors was not affected in ΔTTA1 and ΔTTA2 mutants. These studies provide evidence that TTA1 and TTA2 are essential components of the T. brucei TAM complex Citation[120].
TTA1 and TTA2 have no significant sequence homology to PIG-S and PIG-U or any other proteins of known function. Nevertheless, hydrophobicity profiles and membrane orientations of TTA1 and TTA2 are similar to those of PIG-S and PIG-U, respectively Citation[120], Citation[124], Citation[128]. Given that between mammalian and T. brucei cells, the GPI-addition signal sequence of the precursor proteins are not interchangeable and the structures of the precursor GPIs are different in the glycan and inositolphospholipid parts, it is likely that the TAM components that recognize the GPI-addition signal sequence, C/A site, and the precursor GPI in T. brucei are different from those in mammalian cells. Whether TTA1 and TTA2, the two different components of T. brucei TAM complex, are involved in such functions would hopefully be addressed in future studies.
With the publication of the complete genome sequences of L. major, T. brucei and T. cruzi on 15 July 2005 Citation[129–132], other gene homologues of the described TAM complex, e.g., GAA1 in T. cruzi, can now be identified by searching the public databases. We anticipate that this information will facilitate additional genetic or functional studies to evaluate the importance of these published proteins in the TAM mechanism of the respective organisms.
Conclusion
The collection of genetic and biochemical studies presented in this review suggest that GPI8 is responsible for anchoring GPIs to proteins and that GPI8 functions in association with other proteins in the TAM complex. Much is to be learned about the biochemical and physical interaction among the multiple components of the TAM complex, their exact function, and the requirement for such a large complex for GPI-protein anchoring. Nevertheless, substantial differences are noted in substrate specificity and function of the subunits of the TAM complexes of mammalian cells and parasitic trypanosomes. Further, trypanosomes are highly dependent upon protein-GPI anchoring machinery for their survival and virulence in mammalian host. Future studies are, thus, likely to exploit these differences for the development of novel therapeutics against parasitic diseases.
The work in NG's laboratory has been supported, in part, by grants from the American Heart Association, John Sealy Memorial Endowment Fund for Biomedical Research, American Health Assistance Foundation, and National Institutes of Health (AI053098-01, AI054578-01). MZ was awarded the James W. McLaughlin Predoctoral Fellowship at the University of Texas Medical Branch and has been awarded an appointment as a Postdoctoral Research Fellow in the Emerging Infectious Diseases (EID) Fellowship Program administered by the Association of Public Health Laboratories (APHL) and funded by the Centers for Disease Control and Prevention (CDC). MZ thanks Drs Anant K. Menon and Paul J. Brindley for valuable critical input and discussions. Our thanks are due to Mardelle Susman for proofreading and editing of the manuscript.
References
- Ferguson MAJ. What can GPI do for you?. Parasitol Today 1994; 10: 48–52
- Ferguson MA. Colworth Memorial Lecture. Glycosyl-phosphatidyliniositol membrane anchors: the tale of a tail. Biochem Soc Transact 1992; 20: 243–256
- Ferguson MA. The structure, biosynthesis and functions of glycosylphosphatidylinositol anchors, and the contributions of trypanosome research. J Cell Sci 1999; 112: 2799–2809
- McConville MJ, Ferguson MA. The structure, biosynthesis and function of glycosylated phosphatidylinositols in the parasitic protozoa and higher eukaryotes. Biochem J 1993; 294: 305–324
- Fankhauser C, Homans SW, Thomas-Oates JE, McConville MJ, Desponds C, Conzelmann A, Ferguson MA. Structures of glycosylphosphatidylinositol membrane anchors from Saccharomyces cerevisiae. J Biol Chem 1993; 268: 26365–26374
- Ferguson MA. The surface glycoconjugates of trypanosomatid parasites. Philos Trans R Soc Lond B Biol Sci 1997; 352: 1295–1302
- Conzelmann A, Fankhauser C, Puoti A, Desponds C. Biosynthesis of glycophosphoinositol anchors in Saccharomyces cerevisiae. Cell Biol Int Rep 1991; 15: 863–873
- de Lederkremer RM. Free and protein-linked glycoinositolphospholipids in Trypanosoma cruzi. Braz J Med Biol Res 1994; 27: 239–242
- Schofield L, Tachado SD. Regulation of host cell function by glycosylphosphatidylinositols of the parasitic protozoa. Immunol Cell Biol 1996; 74: 555–563
- Nosjean O, Briolay A, Roux B. Mammalian GPI proteins: sorting, membrane residence and functions. Biochim Biophys Acta 1997; 1331: 153–186
- Sacks DL, Modi G, Rowton E, Spath G, Epstein L, Turco SJ, Beverley SM. The role of phosphoglycans in Leishmania-sand fly interactions. Proc Natl Acad Sci USA 2000; 97: 406–411
- Spath GF, Epstein L, Leader B, Singer SM, Avila HA, Turco SJ, Beverley SM. Lipophosphoglycan is a virulence factor distinct from related glycoconjugates in the protozoan parasite Leishmania major. Proc Natl Acad Sci USA 2000; 97: 9258–9263
- Ropert C, Gazzinelli RT. Signaling of immune system cells by glycosylphosphatidylinositol (GPI) anchor and related structures derived from parasitic protozoa. Curr Opin Microbiol 2000; 3: 395–403
- Turco SJ, Spath GF, Beverley SM. Is lipophosphoglycan a virulence factor? A surprising diversity between Leishmania species. Trends Parasitol 2001; 17: 223–226
- Davidson EA, Gowda DC. Glycobiology of Plasmodium falciparum. Biochimie 2001; 83: 601–604
- Lekutis C, Ferguson DJ, Grigg ME, Camps M, Boothroyd JC. Surface antigens of Toxoplasma gondii: variations on a theme. Int J Parasitol 2001; 31: 1285–1292
- McConville MJ, Mullin KA, Ilgoutz SC, Teasdale RD. Secretory pathway of trypanosomatid parasites. Microbiol Mol Biol Rev 2002; 66: 122–154
- Mensa-Wilmot K, LeBowitz JH, Chang KP, Al-Qahtani A, McGwire BS, Tucker S, Morris JC. A glycosylphosphatidylinositol (GPI)-negative phenotype produced in Leishmania major by GPI phospholipase C from Trypanosoma brucei: topography of two GPI pathways. J Cell Biol 1994; 124: 935–947
- Garg N, Postan M, Mensa-Wilmot K, Tarleton RL. Glycosylphosphatidylinositols are required for the development of Trypanosoma cruzi amastigotes. Infect Immun 1997; 65: 4055–4060
- Mensa-Wilmot K, Garg N, McGwire BS, Lu HG, Zhong L, Armah DA, LeBowitz JH, Chang KP. Roles of free GPIs in amastigotes of Leishmania. Mol Biochem Parasitol 1999; 99: 103–116
- Butikofer P, Boschung M, Brodbeck U, Menon AK. Phosphatidylinositol hydrolysis by Trypanosoma brucei glycosylphosphatidylinositol phospholipase C. J Biol Chem 1996; 271: 15533–15541
- Toker A. Phosphoinositides and signal transduction. Cell Mol Life Sci 2002; 59: 761–779
- Martelli AM, Follo MY, Evangelisti C, Fala F, Fiume R, Billi AM, Cocco L. Nuclear inositol lipid metabolism: more than just second messenger generation?. J Cell Biochem 2005; 96: 285–292
- Martelli AM, Fiume R, Faenza I, Tabellini G, Evangelista C, Bortul R, Follo MY, Fala F, Cocco L. Nuclear phosphoinositide specific phospholipase C (PI-PLC)-beta1: A central intermediary in nuclear lipid-dependent signal transduction. Histol Histopathol 2005; 20: 1251–1260
- Joshi PB, Sacks DL, Modi G, McMaster WR. Targeted gene deletion of Leishmania major genes encoding developmental stage-specific leishmanolysin (GP63). Mol Microbiol 1998; 27: 519–530
- Ilgoutz SC, Zawadzki JL, Ralton JE, McConville MJ. Evidence that free GPI glycolipids are essential for growth of Leishmania mexicana. Embo J 1999; 18: 2746–2755
- Hilley JD, Zawadzki JL, McConville MJ, Coombs GH, Mottram JC. Leishmania mexicana mutants lacking glycosylphosphatidylinositol (GPI): protein transamidase provide insights into the biosynthesis and functions of GPI-anchored proteins. Mol Biol Cell 2000; 11: 1183–1195
- Joshi PB, Kelly BL, Kamhawi S, Sacks DL, McMaster WR. Targeted gene deletion in Leishmania major identifies leishmanolysin (GP63) as a virulence factor. Mol Biochem Parasitol 2002; 120: 33–40
- King DL, Turco SJ. A ricin agglutinin-resistant clone of Leishmania donovani deficient in lipophosphoglycan. Mol Biochem Parasitol 1988; 28: 285–293
- Cappai R, Morris L, Aebischer T, Bacic A, Curtis JM, Kelleher M, McLeod KS, Moody SF, Osborn AH, Handman E. Ricin-resistant mutants of Leishmania major which express modified lipophosphoglycan remain infective for mice. Parasitology 1994; 108: 397–405
- Elhay M, Kelleher M, Bacic A, McConville MJ, Tolson DL, Pearson TW, Handman E. Lipophosphoglycan expression and virulence in ricin-resistant variants of Leishmania major. Mol Biochem Parasitol 1990; 40: 255–267
- Ilg T. Lipophosphoglycan is not required for infection of macrophages or mice by Leishmania mexicana. Embo J 2000; 19: 1953–1962
- Ilg T, Demar M, Harbecke D. Phosphoglycan repeat-deficient Leishmania mexicana parasites remain infectious to macrophages and mice. J Biol Chem 2001; 276: 4988–4997
- Spath GF, Lye LF, Segawa H, Sacks DL, Turco SJ, Beverley SM. Persistence without pathology in phosphoglycan-deficient Leishmania major. Science 2003; 301: 1241–1243
- Spath GF, Lye LF, Segawa H, Turco SJ, Beverley SM. Identification of a compensatory mutant (lpg2-REV) of Leishmania major able to survive as amastigotes within macrophages without LPG2-dependent glycoconjugates and its significance to virulence and immunization strategies. Infect Immun 2004; 72: 3622–3627
- Dermine JF, Scianimanico S, Prive C, Descoteaux A, Desjardins M. Leishmania promastigotes require lipophosphoglycan to actively modulate the fusion properties of phagosomes at an early step of phagocytosis. Cell Microbiol 2000; 2: 115–126
- Naderer T, McConville MJ. Characterization of a Leishmania mexicana mutant defective in synthesis of free and protein-linked GPI glycolipids. Mol Biochem Parasitol 2002; 125: 147–161
- Garami A, Ilg T. The role of phosphomannose isomerase in Leishmania mexicana glycoconjugate synthesis and virulence. J Biol Chem 2001; 276: 6566–6575
- Zufferey R, Allen S, Barron T, Sullivan DR, Denny PW, Almeida IC, Smith DF, Turco SJ, Ferguson MA, Beverley SM. Ether phospholipids and glycosylinositolphospholipids are not required for amastigote virulence or for inhibition of macrophage activation by Leishmania major. J Biol Chem 2003; 278: 44708–44718
- Nagamune K, Nozaki T, Maeda Y, Ohishi K, Fukuma T, Hara T, Schwarz RT, Sutterlin C, Brun R, Riezman H, Kinoshita T. Critical roles of glycosylphosphatidylinositol for Trypanosoma brucei. Proc Natl Acad Sci USA 2000; 97: 10336–10341
- Lillico S, Field MC, Blundell P, Coombs GH, Mottram JC. Essential roles for GPI-anchored proteins in African trypanosomes revealed using mutants deficient in GPI8. Mol Biol Cell 2003; 14: 1182–1194
- Hirose S, Mohney RP, Mutka SC, Ravi L, Singleton DR, Perry G, Tartakoff AM, Medof ME. Derivation and characterization of glycoinositol-phospholipid anchor-defective human K562 cell clones. J Biol Chem 1992; 267: 5272–5278
- Mohney RP, Knez JJ, Ravi L, Sevlever D, Rosenberry TL, Hirose S, Medof ME. Glycoinositol phospholipid anchor-defective K562 mutants with biochemical lesions distinct from those in Thy-1- murine lymphoma mutants. J Biol Chem 1994; 269: 6536–6542
- Chen R, Udenfriend S, Prince GM, Maxwell SE, Ramalingam S, Gerber LD, Knez J, Medof ME. A defect in glycosylphosphatidylinositol (GPI) transamidase activity in mutant K cells is responsible for their inability to display GPI surface proteins. Proc Natl Acad Sci USA 1996; 93: 2280–2284
- Yu J, Nagarajan S, Knez JJ, Udenfriend S, Chen R, Medof ME. The affected gene underlying the class K glycosylphosphatidylinositol (GPI) surface protein defect codes for the GPI transamidase. Proc Natl Acad Sci USA 1997; 94: 12580–12585
- Conzelmann A, Spiazzi A, Hyman R, Bron C. Anchoring of membrane proteins via phosphatidylinositol is deficient in two classes of Thy-1 negative mutant lymphoma cells. Embo J 1986; 5: 3291–3296
- Conzelmann A, Spiazzi A, Bron C, Hyman R. No glycolipid anchors are added to Thy-1 glycoprotein in Thy-1-negative mutant thymoma cells of four different complementation classes. Mol Cell Biol 1988; 8: 674–688
- Inoue N, Murakami Y, Kinoshita T. Molecular genetics of paroxysmal nocturnal hemoglobinuria. Int J Hematol 2003; 77: 107–112
- Kawagoe K, Kitamura D, Okabe M, Taniuchi I, Ikawa M, Watanabe T, Kinoshita T, Takeda J. Glycosylphosphatidylinositol-anchor-deficient mice: implications for clonal dominance of mutant cells in paroxysmal nocturnal hemoglobinuria. Blood 1996; 87: 3600–3606
- Tarutani M, Itami S, Okabe M, Ikawa M, Tezuka T, Yoshikawa K, Kinoshita T, Takeda J. Tissue-specific knockout of the mouse Pig-a gene reveals important roles for GPI-anchored proteins in skin development. Proc Natl Acad Sci USA 1997; 94: 7400–7405
- Hazenbos WL, Murakami Y, Nishimura J, Takeda J, Kinoshita T. Enhanced responses of glycosylphosphatidylinositol anchor-deficient T lymphocytes. J Immunol 2004; 173: 3810–3815
- Hazenbos WL, Clausen BE, Takeda J, Kinoshita T. GPI-anchor deficiency in myeloid cells causes impaired FcgammaR effector functions. Blood 2004; 104: 2825–2831
- Von Heijne G. A new method for predicting signal sequence cleavage sites. Nucleic Acids Res 1986; 14: 4683–4690
- Ng DT, Brown JD, Walter P. Signal sequences specify the targeting route to the endoplasmic reticulum membrane. J Cell Biol 1996; 134: 269–278
- Wang J, Maziarz K, Ratnam M. Recognition of the carboxyl-terminal signal for GPI modification requires translocation of its hydrophobic domain across the ER membrane. J Mol Biol 1999; 286: 1303–1310
- Udenfriend S, Kodukula K. How glycosylphosphatidylinositol-anchored membrane proteins are made. Annu Rev Biochem 1995; 64: 563–591
- Micanovic R, Gerber LD, Berger J, Kodukula K, Udenfriend S. Selectivity of the cleavage/attachment site of phosphatidylinositol-glycan-anchored membrane proteins determined by site-specific mutagenesis at Asp-484 of placental alkaline phosphatase. Proc Natl Acad Sci USA 1990; 87: 157–161
- Micanovic R, Kodukula K, Gerber LD, Udenfriend S. Selectivity at the cleavage/attachment site of phosphatidylinositol-glycan anchored membrane proteins is enzymatically determined. Proc Natl Acad Sci USA 1990; 87: 7939–7943
- Gerber LD, Kodukula K, Udenfriend S. Phosphatidylinositol glycan (PI-G) anchored membrane proteins. Amino acid requirements adjacent to the site of cleavage and PI-G attachment in the COOH-terminal signal peptide. J Biol Chem 1992; 267: 12168–12173
- Kodukula K, Gerber LD, Amthauer R, Brink L, Udenfriend S. Biosynthesis of glycosylphosphatidylinositol (GPI)-anchored membrane proteins in intact cells: specific amino acid requirements adjacent to the site of cleavage and GPI attachment. J Cell Biol 1993; 120: 657–664
- Nuoffer C, Jeno P, Conzelmann A, Riezman H. Determinants for glycophospholipid anchoring of the Saccharomyces cerevisiae GAS1 protein to the plasma membrane. Mol Cell Biol 1991; 11: 27–37
- Nuoffer C, Horvath A, Riezman H. Analysis of the sequence requirements for glycosylphosphatidylinositol anchoring of Saccharomyces cerevisiae Gas1 protein. J Biol Chem 1993; 268: 10558–10563
- Moran P, Caras IW. Fusion of sequence elements from non-anchored proteins to generate a fully functional signal for glycophosphatidylinositol membrane anchor attachment. J Cell Biol 1991; 115: 1595–1600
- Bohme U, Cross GA. Mutational analysis of the variant surface glycoprotein GPI-anchor signal sequence in Trypanosoma brucei. J Cell Sci 2002; 115: 805–816
- Furukawa Y, Tsukamoto K, Ikezawa H. Mutational analysis of the C-terminal signal peptide of bovine liver 5'-nucleotidase for GPI anchoring: a study on the significance of the hydrophilic spacer region. Biochim Biophys Acta 1997; 1328: 185–196
- Appel RD, Bairoch A, Hochstrasser DF. A new generation of information retrieval tools for biologists: the example of the ExPASy WWW server. Trends Biochem Sci 1994; 19: 258–260
- Moran P, Caras IW. Requirements for glycosylphosphatidylinositol attachment are similar but not identical in mammalian cells and parasitic protozoa. J Cell Biol 1994; 125: 333–343
- Caras IW, Moran P. The requirements for GPI-attachment are similar but not identical in mammalian cells and parasitic protozoa. Braz J Med Biol Res 1994; 27: 185–188
- Vidugiriene J, Menon AK. Early lipid intermediates in glycosyl-phosphatidylinositol anchor assembly are synthesized in the ER and located in the cytoplasmic leaflet of the ER membrane bilayer. J Cell Biol 1993; 121: 987–996
- Vidugiriene J, Menon AK. The GPI anchor of cell-surface proteins is synthesized on the cytoplasmic face of the endoplasmic reticulum. J Cell Biol 1994; 127: 333–341
- Vidugiriene J, Sharma DK, Smith TK, Baumann NA, Menon AK. Segregation of glycosylphosphatidylinositol biosynthetic reactions in a subcompartment of the endoplasmic reticulum. J Biol Chem 1999; 274: 15203–15212
- Menon AK, Watkins WE, Hrafnsdottir S. Specific proteins are required to translocate phosphatidylcholine bidirectionally across the endoplasmic reticulum. Curr Biol 2000; 10: 241–252
- Vishwakarma RA, Menon AK. Flip-flop of glycosylphosphatidylinositols (GPI's) across the ER. Chem Commun (Camb) 2005; 4: 453–455
- Vishwakarma RA, Vehring S, Mehta A, Sinha A, Pomorski T, Herrmann A, Menon AK. New fluorescent probes reveal that flippase-mediated flip-flop of phosphatidylinositol across the endoplasmic reticulum membrane does not depend on the stereochemistry of the lipid. Org Biomol Chem 2005; 3: 1275–1283
- Amthauer R, Kodukula K, Gerber L, Udenfriend S. Evidence that the putative COOH-terminal signal transamidase involved in glycosylphosphatidylinositol protein synthesis is present in the endoplasmic reticulum. Proc Natl Acad Sci USA 1993; 90: 3973–3977
- Reizman H, Conzelmann A. Glycosylphosphatidylinositol: protein transamidase. The handbook of proteolytic enzymes2nd edn, AJ Barrett, ND Rawlings, JF Woessner. Academic Press, London 1998; 756–759
- Mayor S, Menon AK, Cross GA. Transfer of glycosyl-phosphatidylinositol membrane anchors to polypeptide acceptors in a cell-free system. J Cell Biol 1991; 114: 61–71
- Kodukula K, Micanovic R, Gerber L, Tamburrini M, Brink L, Udenfriend S. Biosynthesis of phosphatidylinositol glycan-anchored membrane proteins. Design of a simple protein substrate to characterize the enzyme that cleaves the COOH-terminal signal peptide. J Biol Chem 1991; 266: 4464–4470
- Doering TL, Schekman R. Glycosyl-phosphatidylinositol anchor attachment in a yeast in vitro system. Biochem J 1997; 328: 669–675
- Sharma DK, Vidugiriene J, Bangs JD, Menon AK. A cell-free assay for glycosylphosphatidylinositol anchoring in African trypanosomes. Demonstration of a transamidation reaction mechanism. J Biol Chem 1999; 274: 16479–16486
- Kodukula K, Maxwell SE, Udenfriend S. Processing of nascent proteins to glycosylphosphatidylinositol-anchored forms in cell-free systems. Methods Enzymol 1995; 250: 536–547
- Kodukula K, Cines D, Amthauer R, Gerber L, Udenfriend S. Biosynthesis of phosphatidylinositol-glycan (PI-G)-anchored membrane proteins in cell-free systems: cleavage of the nascent protein and addition of the PI-G moiety depend on the size of the COOH-terminal signal peptide. Proc Natl Acad Sci USA 1995; 89: 1350–1353
- Johnston RB, Mycek MJ, Fruton JS. Catalysis of transamidation reactions by proteolytic enzymes. J Biol Chem 1950; 185: 629–641
- Fruton JS. Enzymic hydrolysis and synthesis of peptide bonds. Harvey Lect 1955; 51: 64–87
- Tate SS, Meister A. Stimulation of the hydrolytic activity and decrease of the transpeptidase activity of gamma-glutamyl transpeptidase by maleate; identity of a rat kidney maleate-stimulated glutaminase and gamma-glutamyl transpeptidase. Proc Natl Acad Sci USA 1974; 71: 3329–3333
- Maxwell SE, Ramalingam S, Gerber LD, Brink L, Udenfriend S. An active carbonyl formed during glycosylphosphatidylinositol addition to a protein is evidence of catalysis by a transamidase. J Biol Chem 1995; 270: 19576–19582
- Ramalingam S, Maxwell SE, Medof ME, Chen R, Gerber LD, Udenfriend S. COOH-terminal processing of nascent polypeptides by the glycosylphosphatidylinositol transamidase in the presence of hydrazine is governed by the same parameters as glycosylphosphatidylinositol addition. Proc Natl Acad Sci USA 1996; 93: 7528–7533
- Sharma DK, Hilley JD, Bangs JD, Coombs GH, Mottram JC, Menon AK. Soluble GPI8 restores glycosylphosphatidylinositol anchoring in a trypanosome cell-free system depleted of lumenal endoplasmic reticulum proteins. Biochem J 2000; 351: 717–722
- Benghezal M, Lipke PN, Conzelmann A. Identification of six complementation classes involved in the biosynthesis of glycosylphosphatidylinositol anchors in Saccharomyces cerevisiae. J Cell Biol 1995; 130: 1333–1344
- Benghezal M, Benachour A, Rusconi S, Aebi M, Conzelmann A. Yeast Gpi8p is essential for GPI anchor attachment onto proteins. Embo J 1996; 15: 6575–6583
- Leidich SD, Kostova Z, Latek RR, Costello LC, Drapp DA, Gray W, Fassler JS, Orlean P. Temperature-sensitive yeast GPI anchoring mutants gpi2 and gpi3 are defective in the synthesis of N-acetylglucosaminyl phosphatidylinositol. Cloning of the GPI2 gene. J Biol Chem 1995; 270: 13029–13035
- Schonbachler M, Horvath A, Fassler J, Riezman H. The yeast spt14 gene is homologous to the human PIG-A gene and is required for GPI anchor synthesis. Embo J 1995; 14: 1637–1645
- Hamburger D, Egerton M, Riezman H. Yeast Gaa1p is required for attachment of a completed GPI anchor onto proteins. J Cell Biol 1995; 129: 629–639
- Vossen JH, Ram AF, Klis FM. Identification of SPT14/CWH6 as the yeast homologue of hPIG-A, a gene involved in the biosynthesis of GPI anchors. Biochim Biophys Acta 1995; 1243: 549–551
- Gaynor EC, Mondesert G, Grimme SJ, Reed SI, Orlean P, Emr SD. MCD4 encodes a conserved endoplasmic reticulum membrane protein essential for glycosylphosphatidylinositol anchor synthesis in yeast. Mol Biol Cell 1999; 10: 627–648
- Ellis M, Sharma DK, Hilley JD, Coombs GH, Mottram JC. Processing and trafficking of Leishmania mexicana GP63. Analysis using GP18 mutants deficient in glycosylphosphatidylinositol protein anchoring. J Biol Chem 2002; 277: 27968–27974
- Eisenhaber B, Maurer-Stroh S, Novatchkova M, Schneider G, Eisenhaber F. Enzymes and auxiliary factors for GPI lipid anchor biosynthesis and post-translational transfer to proteins. Bioessays 2003; 25: 367–385
- Rawlings ND, Barrett AJ. Families of cysteine peptidases. Methods Enzymol 1994; 244: 461–486
- Rawlings ND, Tolle DP, Barrett AJ. MEROPS: the peptidase database. Nucleic Acids Res 2004; 32: D160–164
- Rawlings ND, Morton FR, Barrett AJ. MEROPS- the peptidase database. 2005. Available at: http://merops.sanger.ac.uk/.
- Abe Y, Shirane K, Yokosawa H, Matsushita H, Mitta M, Kato I, Ishii S. Asparaginyl endopeptidase of jack bean seeds. Purification, characterization, and high utility in protein sequence analysis. J Biol Chem 1993; 268: 3525–3529
- Kembhavi AA, Buttle DJ, Knight CG, Barrett AJ. The two cysteine endopeptidases of legume seeds: purification and characterization by use of specific fluorometric assays. Arch Biochem Biophys 1993; 303: 208–313
- Alonso JM, Granell A. A putative vacuolar processing protease is regulated by ethylene and also during fruit ripening in Citrus fruit. Plant Physiol 1995; 109: 541–547
- Ishii S. Legumain: asparaginyl endopeptidase. Methods Enzymol 1994; 244: 604–615
- Chen JM, Dando PM, Rawlings ND, Brown MA, Young NE, Stevens RA, Hewitt E, Watts C, Barrett AJ. Cloning, isolation, and characterization of mammalian legumain, an asparaginyl endopeptidase. J Biol Chem 1997; 272: 8090–8098
- Chen JM, Rawlings ND, Stevens RA, Barrett AJ. Identification of the active site of legumain links it to caspases, clostripain and gingipains in a new clan of cysteine endopeptidases. FEBS Lett 1998; 441: 361–365
- Dalton JP, Hola-Jamriska L, Brindley PJ. Asparaginyl endopeptidase activity in adult Schistosoma mansoni. Parasitology 1995; 111: 575–580
- Hara-Nishimura I. Asparaginyl endopeptidase. The handbook of proteolytic enzymes2nd edn, AJ Barrett, ND Rawlings, JF Woessner. Academic Press, London 1998
- Min W, Jones DH. In vitro splicing of concanavalin A is catalyzed by asparaginyl endopeptidase. Nat Struct Biol 1994; 1: 502–504
- Shimada T, Yamada K, Kataoka M, Nakaune S, Koumoto Y, Kuroyanagi M, Tabata S, Kato T, Shinozaki K, Seki M, Kobayashi M, Kondo M, Nishimura M, Hara-Nishimura I. Vacuolar processing enzymes are essential for proper processing of seed storage proteins in Arabidopsis thaliana. J Biol Chem 2003; 278: 32292–32299
- Takeda O, Miura Y, Mitta M, Matsushita H, Kato I, Abe Y, Yokosawa H, Ishii S. Isolation and analysis of cDNA encoding a precursor of Canavalia ensiformis asparaginyl endopeptidase (legumain). J Biochem (Tokyo) 1994; 116: 541–546
- Manoury B, Hewitt EW, Morrice N, Dando PM, Barrett AJ, Watts C. An asparaginyl endopeptidase processes a microbial antigen for class II MHC presentation. Nature 1998; 396: 695–699
- Vidugiriene J, Vainauskas S, Johnson AE, Menon AK. Endoplasmic reticulum proteins involved in glycosylphosphatidylinositol anchor attachment: photocrosslinking studies in a cell-free system. Eur J Biochem 2001; 268: 2290–2300
- Spurway TD, Dalley JA, High S, Bulleid NJ. Early events in glycosylphosphatidylinositol anchor addition. Substrate proteins associate with the transamidase subunit gpi8p. J Biol Chem 2001; 276: 15975–15982
- Kang X, Szallies A, Rawer M, Echner H, Duszenko M. GPI anchor transamidase of Trypanosoma brucei: in vitro assay of the recombinant protein and VSG anchor exchange. J Cell Sci 2002; 115: 2529–2539
- Meyer U, Benghezal M, Imhof I, Conzelmann A. Active site determination of Gpi8p, a caspase-related enzyme required for glycosylphosphatidylinositol anchor addition to proteins. Biochemistry 2000; 39: 3461–3471
- Ohishi K, Inoue N, Maeda Y, Takeda J, Riezman H, Kinoshita T. Gaa1p and gpi8p are components of a glycosylphosphatidylinositol (GPI) transamidase that mediates attachment of GPI to proteins. Mol Biol Cell 2000; 11: 1523–1533
- Hiroi Y, Komuro I, Chen R, Hosoda T, Mizuno T, Kudoh S, Georgescu SP, Medof ME, Yazaki Y. Molecular cloning of human homolog of yeast GAA1 which is required for attachment of glycosylphosphatidylinositols to proteins. FEBS Lett 1998; 421: 252–258
- Hiroi Y, Chen R, Sawa H, Hosoda T, Kudoh S, Kobayashi Y, Aburatani H, Nagashima K, Nagai R, Yazaki Y, Medof ME, Komuro I. Cloning of murine glycosyl phosphatidylinositol anchor attachment protein, GPAA1. Am J Physiol Cell Physiol 2000; 279: C205–212
- Nagamune K, Ohishi K, Ashida H, Hong Y, Hino J, Kangawa K, Inoue N, Maeda Y, Kinoshita T. GPI transamidase of Trypanosoma brucei has two previously uncharacterized (trypanosomatid transamidase 1 and 2) and three common subunits. Proc Natl Acad Sci USA 2000; 100: 10682–10687
- Vainauskas S, Maeda Y, Kurniawan H, Kinoshita T, Menon AK. Structural requirements for the recruitment of Gaa1 into a functional glycosylphosphatidylinositol transamidase complex. J Biol Chem 2002; 277: 30535–30542
- Vainauskas S, Menon AK. A conserved proline in the last transmembrane segment of Gaa1 is required for glycosylphosphatidylinositol (GPI) recognition by GPI transamidase. J Biol Chem 2003; 279: 6540–6545
- Fraering P, Imhof I, Meyer U, Strub JM, Van Dorsselaer A, Vionnet C, Conzelmann A. The GPI transamidase complex of Saccharomyces cerevisiae contains Gaa1p, Gpi8p, and Gpi16p. Mol Biol Cell 2001; 12: 3295–3306
- Ohishi K, Inoue N, Kinoshita T. PIG-S and PIG-T, essential for GPI anchor attachment to proteins, form a complex with GAA1 and GPI8. Embo J 2001; 20: 4088–4098
- Zhu Y, Fraering P, Vionnet C, Conzelmann A. Gpi17p does not stably interact with other subunits of glycosylphosphatidylinositol transamidase in Saccharomyces cerevisiae. Biochim Biophys Acta 2005; 1735: 79–88
- Winzeler EA, Shoemaker DD, Astromoff A, Liang H, Anderson K, Andre B, Bangham R, Benito R, Boeke JD, Bussey H, Chu AM, Connelly C, Davis K, Dietrich F, Dow SW, El Bakkoury M, Foury F, Friend SH, Gentalen E, Giaever G, Hegemann JH, Jones T, Laub M, Liao H, Davis RW, et al. Functional characterization of the S. cerevisiae genome by gene deletion and parallel analysis. Science 1999; 285: 901–906
- Ohishi K, Nagamune K, Maeda Y, Kinoshita T. Two subunits of GPI transamidase GPI8 and PIG-T form a functionally important intermolecular disulfide bridge. J Biol Chem 2003; 278: 13959–13967
- Hong Y, Ohishi K, Kang JY, Tanaka S, Inoue N, Nishimura J, Maeda Y, Kinoshita T. Human PIG-U and yeast Cdc91p are the fifth subunit of GPI transamidase that attaches GPI-anchors to proteins. Mol Biol Cell 2003; 14: 1780–1789
- Ash C, Jasny BR. Trypanosomatid genomes. Introduction. Science 2005; 309: 399
- Berriman M, Ghedin E, Hertz-Fowler C, Blandin G, Renauld H, Bartholomeu DC, et al. The genome of the African trypanosome Trypanosoma brucei. Science 2005; 309: 416–422
- El-Sayed NM, Myler PJ, Bartholomeu DC, Nilsson D, Aggarwal G, Tran AN, et al. The genome sequence of Trypanosoma cruzi, etiologic agent of Chagas disease. Science 2005; 309: 409–415
- Ivens AC, Peacock CS, Worthey EA, Murphy L, Aggarwal G, Berriman M, et al. The genome of the kinetoplastid parasite, Leishmania major. Science 2005; 309: 436–442
- Trypanosoma cruzi Genome Consortium. 2005. TcruziDB. Available from: http://tcruzidb.org/.
- Kinoshita T, Inoue N. Dissecting and manipulating the pathway for glycosylphos-phatidylinositol-anchor biosynthesis. Curr Opin Chem Biol 2000; 4: 632–638
- Ralton JE, McConville MJ. Delineation of three pathways of glycosylphosphatidylinositol biosynthesis in Leishmania mexicana. Precursors from different pathways are assembled on distinct pools of phosphatidylinositol and undergo fatty acid remodeling. J Biol Chem 1998; 273: 4245–4257