Abstract
A nano-aggregate liposome-polycation-DNA (LPD), composed of a cationic lipid, protamine and plasmid DNA was found to effectively deliver a human papillomavirus (HPV)-E7 epitope antigen to the antigen presenting cells of the immune system, eliciting enhanced anti-tumor immune responses in mouse models of cervical carcinoma. Both the cationic liposome and plasmid DNA were essential for the full immunostimulation activity of LPD. Interestingly, cationic liposomes alone could stimulate the antigen presenting dendritic cells (DC) leading to the expression of co-stimulatory molecules, CD80 and CD86. However, cationic lipids could not stimulate DC for the expression of pro-inflammatory cytokines. Moreover, they were unable to enhance the expression of NF-κB, suggesting that dendritic cells stimulation by cationic lipids is signaled through an NF-κB independent mechanism. DC stimulation was specific to cationic lipids, the zwitterionic and anionic lipids showed little or no activity. The ability of different cationic lipids to stimulate the expression of co-stimulatory molecules on DC varied significantly. In general, the cationic lipids bearing ethyl phosphocholine head groups were better stimulants than their trimethylammonium counterparts. In case of the cationic lipids bearing trimethyl ammonium head groups, the ones bearing unsaturated or shorter saturated hydrophobic chains exhibited enhanced immunostimulatory activity. The LPS-induced TNF-α expression by dendritic cells was inhibited by active cationic lipids but not the inactive ones, suggesting the possible involvement of lipopolysaccharide binding protein (LBP) in cationic lipid mediated DC stimulation. Based on the structure-specific activation of dendritic cells by cationic lipids, a model for the immunostimulation of DC by such lipids is proposed.
Acronyms | ||
DC | = | dendritic cells |
BMDC | = | bone marrow-derived dendritic cells |
LPD | = | Lipid-Polycation-DNA |
DOTAP | = | 1,2-dioleoyl-3-trimethylammoninum propane |
DOPE | = | 1,2-dioleoyl-sn-glycero-3-phosphoethanolamine |
DOSPA | = | 2,3-dioleyloxy-N-[2-(sperminecarboxamido)ethyl]-N-N-dimethyl-1-propanaminium trifluoroacetate |
DOTMA | = | N-[1-(2,3-dioleyloxy)propyl]-N,N,N-trimethylammonium chloride |
DMRIE | = | 1,2-dimyritsyloxypropyl-3-dimethyl-hydroxyethyl ammonium bromide |
DMKE | = | O,O’-dimyristyl-N-lysyl aspartate |
DMKD | = | O,O’-dimyristyl-N-lysyl glutamate |
DDAB | = | dimethyldioctadecylammonium bromide |
DMTAP | = | 1,2-dimyristoyl-3-trimethylammonium propane |
DPTAP | = | 1,2-dipalmitoyl-3-trimethylammonium propane |
DSTAP | = | 1,2-distearoyl-3-trimethylammonium propane |
EPC | = | ethyl phosphocholine |
DOEPC | = | 1,2-dioleoyl-sn-glycero-3-ethylphosphocholine |
DMEPC | = | 1,2-dimyristoyl-sn-glycero-3-ethylphosphocholine |
DPEPC | = | 1,2-dipalmitoyl-sn-glycero-3-ethylphosphocholine |
DSEPC | = | 1,2-distearoyl-sn-glycero-3-ethylphosphocholine |
DLEPC | = | 1,2-dilauroyl-sn-glycero-3-ethylphosphocholine |
DOG | = | dioleoyl glycerol |
Introduction
Vaccine systems that are designed based on proteins, peptides or DNA are of great current interest. Due to the lower efficiencies of these new-generation vaccines, however, they are seldom used alone. Rather, their formulations often contain adjuvants either to enhance the immune responses or to reduce the dosing Citation[1], Citation[2]. In this direction, liposome-based vaccine formulations have been developed and were found to be capable of augmenting the immune responses of various vaccines Citation[3–5]. Felgner et al. Citation[6] has also reported that cationic lipids are capable of facilitating transport of biologically active agents into cells, including the transfection of cells by therapeutic polynucleotides, the delivery of antiviral drugs, and the introduction of immunogenic peptides. It has been proposed that the adjuvant effect of liposomes was due to the selective uptake of liposomal antigens into the regional lymph nodes and more efficient antigen presentation due to antigen clustering and massive delivery to the antigen presenting cells Citation[7].
Recently we have reported a cationic lipid-based cancer vaccine system toward human papillomavirus (HPV)-mediated cervical carcinoma in which LPD (lipid-polycation-DNA) was used as an adjuvant Citation[8]. LPD, originally designed as a DNA delivery system Citation[9], is a self-assembled nano-aggregate comprising a cationic lipid, protamine and plasmid DNA. Intravenous administration of LPD was found to potentiate efficient anti-tumor activity in murine tumor model 24JK cells, even when the formulation contained an empty, non-coding plasmid Citation[10]. The observed anti-tumor activity was thought to originate from the innate immunostimulatory activity of the unmethylated CpG motif sequences present in the plasmid DNA. It was further correlated with its ability to induce Th-1 cytokines like TNF-α, IFN-γ and IL-12 and to the development of tumor-specific CD8+ cytotoxic lymphocytes (CTL) Citation[11], Citation[12].
When LPD nanoparticles loaded with a MHC class I-restricted peptide (RAHYNIVTF) epitope antigen derived from E7 protein of HPV-16 was used to immunize the mice, a strong antigen-specific anti-tumor response was observed Citation[8]. It induced an E7-specific CTL response and prevented the establishment of E7-expressing TC-1 tumors. Moreover, administration of LPD/E7 to TC-1 tumor-bearing mice caused complete tumor regression. Flow cytometry experiments with fluorescently labeled complexes revealed that LPD/peptide particles are selectively accumulated in the spleen or the local nymph nodes and are effectively taken up by the antigen presenting cells of the immune system. Moreover, a murine dendritic cell line (DC2.4) pulsed with LPD/E7 complex could effectively induce an E7-specific CTL response and immunize the mouse against tumor challenge. It was also observed that LPD particles could stimulate the expression of co-stimulatory molecules CD80 and CD86 in this cell line.
To elucidate the immunostimulatory mechanism of LPD and to further improvise its adjuvant activity we envisaged a detailed mechanistic investigation of the stimulatory activity of LPD on dendritic cells. DC, being the primary antigen capturing and presenting cell of the immune system, offers an excellent experimental system to understand the adjuvant effects of LPD. The murine cell line DC2.4, which has proven to be a good in vitro model for the antigen presenting cells (APCs) was selected for these studies Citation[13], Citation[14]. The observations were further correlated with primary dendritic cells derived from the murine bone marrow. During our investigation, we observed that the cationic lipid part of the LPD formulation significantly influences its immunostimulatory activity on dendritic cells. DC activation correlates with the concentration of cationic lipid. The fact that cationic lipids alone could stimulate the antigen presenting cells was of high importance since the immunomodulatory adjuvant activities of LPD were initially thought to be arising only by unmethylated CpG motifs of the plasmid DNA via toll-like receptor 9 (TLR9). The present manuscript details our systematic investigation of structure-specific immunomodulatory effects of cationic lipids on DC. Our data suggests the existence of a possible cationic lipid receptor (CLR) on such antigen presenting cells.
Materials and methods
Lipids, plasmid and cells
DOTAP, DMTAP, DPTAP, DSTAP, DOEPC, DLEPC, DMEPC, DPEPC, DSEPC were purchased from Avanti Polar Lipids, Inc. (Alabaster, AL). Lipofectamine®, a 3:1 (w/w) liposome formulation of DOSPA and DOPE, Lipofectin®, a 1:1 (w/w) liposome formulation of DOTMA and DOPE, and DMRIE-C®, a 1:1 (m/m) liposome formulation of DMRIE and cholesterol were purchased from Invitrogen (Carlsbad, CA). DMKE and DMKD were provided by Dr Yong-Serk Park from Yonsei University (Seoul, South Korea). DDAB, protamine sulfate (fraction X from salmon) and SDS were from Sigma-Aldrich (St Louis, MO). Plasmid (pNGVL3) containing a CMV promoter and no coding insert were obtained from the National Gene Vector Laboratory (Ann Arbor, MI). Plasmid DNA was purified using the Endofree Giga-Prep kit (Qiagen, Valencia, CA).
DC2.4 cell line (obtained from Dr. Kenneth L. Rock, Dana Farber Cancer Institute, Boston, MA) was derived from C57BL/6 bone marrow cells. DC2.4 cells were grown in RPMI-1640 medium (Invitrogen) supplemented with 10% fetal bovine serum (FBS), 100 U/ml of penicillin (Sigma) and 100 µg/ml of Streptomycin (Sigma).
Preparation of primary dendritic cells
Primary dendritic cells (DC) were prepared from the bone marrow of C57BL/6 mice as described Citation[26]. Cells were cultured in RPMI-1640 supplemented with 10% FBS, antibiotic/antimycotic agents, 2-mercaptoethanol and the cytokines, GM-CSF and IL-4 (103 U/ml each). Briefly, the bone marrow taken from the femur and tibia was passed through a cell strainer and washed with PBS. Cells were then incubated with RBC lysis buffer for 5 min, washed with PBS and incubated for 40 min with antibodies against CD4, CD8 and B220. The antibodies were generated from the corresponding production cell lines, GK1.5 (ATCC TIB 207), 2.43 (ATCC TIB 210), B220 (anti-B cell surface glycoprotein, ATCC TIB 146), respectively. Cells were then depleted of lymphocytes by incubating with rabbit complement (Cedarlane International distributors) for 30 min at 37°C. Cells were pelleted, rinsed with media and then cultured in T-75 flasks at a concentration of 5×105 cells/ml. A total of 75% of the media was changed every alternate day. Loosely adherent cells were collected on day 5 and stained using anti-CD11c-conjugated magnetic beads (Miltenyi Biotec, Bergisch Gladbach, Germany). Primary DC were purified by immunomagnetic bead cell sorting (purity > 80% as confirmed by flow cytometry analysis). Purified DC were washed twice in PBS and used for the experiments.
Preparation of liposomes and LPD
Unless otherwise stated, liposomes (5 mM) were prepared in the following experiments. Cell culture grade water (Cambrex, Walkersville, MD) was used in all liposome preparation procedures. Briefly, lipid films were made in glass vial by evaporating the chloroform solution under a steady stream of dry nitrogen gas. Traces of organic solvents were removed by keeping these films under vacuum overnight. Lipid films were then hydrated for 12 h by adding required amount of water to make final concentration of 5 mM. Hydrated lipid suspensions were freeze-thawed between 0°C and 60°C with intermittent vortexing. The suspensions were then sonicated in a bath type sonicator for 30 min at 50°C followed by extrusion (Hamilton Co., Reno, NV) through 600, 400 and 100 nm membranes filters and stored at 4°C.
For the preparation of LPD, DOTAP liposomes were made at a concentration of 10 mg/ml following the same route as mentioned above. LPD formulation comprises DOTAP, protamine and plasmid DNA at a ratio of 9:0.6:1 (w/w/w). To prepare LPD, required amounts of liposomes (43 µl) and protamine (30 µg) were dispersed in 150 µl of water. 150 µl of aqueous solution of pDNA (30 µg) was then added drop wise into the mixture of liposome and protamine with gentle shaking. The complex was allowed to stay at room temperature for at least 20 min prior to use.
The particle size and the zeta (ζ) potential of the liposome, LPD, and LPD-derived formulations were measured following the manufacturer's suggestion using a Coulter N4 Plus particle sizer (Beckman Coulter, San Francisco, CA) and a Zetasizer 4 (Malven Instruments, Inc., Southborough, MA), respectively. Particle sizes were reported as the mean±SD [polydispersity index (PI)]. Zeta potentials were reported as the mean±SD (n=3).
Expression of co-stimulatory molecules (CD80/86) on DC2.4 cells after in vitro stimulation
All of the fluorescent antibodies were purchased from BD Biosciences (San Diego, CA). DC2.4 cells (1.5×106 cells per well) were seeded into a 24-well plate in 2 ml of culture medium per well and incubated with liposomal formulations (75 µM liposome/surfactant unless otherwise mentioned) or LPD 75 µl (containing 75 µM liposome) at a 37°C, 5% CO2 incubator. After 16 h, cells were pelleted and washed twice with BD Pharmingen staining buffer and then stained with FITC-labeled anti-CD80 antibody or PE labeled anit-CD86 antibody for 20 min at 4°C. Cells were washed twice to remove any unbound antibody and were analyzed for FITC or PE fluorescence using an EPICS-XL bench top flow cytometer (Beckman Coulter) equipped with EXPO 32 software. Data are represented as the percent population of DC2.4 cells that were CD80 or CD86 positive.
For primary dendritic cells, double-staining was performed with PE labeled anti-CD11c antibody and FITC-labeled anti-CD80 or anti-CD86 antibody. The live population was gated based on size and granularity and only those cells that are CD11c positive were considered for the evaluation of the expression of CD80 or CD86. Appropriate isotype controls were used in each experiment.
In the case of liposomal formulations like Lipofectin®, Lipofectamine®, DMRIE-C®, the equivalent amount (wt) corresponding to the cationic lipid formulations were used to stimulate DC2.4 cells.
Release of TNF-α from DC after in vitro stimulation
DC2.4 cells (1.5×106 cells/well, n=3) were seeded into 24-well plates and incubated with the liposomal formulations (75 µM) at 37°C, 5% CO2 for the desired time points. TNF-α concentration in the culture supernatant was determined by using an ELISA kit from R&D System Inc. (Minneapolis, MN). For the competitive binding experiments between cationic lipid and LPS, the cationic lipids were incubated with the cells in the presence of 10% serum for at least 30 min before the addition of LPS (List Biological Laboratories, Inc). The concentration of LPS was kept at 0.5 µg per ml.
Results and discussion
The interaction of pathogen-associated molecular pattern (PAMP) and toll-like receptors (TLR) is crucial in inducing both innate and adaptive immunity Citation[15]. TLRs were identified as major recognition receptors for PAMPs such as LPS, peptidoglycan, lipoteichoic acid, CpG-containing oligonucleotides (CpG-ODN) and dsRNA Citation[15], Citation[16]. Dendritic cells (DC) constitute an important class of antigen presenting cells (APCs) of the immune system and are involved in antigen capturing, processing and subsequent presentation to the antigen-specific T cells Citation[17]. They are the key elements in the optimization of antigen capture and the clonal expansion of the rare CD4+ and CD8+ T lymphocytes. Moreover, they also function as inducers of tolerance by the deletion of self-reactive thymocytes and anergy of mature T cells. Interaction of PAMP with TLR on DC stimulates the maturation of DC, which then migrate to the local lymph nodes (LN) to present antigen to T cells as a complex of MHC/antigen. In addition, TLR signaling also stimulates the expression of co-stimulatory molecules such as CD80/CD86, which are essential for successful antigen presentation and the subsequent clonal expansion. Besides CD80/CD86 expression, production of pro-inflammatory cytokines such as TNF-α by APC is another indication of TLR signaling. Such strong influences of TLR on immunostimulation has led to the concept of TLR as a general adjuvant receptor and the expression of co-stimulatory molecules and cytokines by dendritic cells are considered as a good indication of immunostimulatory activities of adjuvants.
To have a better understanding of the immunostimulation mechanism of LPD and to improve the vaccine formulation further, we systematically followed the stimulation of dendritic cells by LPD. The well-established dendritic cell line DC2.4 was primarily used for experiments, but the data was further confirmed with murine bone marrow-derived primary dendritic cells (BMDC). It was found that LPD stimulates the DC2.4 cells toward the expression of co-stimulatory molecules, CD80 and CD86 (). It also induced a strong TNF-α release in DC2.4 cells. Interestingly, we observed that the cationic lipid component, dioleoyl-trimethyl amino propane (DOTAP) of the LPD/E7 formulation alone stimulated the DC2.4 cells toward the expression of co-stimulatory molecules Citation[18], Citation[19] and the EC50 of DOTAP liposome was around 30 µM (A). DOTAP liposome also stimulated the primary dendritic cells derived from murine bone marrow (C). The stimulatory activity of cationic liposome (139±37 nm, 27±2 mV) alone comprised greater than 60% activity of LPD. This strongly suggests that the cationic lipid part of LPD is responsible for majority of the LPD adjuvant activity. We have also done independent MTT assays which revealed that cell viability was greater than 80% at 75 µM lipid concentration and the IC50 of DOTAP liposome was around 250–300 µM (data not shown). This result ruled out the possibility that DOTAP could bind to DNA/RNA released from apoptotic/necrotic cells during the cell-culture period and subsequent translocation of its cargo into the TLR9 pathway Citation[20]. A concentration of 75 µM liposomes was therefore used for all further experiments.
Figure 1. DC2.4 cells activation by cationic liposome stimulation. (A) Expression of co-stimulatory molecule CD80 on DC2.4 cells after incubation with DOTAP of different concentration or with LPD (contains 75 µM of DOTAP). Experiments were performed in triplicates and the data reported as mean±SD (n=3). (B) Expression of co-stimulatory molecules, CD80 and CD86 on DC2.4 cells after stimulation with different cationic liposomes.
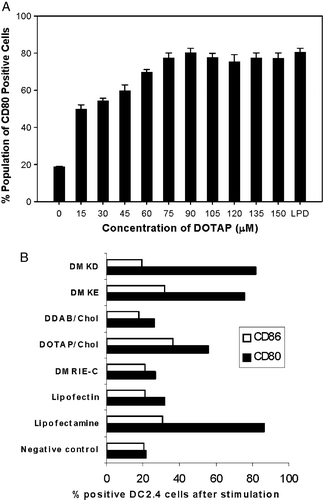
Figure 2. Influence of lipid head group on the expression of CD80 on DC2.4 cells or bone marrow derived dendritic cells. DC2.4 cells were stimulated by liposomes with different charged head groups (A) or counter ions (B) and CD80 expression on DC2.4 was measured by flow cytometry as described. The concentration of each liposomal formulation was 75 µM. LPD is the positive control. Untreated cells stained with FITC anti-CD80 were used as negative control. Experiments were done in triplicates and the data shown as the mean±SD. (C) Expression of co-stimulatory molecule CD80 on bone marrow derived primary dendritic cells upon incubation with various liposomal formulations. The concentration of each formulation was 75 µM. DCs were stained with anti-CD11c and anti-CD80 antibodies. Untreated cells were used as negative control (thin lines) and LPS treated cells (1 µg/ml) was used as positive control. Solid line represents the treated samples where as the shaded region represents the isotype control.
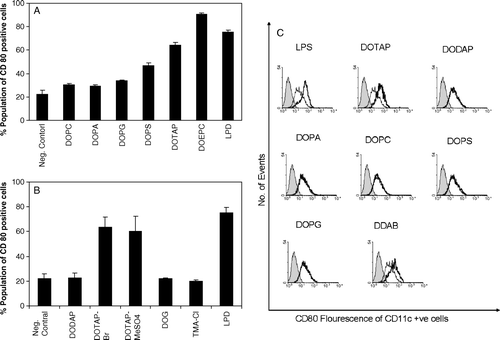
Since a significant amount of the immunostimulatory effect of LPD was from the cationic lipid part, we tested other cationic lipid formulations for their ability to stimulate the expression of co-stimulatory molecules by DC2.4 cells (B). It was observed that the ability to stimulate the DC2.4 cells by different cationic lipids varies significantly. Lipofectamine®, a 3:1 (w/w) liposome formulation of the polycationic lipid 2,3-dioleyloxy-N-[2(sperminecarboxamido)ethyl]-N,N-dimethyl-1-propanaminium trifluoroacetate (DOSPA) and the neutral lipid dioleoyl phosphatidylethanolamine (DOPE), and liposomes prepared from O,O’-dimyristyl-N-lysyl aspartate (DMKE) and O,O’-dimyristyl-N-lysyl-glutamate (DMKD), strongly stimulated the expression of CD80/CD86 on DC2.4 cells. Lipofectin®, a 1:1 (w/w) liposome formulation of cationic lipid N-[1-(2,3-dioleyloxy)propyl]-N,N,N-trimetylammonium chloride (DOTMA) and DOPE, DMRIE-C®, a 1:1 (M/M) liposome formulation of cationic lipid 1,2-dimyristyloxypropyl-3-dimethyl-hydroxy ethyl ammonium bromide (DMRIE) and cholesterol, and liposomes prepared from cationic lipid dimethyl dioctadecyl ammonium bromide (DDAB) and cholesterol did not show any significant DC2.4 stimulation on the expression of CD80/CD86. Many of these liposomal formulations are known to be efficient gene transfection reagents. Thus, it was interesting to investigate how and why certain lipids activate the dendritic cells, while others do not.
To decipher a possible structure-activity relationship in cationic lipid-mediated immunostimulation, a series of lipids bearing the same hydrocarbon chains and different head group functionalities were used to stimulate the dendritic cells. Only the positively charged lipids significantly induce the expression co-stimulatory molecules in DC2.4 cells and BMDCs (A and C). The expression of CD86 essentially followed the same trend as that of CD80 (data not shown). The zwitterionic lipid, dioleoyl phosphatidyl choline (DOPC) and the negatively charged lipids dioleoyl phosphatidic acid (DOPA) and dioleoyl phosphatidyl glycerol (DOPG) did not stimulate dendritic cells. Negatively charged lipid, dioleoyl phosphatidyl serine (DOPS) could stimulate the DC2.4 cells but to a much lower level. Even lower levels of stimulation by DOPS were observed in primary dendritic cells.
The ability of different cationic lipids to stimulate the dendritic cells also varied significantly. The activity of dioleoyl dimethylaminoproapne (DODAP) was significantly lower than that of DOTAP. DODAP and DOTAP differ only in the fact that DODAP possesses a weak positive charge with a tertiary amine group as the head group while DOTAP has a strong charge with a quaternary ammonium moiety at the head group level. We also found that DOEPC which has an ethylphosphocholine (EPC) head group induced significantly higher levels of CD80 expression in DC2.4 than DOTAP, which contains a trimethylaminopropane head group functionality (A). The effect of counter ions in the liposomal formulations was found to be insignificant during such activation events (B). Further, the neutral lipid, dioleoyl glycerol (DOG) bearing similar hydrocarbon and linkage functionalities as that of DOTAP did not stimulate DC2.4 cells toward co-stimulatory molecule expression.
Apart from the head group structure dependency, the ability of different cationic lipids to stimulate the dendritic cells was also significantly influenced by the hydrocarbon chain of the individual lipid monomers. The effect of the hydrocarbon tail region of cationic lipids on dendritic cell stimulation is presented in . In general, the DXEPC lipids with the ethyl phosphocholine (EPC) head groups were more potent than the DXTAP lipids with trimethylammonium propane (TAP) head group. Within the lipids bearing identical head group structure, lipids with shorter [1,2-dilauroyl-sn-glycero-3-ethylphosphocholine (DLEPC-12:0), 1,2-dimyristoyl-sn-glycero-3-ethylphosphocholine (DMEPC-14:0)] or unsaturated [1,2–dioleoyl-sn-glycero-3-ethylphosphocholine (DOEPC-18:1)] acyl chains stimulated the DC2.4 cells better than those with longer [1,2-dipalmitoyl-sn-glycero-3-ethylphosphocholine (DPEPC-16:0)] or saturated [1,2-distearoyl-sn-glycero-3-ethylphosphocholine (DSEPC-18:0)] acyl chains. Similar trends were also observed with lipids having TAP head groups. 1,2-distearoyl-3-trimethylammonium propane (DSTAP-18:0) and 1,2-palmitoyl-3-trimethylammonium propane (DPTAP-16:0) induced much lower expression of CD80 on DC2.4 cells compared to that of 1,2-myristoyl-3-trimethylammonium propane (DMTAP-14:0) and 1,2-oleoyl-3-trimethylammonium propane (DOTAP-18:1). Similar trends in chain dependency were also observed in BMDCs in case of lipids having the TAP head groups (B). However, the cationic lipid, DOEPC failed to induce significant levels of co-stimulatory molecules in primary dendritic cells in contrast to DC2.4 cell line. Liposomes composed of lipids with unsaturated (18:1) or shorter hydrocarbon chains (12:0 or 14:0) are expected to be in their liquid crystalline state at ambient temperature and so would be more ‘fluid’ compared to their saturated analogues. So it is possible that, upon uptake by DC2.4 cells these liposomes fluidize the cell membrane. The observed higher immunostimulatory activity of such liposomes could therefore be happening due to the possible cell membrane destabilization, which could further trigger some pathways that stimulate the DC2.4 cells leading to the expression of co-stimulatory molecules. In this regard, it was observed that sodium dodecyl sulfate (SDS) could not activate DC2.4 cells () suggesting that the DC2.4 activation by cationic lipids with shorter hydrophobic chains were not just due to a possible detergent effect of shorter chain lipids on the cellular membrane. Rather, these observations point toward a structure-specific immunostimulatory activity of lipids influenced by both the hydrophilic head and the lipophilic tail of cationic lipids.
Figure 3. Effect of hydrocarbon chain lengths of cationic lipids on the expression of CD80 on DC2.4 cells or bone marrow derived dendritic cells. (A) DC2.4 cells were stimulated by liposomes with variant hydrocarbon chain lengths and CD80 expression was measured by flow cytometry. Data reported are the mean±SD (n=3). Concentration of the liposomes used was 75 µM. (B and C) Bone marrow derived mouse primary dendritic cells were incubated with liposomes with variant hydrocarbon chain lengths and CD80 expression was measured by flow cytometry. The concentration of each formulation was 75 µM. DCs were stained with anti-CD11c and anti-CD80 antibodies. Untreated cells were used as negative control (thin lines). Solid line represents the treated samples where as the shaded region represents the isotype control.
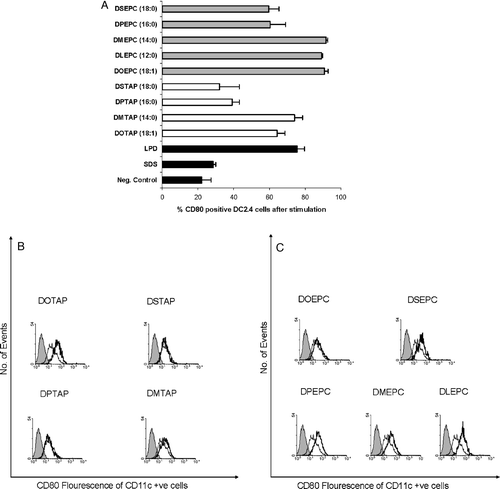
To further corroborate this observation, the effect of a fusogenic lipid, dioleoyl phosphatidyl ethanolamine (DOPE) on the stimulatory activity of cationic lipids was investigated. DOPE, is widely used as a helper lipid in cationic lipid-mediated gene delivery to enhance the transfection owing to its tendency to form hexagonal (HII) phases thus promoting the fusion of liposomes with cell membranes. However, as can be seen in A, DOPE did not enhance the expression of co-stimulatory molecules on DC2.4 when it was incorporated in cationic liposomes at a 1:1 molar ratio. Independent experiments showed that cationic lipid (i.e., DOEPC) could stimulate the DC2.4 cells even when they were added as a DMSO solution (B). Although this experiment suggests that the immunostimulation is likely a property of the lipid monomer, we cannot rule out the possibility that the involvement of lipid aggregates also contributes in the DC activation.
Figure 4. Influence of the fusogenic lipid on the expression of CD80 by cationic lipids on DC2.4 cells. (A) Cationic lipid concentration was used as 75 µM in all the formulations and the fusogenic lipid, DOPE was added in a 1:1 molar ratio. Untreated, FITC anti-CD80 stained cells were used as negative control. (B) DC2.4 cells were treated with DOEPC in either aqueous solution or in DMSO. Untreated and DMSO treated DC2.4 cells were used as controls. Data are presented as mean±SD (n=3).
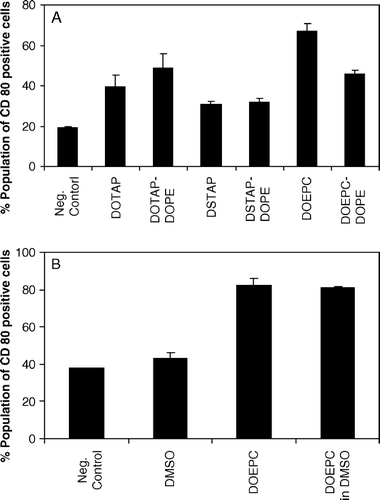
Besides the expression of co-stimulatory molecules on the dendritic cell surface, production of pro-inflammatory cytokines such as TNF-α by APC is another indication of the initiation of TLR signaling Citation[21]. compares the induction of TNF-α by different cationic lipids in DC2.4 cells with that of lipopolysaccharide (LPS), a strong activator of the innate immune system and a potent inducer of inflammation. It was observed that none of the cationic lipids could induce significant levels of TNF-α in DC2.4 cells. Moreover, from independent experiments it was found that the cationic liposome alone could not stimulate any luciferase expression in DC2.4 cells transfected with a plasmid encoding the luciferase gene driven by a NF-κB responsive promoter. Interestingly, significantly higher levels of both TNF-α secretion and luciferase expression driven by a NF-κB promoter were induced by LPD (data not shown). This result suggests that, among the composition of LPD, it is the DNA, but not lipid, contributes to the secretion of TNF-α as well as the activation of NF-κB. Cationic lipids possibly induce the expression of co-stimulatory molecules through a signaling cascade which does not involve the commonly observed NF-κB mediated signaling pathway. This finding again provides strong evidence that DNA and lipid trigger two distinct downstream pathways. In contrast to DNA that undergoes NF-κB activation via TLR9 and induces cytokines release; cationic lipid seems to go through a unique NF-κB independent pathway and not likely to induce inflammatory cytokines.
Figure 5. TNF-α release from DC2.4 cells upon 3 h incubation with different cationic lipids or lipopolysaccharide. The concentration of each of the lipid formulation was 75 µM. LPS was used at a concentration of 0.5 µg/ml. The data represented as mean±SD (n=3) of one representative experiment.
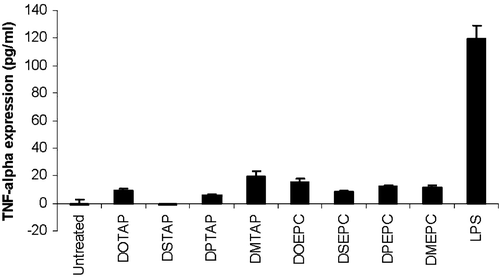
Taken together, the above experiments revealed that cationic liposomes stimulate the expression of co-stimulatory molecules on DC2.4 cells in a structure-specific manner. However, they were incapable of inducing any TNF-α cytokine release. A similar observation is also reported in the case of LPS acting via a TLR4 signaling cascade. There, the MyD88-independent pathway leads only to the expression of co-stimulatory molecules, but not the cytokine release Citation[21–23]. It is quite possible that such mechanism also exists for cationic lipid molecules. However, it is very unlikely that TLR4 might be the cationic lipid receptor since LPS, the ligand for TLR4, has a very different chemical structure and charge content compared to a cationic lipid such as DOTAP. Nevertheless, it was worthwhile to investigate if any such correlations exist between the signaling cascades of LPS and cationic lipids. It is interesting to note that, while investigating the relationship between chain length and the position of the fatty acyl groups to the ability of lipid A (the portion of LPS responsible for the adjuvant activity of LPS) to abolish the expression of suppressor T-cell activity, Baker et al observed that fatty acyl chain lengths of C12 and C14 appeared optimal for the T-cell suppressor activity Citation[24]. This coincidentally agrees with our observation that the cationic lipids with shorter (12:0 or 14:0) acyl chains are more potent immunostimulators than those with longer (16:0 or 18:0) chains. In the case of LPS, it is well known that a lipopolysaccharide binding protein (LBP) initiates the immunostimulatory signal transduction cascade of LPS by transferring the LPS monomers from its aggregates to the cell surface receptor CD14 Citation[25]. LBP is an acute phase protein and is shown to have increased levels of expression during inflammation. It has also been shown previously that in the absence of serum, much higher concentration of LPS is required to activate monocytes than in the presence of serum or of purified LBP Citation[25]. It was therefore interesting to see if there exists any signaling mechanism similar to LPS, probably involving LBP or any other specific cationic lipid binding protein (CLBP).
To investigate whether LPS and the cationic lipids activate the dendritic cells through a common mechanism, a competitive binding assay was designed. From the previous experiments, it is clear that cationic lipids like DOTAP do not induce cytokine expression by itself, whereas LPS does. Therefore, it is reasonable to assume that if a common binding partner exists between LPS and DOTAP in their signaling pathways, the presence of DOTAP should compete with LPS for its binding to the common partner and thus should inhibit the LPS-induced cytokine release. To test this hypothesis, DOTAP liposomes were incubated with the serum before the addition of LPS and its influence on LPS-induced cytokine expression on DC2.4 cells was monitored. As can be seen in A, it was indeed observed that DOTAP could inhibit the LPS-induced expression of TNF-α in DC2.4 cells suggesting that some of their signaling pathways could have some common partners. The inhibitory effect of DOTAP on LPS was more significant in the presence of serum suggesting that a serum element (could be LBP itself or some other components of serum) might also be involved in the signaling cascade initiated by DOTAP. Moreover, it was observed that the saturated analogue of DOTAP namely DSTAP, did not inhibit the LPS activity towards cytokine production (B). DOG, a lipid having the same hydrophobic chain portion as that of DOTAP but possessing no cationic head group also could inhibit LPS, but to a lesser extent. It is interesting to note here that the unsaturated analogues of cationic lipids were better inducers of co-stimulatory molecules than that of their corresponding saturated analogues. Taken together, these experiments point towards the possible existence of a cationic lipid binding protein (could be LBP itself) that possess similar activities of LBP as in the case of LPS. This hypothetical protein must have preferred binding affinity towards lipids bearing unsaturated oleoyl chains or shorter saturated chains, both occupying a reduced hydrophobic volume.
Figure 6. Inhibition of the LPS-induced TNF-α expression from DC2.4 cells by (a) DOTAP with the absence (w/o) or presence (w) of 10% serum or by (b) other different cationic lipids. The concentration of the lipid formulation was 75 µM. LPS was used at a concentration of 0.5 µg/ml. The data reported are mean±SD (n=3). For the inhibition experiments the cationic lipids were incubated with serum for 30 min before the addition of LPS.
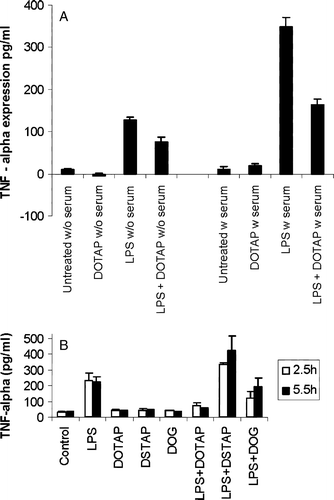
In summary, we have shown that cationic lipids stimulated the expression of co-stimulatory molecules in dendritic cells and this activity was structure and charge specific. By comparing different cationic lipids we were able to draw a structure-activity relationship for this class of molecule. Interestingly, among the cationic lipids with identical head groups, a characteristic hydrocarbon chain length dependency for dendritic cell stimulation was observed. Further, the inability of cationic lipids to induce the expression of NF-?B and TNF-α suggests that the activation of dendritic cells by cationic lipid follows a signaling pathway different from the MyD88/NF-κB route commonly observed during endotoxin stimulation. To account for all these observed data, we propose a hypothetic model (). An unknown cationic lipid receptor (CLR) could exist, either on the cell surface or inside the cell such as endosome, which interact with the cationic lipids to initiate a signaling cascade that leads to the expression of co-stimulatory molecules. The proposed cationic lipid binding protein (CLBP) might have the same function as that of LBP, bringing the lipid monomers from their aggregates toward CLR. The signaling cascade does not involve NF-κB and does not lead to the expression of pro-inflammatory cytokines. The differential ability of different cationic liposomes to invoke this signaling cascade might be either due to the selectivity of CLBP or CLR, or both. In any case, additional experiments are required to demonstrate if LBP and CLBP are essentially the same or if they share most of the activities. Experiments are underway in our laboratory to identify these proteins involved in cationic lipid mediated dendritic cell stimulation.
The authors would like to thank Erin McClelland at University of Pittsburgh Cancer Institute for her assistance with the flow cytometry analysis. This work was supported by NIH grants CA74918 and AI48851 to Leaf Huang.
References
- Cox JC, Coulter AR. Adjuvants – a classification and review of their modes of action. Vaccine 1997; 15: 248–256
- Singh M, O'Hagan D. Advances in vaccine adjuvants. Nat Biotechnol 1999; 17: 1075–1081
- Brunel F, Darbouret A, Ronco J. Cationic lipid DC-Chol induces an improved and balanced immunity able to overcome the unresponsiveness to the hepatitis B vaccine. Vaccine 1999; 17: 2192–2203
- Holten-Andersen L, Doherty TM, Korsholm KS, Andersen P. Combination of the cationic surfactant dimethyl dioctadecyl ammonium bromide and synthetic mycobacterial cord factor as an efficient adjuvant for tuberculosis subunit vaccines. Infect Immun 2004; 72: 1608–1617
- Perrie Y, Obrenovic M, McCarthy D, Gregoriadis G. Liposome (Lipodine)-mediated DNA vaccination by the oral route. J Liposome Res 2002; 12: 185–197
- Felgner PL, Kumar R, Basava C, Border RC, Hwang-Felgner JY. 1993. Cationic lipids for intracellular delivery of biologically active molecules. US Patent 5,459,127.
- Alving CR, Koulchin V, Glenn GM, Rao M. Liposomes as carriers of peptide antigens: induction of antibodies and cytotoxic T lymphocytes to conjugated and unconjugated peptides. Immunol Rev 1995; 145: 5–31
- Dileo J, Banerjee R, Whitmore M, Nayak JV, Falo LD, Jr, Huang L. Lipid-protamine-DNA-mediated antigen delivery to antigen-presenting cells results in enhanced anti-tumor immune responses. Mol Ther 2003; 7: 640–648
- Li S, Huang L. In vivo gene transfer via intravenous administration of cationic lipid-protamine-DNA (LPD) complexes. Gene Ther 1997; 4: 891–900
- Whitmore M, Li S, Huang L. LPD lipopolyplex initiates a potent cytokine response and inhibits tumor growth. Gene Ther 1999; 6: 1867–1875
- Whitmore MM, Li S, Falo L, Jr, Huang L. Systemic administration of LPD prepared with CpG oligonucleotides inhibits the growth of established pulmonary metastases by stimulating innate and acquired antitumor immune responses. Cancer Immunol Immunother 2001; 50: 503–514
- Li S, Wu SP, Whitmore M, Loeffert EJ, Wang L, Watkins SC, Pitt BR, Huang L. Effect of immune response on gene transfer to the lung via systemic administration of cationic lipidic vectors. Am J Physiol 1999; 276: L796–804
- Okada N, Saito T, Mori K, Masunaga Y, Fujii Y, Fujita J, Fujimoto K, Nakanishi T, Tanaka K, Nakagawa S, Mayumi T, Fujita T, Yamamoto A. Effects of lipofectin-antigen complexes on major histocompatibility complex class I-restricted antigen presentation pathway in murine dendritic cells and on dendritic cell maturation. Biochim Biophys Acta 2001; 1527: 97–101
- Mendoza L, Bubenik J, Simova J, Jandlova T, Vonka V, Mikyskova R. Prophylactic, therapeutic and anti-metastatic effects of BMDC and DC lines in mice carrying HPV 16-associated tumours. Int J Oncol 2003; 23: 243–247
- Hemmi H, Takeuchi O, Kawai T, Kaisho T, Sato S, Sanjo H, Matsumoto M, Hoshino K, Wagner H, Takeda K, Akira S. A Toll-like receptor recognizes bacterial DNA. Nature 2000; 408: 740–745
- Poltorak A, He X, Smirnova I, Liu MY, Van Huffel C, Du X, Birdwell D, Alejos E, Silva M, Galanos C, Freudenberg M, Ricciardi-Castagnoli P, Layton B, Beutler B. Defective LPS signaling in C3H/HeJ and C57BL/10ScCr mice: mutations in Tlr4 gene. Science 1998; 282: 2085–2088
- Banchereau J, Steinman RM. Dendritic cells and the control of immunity. Nature 1998; 392: 245–252
- Cui Z, Han SJ, Vangasseri DP, Huang L. Immunostimulation mechanism of LPD nanoparticle as a vaccine carrier. Mol Pharm 2005; 2: 22–28
- Heil F, Hemmi H, Hochrein H, Ampenberger F, Kirschning C, Akira S, Lipford G, Wagner H, Bauer S. Species-specific recognition of single-stranded RNA via toll-like receptor 7 and 8. Science 2004; 303: 1526–1529
- Lovgren T, Eloranta ML, Bave U, Alm GV, Ronnblom L. Induction of interferon-alpha production in plasmacytoid dendritic cells by immune complexes containing nucleic acid released by necrotic or late apoptotic cells and lupus IgG. Arthritis Rheum 2004; 50: 1861–1872
- Muzio M, Ni J, Feng P, Dixit VM. IRAK (Pelle) family member IRAK-2 and MyD88 as proximal mediators of IL-1 signaling. Science 1997; 278: 1612–1615
- Kaisho T, Takeuchi O, Kawai T, Hoshino K, Akira S. Endotoxin-induced maturation of MyD88-deficient dendritic cells. J Immunol 2001; 166: 5688–5694
- Kawai T, Takeuchi O, Fujita T, Inoue J, Muhlradt PF, Sato S, Hoshino K, Akira S. Lipopolysaccharide stimulates the MyD88-independent pathway and results in activation of IFN-regulatory factor 3 and the expression of a subset of lipopolysaccharide-inducible genes. J Immunol 2001; 167: 5887–5894
- Baker PJ, Hraba T, Taylor CE, Myers KR, Takayama K, Qureshi N, Stuetz P, Kusumoto S, Hasegawa A. Structural features that influence the ability of lipid A and its analogs to abolish expression of suppressor T cell activity. Infect Immun 1992; 60: 2694–2701
- Heumann D, Gallay P, Barras C, Zaech P, Ulevitch RJ, Tobias PS, Glauser MP, Baumgartner JD. Control of lipopolysaccharide (LPS) binding and LPS-induced tumor necrosis factor secretion in human peripheral blood monocytes. J Immunol 1992; 148: 3505–3512
- Celluzzi CM, Falo LD, Jr. Physical interaction between dendritic cells and tumor cells results in an immunogen that induces protective and therapeutic tumor rejection. J Immunol 1998; 160: 3081–3085