Abstract
This study aimed to determine the effects of dietary mannoheptulose (MH; 2 mg kg−1), a glycolytic inhibitor, on glucose oxidation and biomarkers of energy metabolism in neutered, adult, male Labrador Retriever dogs (N = 6). Fasting and post-prandial respiratory quotient (RQ) and energy expenditure (EE) were determined by indirect calorimetry (d 16). Glucose turnover and oxidation were assessed during fasting and repeated meal feeding using indirect calorimetry and a constant intravenous infusion of U-13C-glucose (d 18). A sample from the biceps femoris was obtained to determine the muscle protein content of adenosine monophosphate-activated protein kinase (AMPK) and acetyl CoA carboxylase (ACC; total and phosphorylated forms; d 21). MH did not affect EE, serum glucose, insulin or free fatty acids or ACC protein content. In fasting, MH significantly increased the fasting RQ (p = 0.01) and glucose oxidation (p = 0.01) and tended to decrease the ratio of phosphorylated to total AMPK protein content (p = 0.16). In contrast, post-prandial glucose oxidation tended to be lower (p = 0.14) in dogs fed MH. These results suggest that MH has differential effects on fasting and post-prandial whole body glucose and fat oxidation. However, further research that uses a greater number of animals and/or higher dose of MH is required.
1. Introduction
Calorie restriction (CR) without malnutrition (30–60% of ad libitum) has been shown to effectively delay the ageing process by increasing median and maximal lifespan and/or providing protection from age-related disease development in several species, including dogs and humans (Kealy et al. Citation2002; Roth et al. Citation2004; Fontana & Klein Citation2007). The mechanism by which CR exerts its beneficial effects is not completely understood. Current evidence suggests that CR impacts intracellular energy metabolism by altering key energy-sensing pathways leading to improved metabolic efficiency, enhanced insulin signalling and reduced oxidative damage (Fontana & Klein Citation2007).
Despite the reported benefits of CR, maintaining a low calorie diet remains a challenge for people and companion animals. The obesity epidemic plaguing the human population also impacts companion animals with 55% of dogs in the USA reported to be overweight or obese (Ward et al. Citation2011). Addressing this problem in pets has been challenging due to difficulties in defining the ideal weight for dogs, coupled with poor owner adherence to recommended feeding regimes. In addition, the perceived negative behavioural changes associated with CR (begging and aggression) has prompted researchers to seek alternative approaches to CR, such as CR mimetics.
CR mimetics are therapeutic agents or food ingredients that mimic the metabolic, hormonal and physiological effects of CR without altering caloric intake (Ingram et al. Citation2006). Mannoheptulose (MH), a seven carbon sugar found in high concentrations in avocados, has been proposed as a CR mimetic (Roth et al. Citation2009). Early research demonstrated MH functions as a glycolytic inhibitor through its ability to competitively inhibit hexokinases (HK, EC 2.7.1.1; Viktora et al. Citation1969). Dogs given MH doses ranging 1–2 g kg−1 (intravenous or intra-arterial) exhibit a transient diabetic state, characterized by marked hyperglycaemia, dramatically decreased insulin to glucagon ratio and increased hepatic glucose output (Viktora et al. Citation1969; Muller et al. Citation1971; Issekutz et al. Citation1974; Klain et al. Citation1976). These responses were well outside normal physiological ranges, suggesting that the dosage was supra-physiological. A recent report on Fox Terriers and Labrador Retrievers found that MH doses of 2, 10 and 20 mg kg−1 delivered in a gelatin capsule lowered fasting insulin concentrations with no change in the circulating glucose (Davenport et al. Citation2010). However, the effects of low oral doses of MH on other aspects of glucose metabolism are largely unknown. Furthermore, previous studies have only examined MH effects on fasting metabolism. As MH inhibits glucokinase in intestine, liver and pancreas, it is of interest to examine MH effects on post-prandial metabolism.
The objective of this pilot study was to determine the effects of low dietary doses of MH (2 mg kg−1) on glucose metabolism, specifically glucose oxidation. Given that MH inhibits glucokinase, we expected MH to decrease glucose utilization. In addition, we investigated the effects of MH on biomarkers of energy metabolism known to be associated with CR, including energy expenditure (EE) and 5′ adenosine monophosphate-activated protein kinase (AMPK) and acetyl CoA carboxylase (ACC) protein content in skeletal muscle.
2. Materials and methods
2.1. Animals and housing
All procedures were approved by the Institutional Animal Care and Use Committee of P&G Pet Care (Mason, OH, USA). A total of six neutered male Labrador Retrievers (three black-coated: 3.9 yr, 27.3 kg; and three chocolate-coated: 6.8 yr, 35.0 kg) were used in this study. All dogs were housed at the P&G Pet Health and Nutrition Center (Lewisburg, OH) and were considered healthy at the initiation of the study based on a general health evaluation by a licensed veterinarian. All dogs were at an optimal body condition score of 3.0 (5-point scale) at the initiation of the study. Dogs were pair-housed with free access to water and indoor and outdoor runs. Indoor runs were maintained on a 12 h light and dark cycle, in addition to natural light, and were equipped with raised canvas beds, toys and heated flooring. Outdoor runs were equipped with toys and play yard equipment. All dogs received 40 min of supervised group exercise and socialization in a separate fenced yard daily.
2.2. Study design
This study was designed as a crossover with each dog receiving both dietary treatments, control (CON) and MH, in random order. The duration of study was 44 d with each treatment leg (period) lasting 22 d. For each treatment leg, blood parameters, indirect calorimetry and glucose kinetics were measured and muscle biopsies were collected. The indirect calorimetry method only allowed three dogs to be measured per day. Therefore, dogs were blocked by coat colour (i.e. chocolate and black) for all collections and were staggered one day apart irrespective of their dietary treatment.
2.3. Diets and feeding
Test diets were formulated to represent Eukanuba Large Breed Senior Maintenance Formula, which is a commercially available diet that is nutritionally complete and balanced. Test diets were similar in overall nutrient content (92% dry matter; 24% protein; 15% fat; 43% carbohydrate; ME 3.17 kcal g−1). The MH diet was formulated with avocado whole fruit extract to supply 200 mg kg−1 MH. The MH-enriched avocado extract was produced using commercially available avocados (Hass variety). Frozen, whole avocados comprising the flesh, peel and pit were initially ground before suspension in water (1:3 w/w). The resultant slurry was centrifuged to remove non-aqueous solids. A series of microfiltration (de-oiling), ultrafiltration (10 kDa) and nanofiltration (100 kDa) was used to produce the MH-enriched fraction. Lyophilization was used to form the final crystalline powder yielding 18% MH.
Dogs were fed to maintain body weight, based on their historical caloric intake records (CON: 2066 ± 191 kcal d−1; MH: 2111 ± 196 kcal d−1). For one week prior to study initiation, dogs were fed the CON diet (‘wash-in’ period). Dogs were individually fed their daily ration in two meals (0800 and 1300) to deliver a daily dose of 2 mg kg−1 MH. As mentioned previously, a dose of 2 mg kg−1 was shown to decrease circulating insulin, but not glucose, in Labrador Retrievers (Davenport et al. Citation2010). The dose therefore, appears to be bioavailable and non-pharmacological.
2.4. Measurements
Food intake was measured daily and body weight weekly. On d 1 (baseline), 7 and 14 of each treatment leg, blood samples (each 3 mL) were obtained via jugular venipuncture prior to the dog's morning meal (fasting) and again precisely 4 h after the morning meal. All dogs were acclimated to the venipuncture procedure prior to study. Fasting blood samples were analysed for glucose, insulin and free fatty acids. Only plasma MH concentrations were measured in the fed samples to confirm that dogs received the correct dietary treatment.
2.5. Indirect calorimetry
Respiratory gas exchange measurements were conducted via whole body indirect calorimetry on d 16 of each treatment leg. The calorimetry chambers (76 cm × 53 cm × 61 cm, L × W × H) were made of clear plexiglass and fitted with a hinged access top door or feeding drawer for providing food to the dog. Chambers were designed as open-flow circuits with room air pulled into the chambers at a rate of 22–26 L min−1 to maintain CO2 levels in the chamber between 0.4% and 0.8%. The exiting chamber air was dried by passing it through columns of Drierite™ and magnesium perchlorate before reaching the O2 and CO2 analysers (Qubit Systems Inc., Kingston, ON, Canada). Each chamber was sampled every three seconds over a 5-min period. O2 and CO2 exchange and respiratory quotient (RQ) data were logged using data acquisition software (Qubit Systems Inc., Kingston, ON, Canada). Prior to study initiation, dogs were acclimated over an eight-week period (minimum exposure of 1 h per week) to rest comfortably in the chamber with no excessive activity or movement and were discouraged from urinating or defecating in the chamber.
Prior to any measurements, the O2 and CO2 analyzers were calibrated and dogs rested in the chamber for a minimum of 25 min to ensure adequate CO2 accumulation. Gas exchange measurements consisted of two fasting collections occurring 18 h after the dog's last meal (time –50 min and –25 min). Dogs were then fed their full daily ration of test diet as a single meal (considered time 0). After feeding, gas exchange measurements were collected every 25 min for 12 h. Dogs were provided a brief 10 min break at 6 h post-feeding in which they were removed from the chamber, taken outdoors into a fenced area and provided an opportunity to urinate and defecate. During this break, the O2 and CO2 analyzers were recalibrated. After the break, dogs rested in the calorimetry chambers for a minimum of 25 min to ensure adequate CO2 accumulation prior to the resumption of gas exchange measurements. EE was calculated from O2 consumption and CO2 production using the abbreviated de Wier equation (de Wier Citation1949) and expressed on a per kg metabolic BW basis (BW0.75).
2.6. Glucose kinetics
Glucose kinetics were assessed during fasting and repeated meal feeding (fed state) on d 19 of both treatment legs using indirect calorimetry to measure CO2 exchange and a primed, continuous intravenous infusion of U-13C-Glucose (99% atom, Sigma Aldrich, Isotec, Miamisburg, OH, USA, 45342) to quantify glucose flux. Both front legs were aseptically prepared and an 18 gauge catheter was inserted into the cephalic vein of each leg. One catheter was used for isotope infusion, while the other catheter was only used if that catheter failed. All blood samples were taken by jugular venipuncture.
Animals began measurements in the fasted state (18 h since last meal). Baseline breath and blood samples were collected prior to isotope infusion to determine background 13C enrichment. At time 0, a bolus dose (14 mg kg−1 BW) of isotope was administered, followed immediately by a continuous intravenous infusion (0.025 mg kg−1 min−1) delivered via the cephalic catheter using an NE-1000 Syringe Pump (New Era Pump Systems Inc., Farmingdale, NY, USA). Six fasting blood samples (each 3 mL) were taken at 60, 85, 110, 135, 160 and 185 min followed by four breath samples collected at 220, 245, 270 and 295 min post-infusion. After the completion of fasting measurements, fed-state kinetics were examined. Dogs were fed their test diet divided into 15 equal meals (14 g kg−1 per meal). Meals were initially fed three times, 10 min apart and then every 25 min thereafter. This feeding regime was based on reported gastric emptying time of ~20 min for dogs fed small meals (Gooding et al. Citation2012). Therefore, it was assumed that feeding small meals in 25 min intervals would mimic a continuous fed state and provide a constant dietary infusion of MH. Blood and breath collections were taken at the same time points as fasting measurements, with additional two breath collections at 320 and 345 min.
Blood samples were centrifuged for 8 min at 3000×g at 7°C and the serum and plasma were stored separately at –80°C for subsequent analysis of glucose enrichment. Expired CO2 was trapped in 1 mol/L NaOH and stored at room temperature for later analysis of 13C enrichment.
Plasma glucose turnover and whole body glucose oxidation were calculated according to Bergman's minimal model (Bergman et al. Citation1979). Plasma glucose rate of appearance (Ra) was assumed to equal the rate of disappearance at isotopic steady state (S/S) and represent whole body plasma glucose turnover (1):
The rate of whole body glucose oxidation (Ox) was calculated according to Equation (2):
If a plateau in isotopic enrichment did not occur, S/S was extrapolated by using simple negative exponential curve-fitting over the period of post-peak decline or by using Equation (3):
2.7. Muscle biopsy
All six dogs underwent muscle biopsies on the same day (d 20 or 21 of both treatment legs). Dogs were fasted overnight and sedated using dexmedetomidine (0.02 mg kg−1, Dexdomitor®, Pfizer) and Carprofen (4 mg kg−1, Rimadyl®, Pfizer). Propofol (5–7 mg kg−1, Propoflo®, Abbott) was administered intravenously for induction in Treatment Leg 1. As Propofol was unavailable for purchase due to a worldwide shortage in Treatment Leg 2, ketamine (5.5 mg kg−1) and diazepam (0.275 mg kg−1) were used instead. The use of different induction chemicals was not believed to affect any outcome measures, as each dog received both agents as part of the crossover design. A 3–4 cm incision was made along the dorsal caudal thigh and a no. 10 scalpel blade was used to remove two muscle tissue samples (wet weight ~100 mg per sample) from the biceps femoris. The incision was closed using surgical skin staples (AutoSuture Appose ULCTM, Tyco). At the end of the procedure, each dog was administered atipamezole (0.125 mg kg−1, Antisedan®, Pfizer) as a sedative reversal and placed in a heated cage until recovered. Dogs were monitored for one week for complications. Muscle samples were immediately frozen in liquid N2 and stored at –80°C for later analysis of phosphorylated (p) and total (t) ACC and AMPK protein abundance.
Muscle was homogenized in cold NP40 Cell Lysis Buffer with 1 mmol L−1 PMSF (Invitrogen Corp., Camarillo, CA) and protease inhibitor cocktail (Sigma, Oakville, ON), and centrifuged at 4°C for 30 min. Resulting supernatants were removed and protein concentration was determined by Bradford protein assay. Protein (30 µg) from muscle lysates was diluted (1 v/v) in Laemmli sample buffer (Bio-Rad Laboratories, Mississauga, ON), loaded onto 7.5% Tris HCL pre-cast resolving gels (Bio-Rad Laboratories, Mississauga, ON) and separated by SDS-PAGE (1 h, 150 v). Gels were equilibrated in cold transfer buffer [25 mmol L−1 Tris, 192 mmol L−1 glycine and (20% (v/v) methanol, pAMPK and AMPK only)] and transferred onto nitrocellulose membranes (Bio-Rad Laboratories, Mississauga, ON). Membranes were incubated at 4°C for a minimum of 8 h with either ACC, pACC, AMPK or pAMPK antibody (Cell Signaling Technology Inc., Boston, MA) diluted (1:1000) in 7.5% BSA. Membranes were washed in 1} TBST and then incubated with anti-rabbit immunoglobulin–horseradish-peroxidase-linked whole antibody (1:2000 dilution in TBST) for ~1 h. Washed membranes were submerged in enhanced chemiluminescence reagents (GE Health Care Biosciences Corp., Piscataway, NJ) and exposed using an Alpha Innotech imager. Bands were visualized and quantified using AlphaView Software for FluorChem Systems. Data are expressed as the ratio of phosphorylated (p) to total protein content.
2.8. Biochemical analyses
Serum glucose was measured by a clinical chemistry analyzer (Beckman Coulter AU480). Serum insulin was assayed using a paramagnetic particle, chemiluminescent immunoassay (Beckman, Fullerton, CA) and serum free fatty acids using an enzymatic calorimetric assay (Wako Diagnostics). HPLC tandem mass spectrometry was utilized to determine plasma MH. Briefly, 200 µL of serum was diluted with 800 µL MeCN and 10 µL of internal standard solution (13C7 D-MH, Toronto Research Chemicals, Inc.) and sonicated for 5 min, followed by centrifugation for 5 min at 3750 rcf. The supernatant (10 µL) was injected onto a Shodex NH2P-40 3E column (Showa Denko America Inc.), with guard on a Shimadzu LC10AVP HPLC (flow rate 300 µL min−1, column temperature 50°C). Mobile phase A was 61% acetonitrile, 39% water with 10 mmol L−1 formic acid. Mobile phase B was 50% acetonitrile, 50% water with 10 mmol L−1 formic acid. The gradient was 100% A isocratic for 43 min followed by a 6-min gradient to 100% B. After a 6-min hold at 100% B, the system was equilibrated at 100% A for 12 min. MS/MS was performed in a negative ionization mode on a Sciex API 4000 MS/MS. For MH, the formic acid loss was monitored at m/z 255–209. For C7 MH, the formic acid loss was monitored at m/z 262–216. Plasma isotope enrichment was measured by Metabolic Solutions Inc. (Nashua, NH) using the method described by Previs et al. (Citation1994). Briefly, the isotopic enrichment of plasma samples was determined by gas chromatography–mass spectrometric analysis of the glucose aldonitrile pentaacetate derivative formed with acetic anhydride and hydroxylamine hydrochloride. 13C6-glucose enrichment was measured using an Agilent 5975C EI/CI MSD with an Agilent 7890 GC in the electron ionization mode. A Phenomenex ZB-1MS capillary column was used to separate the derivative of glucose. Selected ion chromatograms were obtained by monitoring ions m/z 328 and 334 for D-glucose and D-[13C6]-glucose, respectively. Isotope enrichment in mole % excess was calculated from peak area ratios. The final value for all determinations was corrected using an enrichment calibration curve. Breath samples were analysed using an automated 13CO2 breath analysis system, BreathMat Plus (Thermo Finnigan, San Jose, CA; Phillips et al. Citation2004). Briefly, 2 mL of the expired breath sample were introduced into the gas chromatograph. Gases not of interest (N2 and O2) as well as water were removed online via a continuous-flow diffusion pump. Pure CO2 was introduced to the isotope ratio mass spectrometer (IRMS) and was analysed at a mass-to-charge ratio of 44:45.
2.9. Statistical methods
All data were analysed using SAS version 9.2 (SAS Institute, Cary, NC) and expressed as mean with pooled standard error. Data were analysed using the proc mixed procedure with treatment leg (period) and diet considered fixed effects and dogs random variables. Repeated measures within period on dogs were subjected to the autoregressive order 1 covariance structure. For calorimetry data, time was considered a fixed effect and fasting, post-prandial (0–6 h) and post-absorptive (7–12 h) data were analysed separately. Statistical significance was considered as p < 0.05. The interactions between fixed effects were also tested but reported only if significant.
3. Results
3.1. Macronutrient oxidation
Fasting RQ was significantly (p = 0.0102) greater in dogs fed MH (). However, there was no effect of diet on post-prandial RQ (). An isotopic S/S was not achieved in breath during fasting, and glucose oxidation was calculated using extrapolated values (derived from Equation (4)). Fasting glucose oxidation was significantly (p = 0.0182) higher in dogs fed MH than those that received the control (). During repeated meal feeding, plateaus in isotope enrichment were achieved and considered as S/S values. While there was no statistically significant difference in post-prandial glucose oxidation, it was numerically lower in dogs that consumed the MH-containing diet ().
Table 1. Fasting RQ, EE, plasma glucose turnover (Ra), and glucose oxidation in adult male neutered Labrador Retrievers receiving either a control diet or mannoheptulose (MH) containing diet (2 mg kg−1). Post-prandial plasma glucose turnover and glucose oxidation data are also presented. Data are means with pooled standard error and N = 6 in a complete cross-over design.
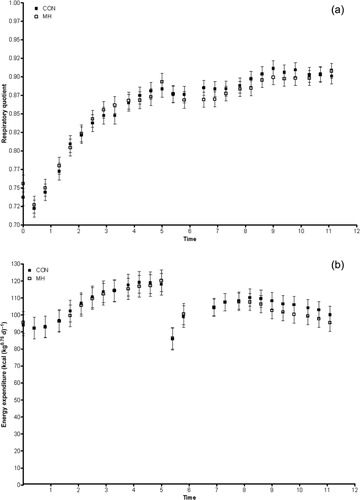
3.2. Glucose metabolism
There were no significant changes in fasting glucose (), insulin () or free fatty acids () over time, and there was no effect of diet (or period). True isotopic S/S was not achieved in plasma during fasting or repeated meal-feeding likely due to an over prime of isotope. Therefore, plasma glucose turnover was calculated using extrapolated S/S values. Fasting and post-prandial plasma glucose turnover was not affected by the diet ().
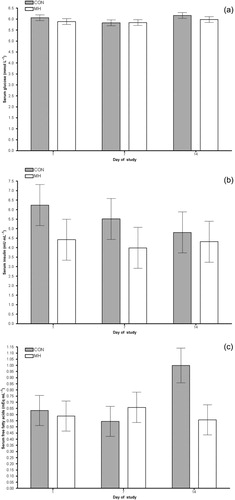
3.3. Energy metabolism
Fasting () and post-prandial EE () were not affected by the diet. Dogs receiving MH tended to have a lower ratio of phosphorylated to total AMPK protein content (p = 0.1634; ). MH did not affect the ratio of phosphorylated to total ACC ().
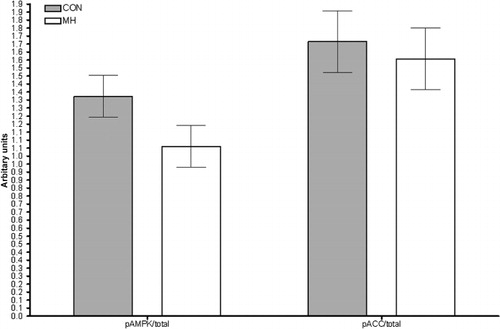
4. Discussion
Overall, MH did not affect the circulating glucose, insulin or free fatty acids or EE. However, MH significantly increased RQ and glucose oxidation in fasting and tended to lower post-prandial glucose oxidation. In addition, MH tended to decrease the ratio of phosphorylated to total AMPK protein content in fasted skeletal muscle, suggesting that fat utilization was decreased (indirect evidence). Together these results suggest that MH shifts the time course of glucose utilization from after a meal to the fasting period. However, a study using a greater number of animals and/or higher dose of MH is needed to fully understand the effects of dietary MH on glucose and energy metabolism.
The ability of MH to induce transient metabolic changes has been demonstrated in rats, dogs and humans with effects lasting from one to four hours after MH administration (Johnson & Wolff Citation1970; Muller et al. Citation1971; Klain & Meikle Citation1974; Klain et al. Citation1976). In contrast to the present study, these studies examined the effects of MH exclusively in the fasted state using very high doses of MH administered intravenously (or intra-arterially). These conditions elicited responses well outside the normal physiological ranges, suggesting that the dosage was supra-physiological. In addition, injecting MH does not allow one to examine the potential effects of MH on gut and/or liver metabolism (i.e. first-pass responses). Nevertheless, the patterns of change in glucose oxidation (fast vs. fed) induced by dietary exposure in our study are consistent with the temporary changes in metabolism observed in these previous reports.
This study was the first to examine in vivo glucose oxidation in response to dietary MH and statistically significant differences between groups were achieved in fasting only. The lack of significance during repeated meal feeding was likely due to a lack of statistical power and future studies with larger sample sizes are warranted. Similarly, glucose oxidation and turnover rates were calculated using extrapolated S/S enrichment values. While fasting plasma extrapolated S/S enrichments were comparable to reported literature values (Coggan et al. Citation1990; Tounian et al. Citation1994), 13CO2 enrichment has been poorly reported in the literature, making it difficult to compare extrapolated S/S breath enrichment values. While fasting oxidation values in this study were lower than those previously reported in mongrel dogs (~0.89 mg kg−1 min−1 Wolfe & Shaw Citation1986; ~1.19 mg kg−1 min−1; Paul et al. Citation1966), they are still within the normal physiological range. As the repeated meal feeding technique has not been used to study glucose turnover or oxidation, we did not have comparable literature data. However, the repeated meal feeding technique was essential to this study as it provided a constant dietary supply of MH and mimicked a continuous fed state. The fed oxidation values were similar to those observed in humans under clamped hyperinsulinaemic conditions (Bonadonna et al. Citation1993; Fery et al. Citation2004).
In contrast to earlier studies, MH did not affect circulating glucose or insulin. The lack of consensus may be due to the major methodological differences between these early reports and our study which have been already noted. The only other published report of oral MH administration observed lower fasting insulin but no change in glucose in Fox Terriers and Labrador Retrievers given 2 mg kg−1 MH for 30 days (Davenport et al. Citation2010). These findings suggest that the dose of MH used in the present study should have been sufficient to elicit changes in circulating insulin levels. However, previous reports demonstrate that MH has a greater impact on circulating glucagon than insulin levels. Indeed, a fivefold increase in glucagon has been reported in dogs during constant MH infusion (Muller et al. Citation1971). As such, it would be beneficial for future studies to examine MH effects on both insulin and glucagon.
MH tended to decrease the ratio of phosphorylated to total AMPK protein content. AMPK is considered a master regulator of intracellular energy metabolism (Ruderman & Prentki Citation2004). When activated (via phosphorylation), AMPK increases cellular energy generation mainly by promoting lipid oxidation but also by increasing glucose uptake (Ruderman & Prentki 2004). The observed decrease in active AMPK indirectly suggests that fat utilization was decreased in fasting. This notion compliments our findings that RQ and glucose oxidation were increased in fasting.
In conclusion, MH at a dose of 2 mg kg−1 increased fasting glucose oxidation and RQ but did not appear to significantly affect post-prandial glucose or energy metabolism. Future studies using larger sample sizes and/or higher doses of MH, coupled with direct measurements of fat oxidation are required. Furthermore, the effects of MH on other proteins known to be associated with CR and cellular fuel selection are needed to better characterize the effects of low doses of dietary MH.
Notes on contributors
E.A.F, G.M.D and A.K.S. are employees of P&G Pet Care.
Acknowledgements
The authors would like to thank the staff at the P&G Pet Health and Nutrition Centre (Lewisburg OH), especially Lisa Fortener and Liz Fuess for their technical assistance. In addition, the authors would like to thank Laelie Snook, Department of Human Health and Nutritional Sciences, University of Guelph, for her technical assistance and Dr David Wright, Department of Human Health and Nutritional Sciences, University of Guelph, for providing laboratory space and contributing to the interpretation of the immunoblot results.
Funding
This work was supported by P& G Pet Care.
Additional information
Funding
References
- Bergman RN, Ider YZ, Bowden CR, Cobelli C. 1979. Quantitative estimation of insulin sensitivity. Am J Physiol. 236:E667–E677.
- Bonadonna RC, Del Prato S, Bonora E, Gulli G, Solini A, DeFronzo RA. 1993. Effects of physiological hyperinsulinemia on the intracellular metabolic partition of plasma glucose. Am J Physiol. 265:E943–E953.
- Coggan AR, Kohrt WM, Spina RJ, Bier DM, Holloszy JO. 1990. Endurance training decreases plasma glucose turnover and oxidation during moderate-intensity exercise in men. J Appl Physiol. 68:990–996.
- Davenport G, Massimino S, Hayek M, Burr J, Ceddia M, Yeh C-H, Roth G, Ingram D. 2010. Biological activity of avocado-derived mannoheptulose in dogs. FASEB J. 24:725.4.
- de Wier JB. 1949. New methods for calculating metabolic rate with special reference to protein metabolism. J Physiol. 109:1–9.
- Fery F, Tappy L, Deviere J, Balasse EO. 2004. Comparison of intraduodenal and intravenous glucose metabolism under clamp conditions in humans. Am J Physiol. 286:E176–E183.
- Fontana L, Klein S. 2007. Aging, adiposity, and calorie restriction. J Am Med Assoc. 297:986. 10.1001/jama.297.9.986
- Gooding MA, Duncan IJH, Atkinson JL, Shoveller AK. 2012. Development and validation of a behavioural acclimation protocol for cats to respiration chambers used for indirect calorimetry studies. J Appl Anim Welfar Sci 15:144–162. 10.1080/10888705.2012.658332
- Ingram DK, Zhu M, Mamczarz J, Zou S, Lane MA, Roth GS, deCabo R. 2006. Calorie restriction mimetics: an emerging research field. Aging Cell. 5:97–108. 10.1111/j.1474-9726.2006.00202.x
- Issekutz,Jr B, Issekutz TB, Elahi D. 1974. Effect of mannoheptulose on glucose kinetics in normal and glucocorticoid treated dogs. Life Sci. 15:635–643. 10.1016/0024-3205(74)90503-7
- Johnson BF, Wolff FW. 1970. Trial of mannoheptulose in man. Metabolism. 19:354–362. 10.1016/0026-0495(70)90132-0
- Kealy RD, Lawler DF, Ballam JM, Mantz SL, Biery DN, Greeley EH, Lust G, Segre M, Smith GK, Stowe HD. 2002. Effects of diet restriction on life span and age-related changes in dogs. J Am Vet Med Assoc. 220:1315–1320. 10.2460/javma.2002.220.1315
- Klain GJ, Meikle AW. 1974. Mannoheptulose and fatty acid synthesis in the rat. J Nutr. 104:473–477.
- Klain GJ, Meikle AW, O'Barr TP. 1976. Gluconeogenic response to mannoheptulose in the rat. J Nutr. 106:68–72.
- Muller WA, Faloona GR, Unger RH. 1971. The effect of experimental insulin deficiency on glucagon secretion. J Clin Invest. 50:1992–1999. 10.1172/JCI106691
- Paul P, Issekutz, Jr B, Miller HI. 1966. Interrelationship of free fatty acids and glucose metabolism in the dog. Am J Physiol. 211:1313–1320.
- Phillips SM, Stewart BG, Mahoney DJ, Hicks AL, McCartney N, Tang JE, Wilkinson SB, Armstrong D, Tarnopolsky MA. 2004. Bodyweight-support treadmill training improves blood glucose regulation in persons with incomplete spinal cord injury. J Appl Physiol. 97:716–724. 10.1152/japplphysiol.00167.2004
- Previs SF, Ciraolo ST, Fernandez CA, Beylot M, Agarwal KC, Soloviev MV, Brunengraber H. 1994. Use of [6,6-2H2]glucose and low-enrichment [U-13C6]-glucose for sequential or simultaneous measurements of glucose turnover by gas chromatography-mass spectrometry. Anal Biochem. 218:192–196. 10.1006/abio.1994.1159
- Roth G, Hayek M, Massimino S, Davenport G, Arking R, Bartke A, Bonkowski M, Ingram D. 2009. Mannoheptulose: glycolytic inhibitor and novel caloric restriction mimetic. FASEB J. 23:553.1. 10.1096/fj.09-0101ufm
- Roth GS, Mattison JA, Ottinger MA, Chachich ME, Lane MA, Ingram DK. 2004. Aging in Rhesus monkeys: relevance to human health interventions. Science. 305:1423–1426. 10.1126/science.1102541
- Ruderman N, Prentki M. 2004. AMP kinase and malonyl-CoA: targets for therapy of the metabolic syndrome. Nat Rev Drug Discov. 3:40–51.
- Tounian P, Schneiter P, Henry S, Jfiquier E, Tappy L. 1994. Effects of infused fructose on endogenous glucose production, gluconeogenesis, and glycogen metabolism. Am J Physiol. 267:E710–E717.
- Viktora JK, Johnson BF, Penhos JC, Rosenberg CA, Wolff FW. 1969. Effect of ingested mannoheptulose in animals and man. Metabolism. 18:87–102. 10.1016/0026-0495(69)90101-2
- Ward E, Budsberg S, Lund E. 2011. Fat pets getting fatter according to latest survey. Association for Pet Obesity Prevention. Available from: http://www.petobesityprevention.com/fat-pets-getting-fatter-according-to-latest-survey/
- Wolfe RR, Shaw JHF. 1986. Effect of epinephrine infusion and adrenergic blockade on glucose oxidation in conscious dogs. Metabolism. 35:673–678. 10.1016/0026-0495(86)90177-0