ABSTRACT
Muscle fiber is the basic unit of skeletal muscle. Muscle fiber types play a crucial role in improving meat quality. In the current study, Wistar rats were chronic injected with 5-aminoimidazole-4-carboxamide-1-β-D-ribofuranoside (AICAR) for four weeks in order to define the 5′-AMP-activated protein kinase (AMPK) protein activity on muscle fiber composition, enzyme activity and the mRNA expression of muscle fiber type-related genes in gastrocnemius (GS) muscle. Food intake and body weight did not show a significant difference after AICAR treatment (P > .05). The AICAR treatment caused a significantly increased number and area proportion of type I muscle fiber and expression level MyHC I mRNA (P < .05), succinic dehydrogenase (SDH) and malate dehydrogenase (MDH) activities (P < .05), but decrease in the number proportion of type IIB muscle fiber and MyHC IIb and MyHC IIx mRNA level (P < .05). The Peroxisome proliferator-activated receptor-γ coactivator-1α (PGC-1α) mRNA level (P < .05) was increased and glucose transporter 4 (GLUT-4) mRNA level tended to be higher (P = .052) in the AICAR group. These results demonstrated that chronic AICRA treatment induces oxidative muscle fiber switching, enhances oxidative enzyme activity and GLUT4 mRNA level, mediated by PGC-1α.
1. Introduction
Skeletal muscle consists of different types of muscle fibers and has a strong plasticity (Herbison et al. Citation1982; Hoh Citation1992; Nakazato and Tsutaki Citation2012). Skeletal muscle can undergo dramatic biochemical and physical changes under the external stimuli. Four isoforms of major myosin heavy chain (MyHC) are detected in mammalian skeletal muscle, namely MyHC I, MyHC IIa, MyHC IIx and MyHC IIb, fundamental determinants of muscle fiber’s contractile properties. They are present in different muscle fibers, which leads to differences in the speed of contraction relating to the corresponding type of energy metabolism (Pette and Staron Citation1997). Based on the contractile properties of skeletal muscle, muscle fiber types are divided into type I and type II. Type I muscle fibers include MyHC I, which contract relatively slowly. And due to the richness of capillaries, mitochondria, cytochrome cytochromes, myoglobin and major oxidative metabolic enzymes, the type I fibers are also called slow muscle fibers, oxidized muscle fibers or red muscle fibers (Pette and Staron Citation2000; Schiaffino and Reggiani Citation2011). The remaining three isoforms of MyHC were included in type II muscle fibers, which contract relatively fast, with less mitochondria and myoglobin. In addition, the main metabolic pathway of type I fibers is glycolysis metabolic, thereby type II fibers are called fast muscle fibers, glycolysis muscle fibers or white muscle fibers (Pette and Staron Citation1997, Citation2000; Schiaffino and Reggiani Citation2011).
Several studies showed muscle fiber type composition is also related to the meat quality. A study in Simmental hybrids found that MyHC I and MyHC IIa were negatively correlated with and intramuscular fat (IMF) content and meat shearing force (MSF), MyHC IIx was inverse associated with MSF(Hatakeyama and Kanzaki Citation2013). These data suggested that MyHC gene may be an inverse factor for IMF content and MSF. Muscles with higher proportion of type IIB fiber showed faster initial pH decline and lighter surface (Choi et al. Citation2016). In sensory evaluation studies, the ratio of myosin heavy chain (MHC) fast/slow was negatively correlated with the juiciness and tenderness, was positively correlated with the off-flavour of Berkshire pigs, suggesting that MHC isoforms had an effect on meat quality in Berkshire pigs (Kang et al. Citation2011). These results revealed that muscle fiber types had great influence on meat quality. Therefore, establishing a method to regulate the composition of muscle fiber types could help control meat quality.
Skeletal muscle can be stimulated by a variety of physiological and external factors to change structural and functional characteristics (Rockl et al. Citation2007; Nakazato and Tsutaki Citation2012). A multitude of important signalling pathways regulated the metabolic capabilities of skeletal muscle, such as calcium signalling, 5′-AMP-activated protein kinase (AMPK) signalling, miRNA/anti-sense RNA regulations, etc. (Nakazato and Tsutaki Citation2012). AMPK is an important signalling molecule for muscle cells to obtain energy (Hardie et al. Citation1998). AMPK can be activated by 5-aminoimidazole-4-carboxamide-1-β-d-ribofuranoside (AICAR), which may cause muscle fiber transformation from fast to slow (Green et al. Citation1992; Suwa et al. Citation2003). Consistent with the conversion of muscle fiber from fast type to slow type, the expression of glucose transporter 4 (GLUT4) and Peroxisome proliferator-activated receptor-γ coactivator-1α (PGC-1α) were phenomenal improved when AMPK was activated, while oxidized and glycolytic enzyme activity changed (Suwa et al. Citation2003; Chen et al. Citation2018). PGC-1α, a transcriptional coactivator, regulate mitochondrial biogenesis (Lin et al. Citation2002; Scarpulla Citation2002; Yan et al. Citation2011; He et al. Citation2015), promoted GLUT-4 gene expression (Michael et al. Citation2001), and induce muscle fiber type switching (Ying et al. Citation2016). PGC-1α can regulate the oxidative capacity and muscle fiber type transformation of skeletal muscle by regulating the expression of Myocyte enhancer factor 2C (MEF2C) (Lin and Liang Citation2002). The evidence suggests a major role of AMPK and PGC-1α for muscle fiber type transformation.
The purpose of the present study was to define the influence of AMPK on muscle fiber characteristics, the mRNA level of MyHC, PGC-1α, GLUT-4, MEF2C and enzyme activation in skeletal muscle of Wistar rats using AMPK agonist AICAR for 4 weeks. To estimate whether the AICAR treatment would affect the transformation of muscle fibers, meanwhile alter activities of the related metabolic enzyme.
2. Materials and methods
2.1. Animal treatment and care
A total of 26 female Wistar rats with an average body weight of 65.50 ± 3.71 g at the age of 3 weeks from Animal Experiment Center of Inner Mongolia University were (Hohhot, Inner Mongolia, China) by divided into control (n = 13) and AICAR treatment (n = 13) groups. Rats were housed in individual cages with an ambient humidity of 55 ± 5%, a temperature 22 ± 2°C. Rats were exposed to light for 12 h a day, protected from light for 12 h a day. Food and water were provided ad libitum until the end of the experiment. Rats in the AICAR treatment group were injected with AICAR subcutaneously (0.5 mg/kg body weight, Toronto Research Chemicals, North York, ON, Canada), and rats in the control group were injected with saline. Before starting the experiment, all rats were pre-experimented for 7 days in order to adapt to the experimental environment and procedures. The period of this experiment was 4 weeks. The food intake and the body weight gain were recorded every day.
2.2. Sample collection
Immediately, the gastrocnemius (GS) muscle was collected for biochemical analyses. A part of the sample was immediately frozen in liquid nitrogen and stored at −80 °C for western blotting, RNA extraction and enzyme activity measurement. Another part of the sample was cut into 0.5 cm × 0.5 cm × 1.0 cm blocks, then the pieces were dehydrated in pre-cooled isopentane for 30 s and immediately frozen in liquid nitrogen and stored at −80 °C for muscle fiber characteristics analysis.
2.3. Muscle fibre characteristics
Transverse serial sections (10 μm) were obtained from blocks using a cryostat microtome (MEV, SLEE, Germany) at −25 °C. Then sections were incubated using staining methods of myosin adenosine triphosphatase (mATPase) (pH 4.60) to classify the muscle fiber types (Brooke and Kaise Citation1970). Approximately 1500 fibers per sample, which randomly selected from no tissue disruption and freeze damage, were detected and defined as fiber type I, fiber type IIA, fiber type IIB. All samples were analysed by image analysis program (Laica QWin V3 Processing-Analysis Software, Leica, Germany). The cross-sectional area (CSA) of the muscle fiber was determined as the ratio of the total measured area to the total measured number of fibers count.
2.4. Western blotting
Preparation of homogenates, determination of protein concentration and immunoblotting were performed as described recently in detail (Su et al. Citation2019). Briefly, the homogenized proteins were separated by SDS–PAGE using a 10% polyacrylamide separating gel and a 5% stacking gel. The proteins were transferred to a polyvinylidene fluoride membrane (Millipore, Eschborn, Germany) and incubated with primary antibodies AMPK, dilution 1:1000, Abcam, Cambridge, MA, USA, Ab131512; p-AMPK, dilution 1:1000, CST, Danvers, MA, USA, 4184S; ACC, dilution 1:1000, CST, Danvers, MA, USA, 4190S; p-ACC, dilution 1:1000, Abcam, Cambridge, MA, USA, Ab31931; β-actin, dilution 1:500, Boster Biological Technology, Wuhan, China, BM0627). Nitrocellulose membranes were washed, and subsequently incubated for 1 h at room temperature with the secondary antibody (mouse anti-goat, dilution 1:5000; Boster Biological Technology, Wuhan, China, BA1051; rabbit anti-goat, dilution 1:5000; Boster Biological Technology, Wuhan, China, BA1054). The blots were developed with an E-Gel imaging system (Thermo, Beijing, China), and band intensities were analysed using ImageJ software (https://imagej.nih.gov/ij/). β-Actin as a reference protein.
2.5. Enzyme activity
The activities of succinic dehydrogenase (SDH) (Code No. A022), malate dehydrogenase (MDH) (Code No. A021-2) and lactate dehydrogenase (LDH) (Code No. A020-2) and the total protein content in GS of rats were evaluated using kits from Nanjing Jiancheng Bioengineering Institute of China (Nanjing, Jiangsu, China). Enzyme activities were represented by U/mgprot.
2.6. RNA extraction and RT–PCR
Total RNA was extracted utilizing RNAiso Plus reagent (Code No. 9109, Takara, Dalian, China). The concentration and purity of the total RNA were calculated using BioDrop μLite (BioDrop, Cambridge, England). The PrimeScript™ RT reagent Kit with gDNA Eraser (Perfect Real Time) (Code No. RR047, Takara, Dalian, China) was used for reverse transcription for the first-strand cDNA. The mRNA expression levels of all genes were measured by RT–PCR using TB GreenTM Premix Ex Taq™II (Tli RNaseH Plus) (Code No. RR820, Takara, Dalian, China). The 18S gene was used as the internal reference for the evaluation of the MyHC I, MyHC IIa, MyHC IIb, MyHC IIx, PGC-1α, MEF2C and GLUT4 genes. The primer sequences used are shown in . The delta-Ct method and PCR data analysis program were used to quantify the Ct values. For all the treated samples, the evaluation of 2-ΔΔCt indicated the fold change in gene expression relative to the control.
Table 1. Primers for cloning and RNA quantitative assay.
2.7. Statistical analysis
Results were expressed as means ± standard errors. Statistical analysis was performed using IBM SPSS Statistics 19.0. We used the one-way analysis of variance followed by unpaired Tukey's test to compare data between groups with a P-value <.05 considered statistically significant.
3. Results
3.1. Food intake and body weight
The results of food intake and body weight are shown in . Food intake and body weight were no statistically significant differences between the AICAR group and control group (P > .05). That suggests that AICAR treatment did not cause the changes in food intake and body weight of rats.
3.2. AMPK phosphorylation
As shown in , compare with the control group, AMPK phosphorylation was increased 2.25-fold in GS muscle after the AICAR injection (P < .01). Because AMPK activity was regulated by phosphorylation, current data sufficient to explain that AMPK was activated after AICAR injection. The phosphorylation of ACC at Ser79 is only phosphorylated by AMPK; therefore, this phosphorylation is usually used to evaluate AMPK activity (Thomson et al. Citation2008; Han et al. Citation2010). Consistently, ACC phosphorylation was increased in the AICAR group, which was highly correlated with AMPK phosphorylation ().
3.3. Muscle fibre characteristics
Representative photomicrographs of the control group and AICAR group are shown in . Muscle fibers were classified into type I (black), type IIA (white) and type IIB (brownness) by staining method of myosin ATPase. The results for muscle fiber characteristics are shown in , AICAR treatment increased number and area proportion of type I muscle fiber (P < .05), decreased number proportion of type IIB muscle fiber (P < .05). No effect on the CSA and diameter of fibers type I, IIA and IIB by AICAR treatment.
Figure 3. Representative photomicrographs of the GS in control group (A) and AICAR group (B). Cross-sections of GS muscle stained for myosin ATPase after pre-incubation in pH 4.60–4.65. Magnification of 200× was used (bar = 200 μm). I: fiber type I, black; IIA: fiber type IIA, white; IIB: fiber type IIB, brownness. n = 13 muscles per group.
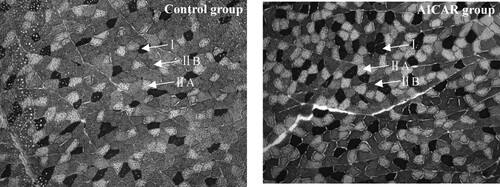
Table 2. Effect of AICAR treatment on muscle fiber characteristics of Wistar rats
3.4. MyHC expression at the mRNA levels
The results of MyHC mRNA levels are shown in . MyHC I mRNA level was increased significantly by AICAR treatment (P < .05), but MyHC IIb and MyHC IIx mRNA level were decreased significantly (P < .05). MyHC IIa was no significant in both groups (P > .05). These data were highly correlated with the results of staining methods of myosin mATPase. The data above showed that AICAR treatment can increase MyHC I mRNA level and reduce MyHC IIb and MyHC IIx mRNA level, which indicated that AICAR treatment can induce the transition of muscle fiber types from glycolysic to oxidized.
3.5. Metabolic enzyme activities
As shown in , the results showed that chronic AICAR treatment increased the SDH and MDH activities (P < .05), while the LDH activity was no significant differences between the AICAR treatment group and control group. That suggests that AICAR treatment can induce the oxidative capacity of muscle.
3.6. Comparison of key factors regulating muscle fibre type
Four weeks after AICAR treatment, we analysed the mRNA levels of PGC-1α, MEF2C and GLUT4 in GS muscle. As shown in , AICAR treatment in rats significantly up-regulated the mRNA levels of PGC-1α (P < .05) and GLUT4 mRNA level tended to be higher (P = .052) than that in the control group, while the mRNA levels of MEF2C were no significant between the AICAR treatment group and control group (P > .05).
4. Discussion
The aim of the current study was to reveal the effect of chronic AICAR treatment on muscle fiber composition in rats. To assess muscle fiber type composition, we detected muscle fiber characteristics and mRNA expression level of MyHC isoforms; meanwhile, the major metabolic enzyme activity and mRNA expression level of regulatory genes were evaluated. Current studies have indicated that chronic AICAR treatment could have an effect on the muscle fiber type composition in rats. AICAR treatment increased number and area proportion of type I muscle fiber, decreased number proportion of type IIB muscle fiber. Moreover, increased the MyHC I mRNA level and decreased the MyHC IIb and MyHC IIx mRNA level. Supporting that AICAR treatment induced the conversion of muscle fiber types from glycolytic to oxidized. This transformation is corresponded with an increase in PGC-1α mRNA level and enzyme activity change. AICAR treatment had higher activity of SDH and MDH, key enzymes involved in aerobic metabolism, while LDH activity which major enzyme relevant to anaerobic glycolysis was no significantly changed. GLUT4 mRNA level tended to be higher; however, MEF2C mRNA level was no significantly altered by AICAR treatment.
Accumulating evidence suggests that AMPK had been considered to play a vital role in the transformation of skeletal muscle fiber types. A sharp and continuous decline in the ratio of free ATP/ADP had been deemed as an important signal for the threshold of glycolytic to oxidized fiber conversion (Conjard et al. Citation1998). Previous studies had suggested that chronic AICAR treatment could induce fiber type transformation from fast to slow (Green et al. Citation1992; Suwa et al. Citation2003). Chronic AICAR treatment resulted in the proportion of type IIB muscle fibers significantly reduced and the proportion of type IIX muscle fibers significantly increased in the extensor digitorum longus (EDL) muscle of rats (Suwa et al. Citation2003). AICAR treatment can increased significantly the number of IIa muscle fibers in the EDL (Vladimir et al. Citation2011). A completely opposite result was observed in the semitendinosus muscle of rapidly growing pigs, which chronic AMPK treatment leaded to a decrease in MyHC IIa, and an increase in MyHC IIx, the result may be in connection with the time of regulation and overall growth of the animal (Park et al. Citation2009b). AMPK was also activated when skeletal muscle contract (Ko et al. Citation2018). Regular endurance exercise could lead to the activation of AMPK, furthermore promoting the transformation of muscle fiber types from fast-twitch to slow-twitch; however, the training-induced fiber transfer was inhibited in transgenic mice expressing a muscle-specific AMPKα2 inactivate subunit (Rockl et al. Citation2007). AMPK was activated by arginine could induce muscle fiber type transformation from fast-twitch to slow-twitch (Chen et al. Citation2018). These results revealed that AMPK was conducive to muscle fiber conversion. The current studies clearly demonstrate that AMPK was activated by AICAR can induce muscle fiber types conversions from glycolytic to oxidized in GS of Wistar rats.
Several studies had shown that PGC-1α is responsible for mitochondrial biological origin, oxidative metabolism and fast-to-slow transformation (Puigserver et al. Citation1998; Puigserver et al. Citation1999; Wu et al. Citation1999; Lin et al. Citation2002; Handschin et al. Citation2007a; Handschin et al. Citation2007b). An important finding was that the overexpression of the PGC1α gene promotes the transformation of muscle fibers from glycolytic to oxidizing muscle fibers of pigs (Ying et al. Citation2016). This finding was consistent with that the expression of PGC-1α gene could transform muscle fiber from fast to slow (Tetsuo et al. Citation2010). I and IIa muscle fibers were significantly reduced, while type IIb muscle fibers were significantly elevated in PGC-1α knockout mice (Handschin et al. Citation2007b). These studies revealed that PGC-1α play a pivotal role in muscle fibers transformation. This finding is consistent with that PGC-1α protein level and oxidative enzyme activities was significantly increased by AICAR treatment moreover, AICAR treatment the reduced proportion of type IIB muscle fibers and improved the proportion of type IIX muscle fibers (Suwa et al. Citation2003).
As cellular energy sensor (Hardie and Sakamoto Citation2006), AMPK activation increases mitochondrial oxidase activity and mitochondrial synthesis (Mu et al. Citation2001), mediated by PGC-1α, as the results of studies transgenic mice expressing a dominant-negative mutant of AMPK in muscle and knockout mice confirmed the important role of PGC-1α in the AMPK signalling pathway (Haihong et al. Citation2002). AMPK had an effect on the genes expression of GLUT4, related mitochondria, and PGC-1α were almost completely depended on the protein function of PGC-1α (Handschin et al. Citation2007b). This finding was consistent with that PGC-1α played an important role in the regulation of AMPK-induced mitochondria and glucose transporter expression in skeletal muscle (Leick et al. Citation2010). The current study had shown that the levels of PGC-1α mRNA were up-regulated by AICAR treatment, which was consistent with the results in the literature. Furthermore, we found that the activity of MDH and SDH which were applied to markers of the capacities oxidative in muscle. These suggest that AICAR treatment increased muscle oxidative capacity mediated by PGC-1α.
Activation of PGC-1α can induce with various specific transcription factors such as PPAR-α, PPARγ, etc., and promote the expression of key genes which induces muscle fiber type from oxidized to glycolytic switching (Puigserver and Spiegelman Citation2003; Handschin and Spiegelman Citation2006). Myocyte enhancer factor-2 (MEF2) is a major coactivator of PGC-1α; it can directly bind to the promoter of PGC-1α gene in muscle, PGC-1α expression and increase oxidative metabolism (Cantó and Auwerx Citation2010). PGC-1α can regulate the oxidative capacity and muscle fiber type transformation of skeletal muscle by regulating the expression of MEF2C (Lin et al. Citation2002). The current study showed that MEF2C mRNA level was not significantly altered by chronic AICAR treatment. This result implies that ME2C is involved in other signalling pathways regulating muscle fiber type switching, such as the calcium signalling, rather than the AMPK signalling pathway. CaN directly dephosphorylates MEF2, thereby enhancing the transcriptional activity of the type I muscle fiber gene (Tanner et al. Citation2002; Czubryt et al. Citation2003).
GLUT4 is a major functional protein involved in glucose transport under the stimulation of physiological factors such as contraction, hypoxia and insulin (James et al. Citation1989). A number of studies had shown that AMPK was a major factor in promoting GLUT4 metastasis. GLUT4 expression was very low in normal conditions, while it was transported to the cell membrane via translocation by the stimulation of insulin or AMPK signal (Hatakeyama and Kanzaki Citation2013), which made the expression of GLUT4 was significantly increased. Chronic AICAR treatment could increase GLUT4 content in skeletal muscle (Holmes et al. Citation1999; Ojuka et al. Citation2000; Koistinen et al. Citation2003; Park et al. Citation2009a). AMPK regulates the absorption of glucose through two pathways: enhancing the translocation of the glucose transporter and promoting the increase of glucose transporter expression, so that the absorption and transport of glucose by skeletal muscle is increased at rest (Avelar et al. Citation2011). AMPK is mainly localized in the cytoplasm (Silva-Grigoletto et al. Citation2011), upon activation, AMPK plays an important role in regulating numerous proteins in nucleus and regulates GLUT4 gene expression (Rønnestad and Ellefsen Citation2011). AICAR treatment could promote AMPK phosphorylation and increasing PGC-1α and GLUT4 protein at 24 h after administration of AICAR (Suwa et al. Citation2011). In this study, AICAR treatment for 4 weeks in GS of rats enhanced AMPK activity, increasing PGC-1α mRNA level and GLUT4 mRNA level. In addition, SDH and MDH activity which key enzymes involved in aerobic metabolism were improved.
GLUT4 had the specificity of muscle fiber type in skeletal muscle cells; moreover, the protein content of GLUT4 was higher in red muscle fibers than in white muscle fibers, which indicated that the content of GLUT4 in oxidized muscle fibers was higher than that in glycolytic muscle fibers (Marette et al. Citation1992). This also accords with our earlier observations, which showed that MyHC I mRNA level was higher in the AICAR treatment group; however, an inverse result was observed in MyHC IIb and MyHC IIx mRNA level. These results suggest that oxidized muscle fibers (slow muscle fibers) were higher and glycolytic muscle fibers (fast muscle fibers) were lower in AICAR treatment group. This was consistent with increased GLUT4 mRNA level.
In summary, this study had shown that AICAR treatment for 4 weeks increased number and area proportion of type I muscle fiber and the MyHC I mRNA level, decreased number proportion of type IIB muscle fiber and the MyHC IIb and MyHC IIx mRNA level in GS of rats. In addition, AICAR treatment also enhanced oxidative enzyme activities and GLUT4 mRNA level in GS of rats. That at least several such adaptations with AICAR treatment may be due to an increased PGC-1α mRNA level. On the other hand, the mRNA level of MEF2C was no significantly altered by AICAR treatment, which suggest that MEF2C mRNA level may be independent of the AMPK signalling pathway.
Ethics statement
All experimental procedures were strictly conducted in accordance with the Inner Mongolia Agricultural University guidelines for the Care and Use of Laboratory Animals and were approved by the University Animal Experiment Committee.
Disclosure statement
No potential conflict of interest was reported by the author(s).
Additional information
Funding
References
- Avelar NC, Simão AP, Tossige-Gomes R, Neves CD, Mezencio B, Szmuchrowski L, Coimbra CC, Lacerda AC. 2011. Oxygen consumption and heart rate during repeated squatting exercises with or without whole-body vibration in the elderly. J Strength Condition Res. 25(12):3495–3500. doi:10.1519/JSC.0b013e3182176664.
- Brooke MH, Kaise KK. 1970. Three “myosin adenosine triphosphatase” systems: the nature of their pH lability and sulfhydryl dependence. J Histochem Cytochem. 18(9):670–672.
- Cantó C, Auwerx J. 2010. AMP-activated protein kinase and its downstream transcriptional pathways. Cell Mol Life Sci. 67(20):3407–3423. doi:10.1007/s00018-010-0454-z.
- Chen X, Gang Jia YG, Liu G, Zhao H, Huang Z. 2018. Arginine promotes skeletal muscle fiber type transformation from fast-twitch to slow-twitch via Sirt1/AMPK pathway. J Nutr Biochem. 61:155–162.
- Choi YM, Hwang S, Lee K. 2016. Comparison of muscle fiber and meat quality characteristics in different Japanese quail lines. Asian-Australas J Anim Sci. 29:1331–1337. doi:10.5713/ajas.16.0329.
- Conjard A, Peuker H, Pette D. 1998. Energy state and myosin heavy chain isoforms in single fibres of normal and transforming rabbit muscles. Pflügers Archiv Eur J Physiol. 436(6):962–969. doi:10.1007/s004240050730.
- Czubryt MP, McAnally J, Fishman GI, Olson EN. 2003. Regulation of peroxisome proliferator-activated receptor γ coactivator 1α (PGC-1α) and mitochondrial function by MEF2 and HDAC5. Proc Natl Acad Sci USA. 100(4):1711–1716.
- Green HJ, Düsterhöft S, Dux L, Pette D. 1992. Metabolite patterns related to exhaustion, recovery and transformation of chronically stimulated rabbit fast-twitch muscle. Pflügers Archiv Eur J Physiol. 420(3-4):359–366. doi:10.1007/BF00374471.
- Haihong Z, Ming RJ, Young LH, Marc P, James M, Birnbaum MJ, Shulman GI. 2002. AMP kinase is required for mitochondrial biogenesis in skeletal muscle in response to chronic energy deprivation. Proc Natl Acad Sci USA. 99(25):15983–15987.
- Han Y, Wang Q, Song P, Zhu Y, Zou MH, Xu A. 2010. Redox regulation of the AMP-activated protein kinase. Plos One. 5(11):e15420.
- Handschin C, Chin S, Li P, Liu F, Maratos-Flier E, LeBrasseur NK, Yan Z, Spiegelman BM. 2007b. Skeletal muscle fiber-type switching, exercise intolerance, and myopathy in PGC-1α muscle-specific knock-out animals. J Biol Chem. 282(41):30014–30021.
- Handschin C, Choi CS, Chin S, Kim S, Kawamori D, Kurpad AJ, Neubauer N, Hu J, Mootha VK, Kim Y-B, et al. 2007a. Abnormal glucose homeostasis in skeletal muscle-specific PGC-1alpha knockout mice reveals skeletal muscle-pancreatic beta cell crosstalk. J Clin Invest. 117(11):3463–3474.
- Handschin C, Spiegelman BM. 2006. Peroxisome proliferator-activated receptor γ coactivator 1 coactivators, energy homeostasis, and metabolism. Endocr Rev. 27(7):728–735. doi:10.1210/er.2006-0037.
- Hardie DG, Carling D, Carlson M. 1998. The AMP-activated/SNF1 protein kinase subfamily: metabolic sensors of the eukaryotic cell? Annu Rev Biochem. 67(1):821–855.
- Hardie DG, Sakamoto K. 2006. AMPK: a key sensor of fuel and energy status in skeletal muscle. Physiology. 21(1):48–60. doi:10.1152/physiol.00044.2005.
- Hatakeyama H, Kanzaki M. 2013. Regulatory mode shift of Tbc1d1 is required for acquisition of insulin-responsive GLUT4-trafficking activity. Mol Biol Cell. 24(6):809–817. doi:10.1091/mbc.e12-10-0725.
- He X, Duan Y, Yao K, Li F, Hou Y, Wu G, Yin Y. 2015. β-Hydroxy-β-methylbutyrate, mitochondrial biogenesis, and skeletal muscle health. Amino Acids. 48(3):653–664.
- Herbison GJ, Jaweed MM, Ditunno JF. 1982. Muscle fiber types. Arch Phys Med Rehabil. 63(5):227–230.
- Hoh JF. 1992. Muscle fiber types and function. Curr Opin Rheumatol. 4(6):801–808.
- Holmes BF, Kurthkraczek EJ, Winder WW. 1999. Chronic activation of 5'-AMP-activated protein kinase increases GLUT-4, hexokinase, and glycogen in muscle. J Appl Physiol. 87(5):1990–1995. doi:10.1016/j.laa.2005.07.006.
- James DE, Strube M, Muecdler M. 1989. Molecular cloning and characterization of an insulin-regulatable glucose transporter. Nature. 338(6210):83–87. doi:10.1038/338083a0.
- Kang YK, Choi YM, Lee SH, Choe JH, Hong KC, Kim BC. 2011. Effects of myosin heavy chain isoforms on meat quality, fatty acid composition, and sensory evaluation in Berkshire pigs. Meat Sci. 89(4):384–389. doi:10.1016/j.meatsci.2011.04.019.
- Ko JR, Seo DY, Park SH, Kwak HB, Kim M, Ko KS, Rhee BD, Han J. 2018. Aerobic exercise training decreases cereblon and increases AMPK signaling in the skeletal muscle of STZ-induced diabetic rats. Biochem Biophys Res Commun. 501(2):448–453. doi:10.1016/j.bbrc.2018.05.009.
- Koistinen HA, Galuska D, Chibalin AV, Yang J, Zierath JR, Holman GD, Wallberg-Henriksson H. 2003. 5-amino-imidazole carboxamide riboside increases glucose transport and cell-surface GLUT4 content in skeletal muscle from subjects with type 2 diabetes. Diabetes. 52(5):1066–1072. doi:10.2337/diabetes.52.5.1066.
- Leick L, Fentz J, Biensø RS, Knudsen JG, Jeppesen J, Kiens B, Wojtaszewski JFP, Pilegaard H. 2010. PGC-1α is required for AICAR-induced expression of GLUT4 and mitochondrial proteins in mouse skeletal muscle. Am J Physiol Endocrinol Metab. 299(3):E456.
- Lin CC, Liang JH. 2002. Effect of antioxidants on the oxidative stability of chicken breast meat in a dispersion system. J Food Sci. 67(2):530–533.
- Lin J, Wu H, Tarr PT, Zhang C-Y, Wu Z, Boss O, Michael LF, Puigserver P, Isotani E, Olson EN, et al. 2002. Transcriptional co-activator PGC-1 alpha drives the formation of slow-twitch muscle fibres. Nature. 418(6899):797.
- Marette A, Richardson JM, Ramlal T, Balon TW, Vranic M, Pessin JE, Klip a. 1992. Abundance, localization, and insulin-induced translocation of glucose transporters in red and white muscle. Am J Physiol. 263(2 Pt 1):C443–C452. doi:10.0000/PMID1514590.
- Michael LF, Wu Z, Cheatham RB, Puigserver P, Adelmant G, Lehman JJ, Kelly DP, Spiegelman BM. 2001. Restoration of insulin-sensitive glucose transporter (GLUT4) gene expression in muscle cells by the transcriptional coactivator PGC-1. Proc Natl Acad Sci USA. 98(7):3820–3825. doi:10.1073/pnas.061035098.
- Mu J, Brozinick JT, Valladares O, Bucan M, Birnbaum MJ. 2001. A role for AMP-activated protein kinase in contraction- and hypoxia-regulated glucose transport in skeletal muscle. Mol Cell. 7(5):1085–1094. doi:10.1016/S1097-2765(01)00251-9.
- Nakazato K, Tsutaki A. 2012. Regulatory mechanisms of muscle fiber types and their possible interactions with external nutritional stimuli. J Phys Fitness Sports Med. 1(4):655–664. doi:10.7600/jpfsm.1.655.
- Ojuka EO, Nolte LA, Holloszy JO. 2000. Increased expression of GLUT-4 and hexokinase in rat epitrochlearis muscles exposed to AICAR in vitro. J Appl Physiol. 88(3):1072–1075. doi:10.1152/jappl.2000.88.3.1072.
- Park S, Scheffler TL, Gunawan AM, Shi H, Zeng C, Hannon KM, Grant AL, Gerrard DE. 2009a. Chronic elevated calcium blocks AMPK-induced GLUT-4 expression in skeletal muscle. Am J Physiol Cell Physiol. 296(1):106–115. doi:10.1152/ajpcell.00114.2008.
- Park SK, Sheffler TL, Spurlock ME, Grant AL, Gerrard DE. 2009b. Chronic activation of 5"-AMP-activated protein kinase changes myosin heavy chain expression in growing pigs. J Anim Sci. 87(10):3124–3133. doi:10.2527/jas.2009-1989.
- Pette D, Staron RS. 1997. Mammalian skeletal muscle fiber type transitions. Int Rev Cytol. 170:143–223. doi:10.1016/S0074-7696(08)61622-8.
- Pette D, Staron RS. 2000. Myosin isoforms, muscle fiber types, and transitions. Microsc Res Tech. 50(6):500–509. doi:10.1002/1097-0029(20000915)50:6 < 500::AID-JEMT7 > 3.3.CO;2-Z.
- Puigserver P, Adelmant G, Wu Z, Fan M, Xu J, O’Malley B, Spiegelman BM. 1999. Activation of PPARgamma coactivator-1 through transcription factor docking. Science. 286:1368–1371.
- Puigserver P, Spiegelman BM. 2003. Peroxisome proliferator-activated receptor-γ coactivator 1α (PGC-1α): transcriptional coactivator and metabolic regulator. Endocr Rev. 24(1):78–90.
- Puigserver P, Wu Z, Park CW, Graves R, Spiegelman BM. 1998. A cold-inducible coactivator of nuclear receptors linked to adaptive thermogenesis. Cell. 92(6):829–839. doi:10.1016/S0092-8674(00)81410-5.
- Rockl KSC, Hirshman MF, Brandauer J, Fujii N, Witters LA, Goodyear LJ. 2007. Skeletal muscle adaptation to exercise training: AMP-activated protein kinase mediates muscle fiber type shift. Diabetes. 56(8):2062–2069. doi:10.2337/db07-0255.
- Rønnestad BR, Ellefsen S. 2011. The effects of adding different whole-body vibration frequencies to preconditioning exercise on subsequent sprint performance. J Strength Cond Res. 25(12):3306–3310. doi:10.1519/JSC.0b013e318215f298.
- Scarpulla RC. 2002. Nuclear activators and coactivators in mammalian mitochondrial biogenesis. Biochim Biophys Acta Gene Struct Expr. 1576(1):1–14. doi:10.1016/S0167-4781(02)00343-3.
- Schiaffino S, Reggiani C. 2011. Fiber types in mammalian skeletal muscles. Physiol Rev. 91(4):1447–1531.
- Silva-Grigoletto MED, Hoyo MD, Sañudo B, Carrasco L, García-Manso JM. 2011. Determining the optimal whole-body vibration dose–response relationship for muscle performance. J Strength Condition Res. 25(12):3326–3333. doi:10.1519/JSC.0b013e3182163047.
- Su R, Luo Y, Wang B, Hou Y, Zhao L, Su L, Yao D, Qian Y, Jin Y. 2019. Effects of physical exercise on meat quality characteristics of Sunit sheep. Small Ruminant Res. 173:54–58. doi:10.1016/j.smallrumres.2019.02.002.
- Suwa M, Nakano H, Kumagai S. 2003. Effects of chronic AICAR treatment on fiber composition, enzyme activity, UCP3, and PGC-1 in rat muscles. J Appl Physiol. 95(3):960–968. doi:10.1152/japplphysiol.00349.2003.
- Suwa M, Nakano H, Radak Z, Kumagai S. 2011. Short-term adenosine monophosphate-activated protein kinase activator 5-aminoimidazole-4-carboxamide-1-β-d-ribofuranoside treatment increases the sirtuin 1 protein expression in skeletal muscle. Metab Clin Exp. 60(3):394–403. doi:10.1016/j.metabol.2010.03.003.
- Tanner CJ, Barakat HA, Dohm GL, Pories WJ, MacDonald KG, Cunningham PRG, Swanson MS, Houmard JA. 2002. Muscle fiber type is associated with obesity and weight loss. Am J Physiol Endocrinol Metab. 282(6):E1191–E1196. doi:10.1152/ajpendo.00416.2001.
- Tetsuo Y, Takayoshi S, Hideaki A, Shihori T, Yoriko A. 2010. Continuous mild heat stress induces differentiation of mammalian myoblasts, shifting fiber type from fast to slow. Am J Physiol Cell Physiol. 298(1):C140–C148.
- Thomson DM, Fick CA, Gordon SE. 2008. AMPK activation attenuates S6K1, 4E-BP1, and eEF2 signaling responses to high-frequency electrically stimulated skeletal muscle contractions. J Appl Physiol. 104(3):625–632.
- Vladimir L, Pedro M, Matthew B, Louise B, Shiemaa K, Lunde JA, Renaud J-M, Jasmin BJ. 2011. Chronic AMPK activation evokes the slow, oxidative myogenic program and triggers beneficial adaptations in mdx mouse skeletal muscle. Hum Mol Genet. 20(17):3478–3493.
- Wu Z, Puigserver P, Andersson U, Zhang C, Adelmant G, Mootha V, Troy A, Cinti S, Lowell B, Scarpulla RC, Spiegelman BM. 1999. Mechanisms controlling mitochondrial biogenesis and respiration through the thermogenic coactivator PGC-1. Cell. 98(1):115–124.
- Yan Z, Okutsu M, Akhtar YN, Lira VA. 2011. Regulation of exercise-induced fiber type transformation, mitochondrial biogenesis, and angiogenesis in skeletal muscle. J Appl Physiol. 110(1):264–274. doi:10.1152/japplphysiol.00993.2010.
- Ying F, Zhang L, Bu G, Xiong Y, Zuo B. 2016. Muscle fiber-type conversion in the transgenic pigs with overexpression of PGC1α gene in muscle. Biochem Biophys Res Commun. 480(4):669–674. doi:10.1016/j.bbrc.2016.10.113.