ABSTRACT
In recent years, the large Low Earth Orbit (LEO) constellations have become a hot topic due to their great potential to improve the Global Navigation Satellite Systems (GNSS) positioning performance. One of the important focus is how to obtain the accurate and reliable orbits for these constellations with dozens of LEO satellites. The GNSS-based Precise Orbit Determination (POD) will be exclusively performed to achieve this goal, where the Integer Ambiguity Resolution (IAR) plays a key role in acquiring high-quality orbits. In this study, we present a comprehensive analysis of the benefit of the single-receiver IAR in LEO POD and discuss its implication for the future LEO constellations. We perform ambiguity-fixed LEO POD for four typical missions, including Gravity Recovery and Climate Experiment (GRACE) Follow-On (GRACE-FO), Swarm, Jason-3 and Sentinel-3, using the Uncalibrated Phase Delay (UPD) products generated by our GREAT (GNSS+ REsearch, Application and Teaching) software. The results show that the ambiguity fixing processing can significantly improve the accuracy of LEO orbits. There are negligible differences between our UPD-based ambiguity-fixed orbits and those based on the Observable Signal Bias (OSB) and Integer Recovery Clock (IRC) products, indicating the good-quality of UPD products we generated. Compared to the float solution, the fixed solution presents a better consistency with the external precise science orbits and the largest accuracy improvement of 5 mm is achieved for GRACE-FO satellites. Meanwhile, the benefit can be observed in laser ranging residuals as well, with a Standard Deviation (STD) reduction of 3–4 mm on average for the fixed solutions. Apart from the absolute orbits, the relative accuracy of the space baseline is also improved by 20–30% in the fixed solutions. The result demonstrates the superior performance of the ambiguity-fixed LEO POD, which appears as a particularly promising technique for POD of future LEO constellations.
1. Introduction
Over the past two decades, Low Earth Orbit (LEO) satellites have been widely used in Earth system monitoring and studying such as meteorology detection, gravity field inversion, and magnetic field exploration. For instance, Gravity Recovery and Climate Experiment (GRACE) was aimed at determining the gravity field and its temporal variations of Earth with unprecedented accuracy (Tapley et al. Citation2004). Another typical example is European Space Agency (ESA) Swarm mission, whose objective is to provide the best ever survey of the geomagnetic field and its temporal evolution, and obtain new insights into improving our knowledge of the Earth’s interior and climate (Friis-Christensen et al. Citation2008). In recent years, the large constellations with dozens of, even hundreds of LEO satellites, have become a hotspot due to its exciting prospect in global communication. Among them, several LEO constellations in construction integrate the function of communication and navigation, such as the LEO constellation called Hong Yan implemented by China Aerospace Science and Technology Corporation. Compared with Global Navigation Satellite System (GNSS) satellites, the LEO spacecraft has a lower orbit altitude and faster motion, which leads to a stronger power of received signals and offers rapid variations in satellites’ geometric distribution (Li et al. Citation2019a, Citation2019b; Ge et al. Citation2021). These LEO constellations have great potential to improve the positioning, navigation and timing service of GNSS by delivering the navigation signals (Reid et al. Citation2018).
Obtaining accurate and reliable orbit information is a key issue for both Earth observation LEO satellites and navigation-augmented LEO constellation. Generally, there are three methods to generate LEO orbits, namely, the kinematic method, the dynamic method, and the reduced dynamic method. The kinematic method makes no use of the dynamic information of satellites and exclusively relies on the observations (Bisnath and Langley Citation1999), which can be affected easily by the quality of tracking observations. In contrast, the dynamic method has a strong dependence on the high-accuracy force models. These force models are not always achievable, especially for the non-gravitational force. In order to solve the limitations of these two methods, the reduced-dynamic orbit determination method is proposed by Yunck et al. (Citation1990) and Wu, Yunck, and Thornton (Citation1988), which compensates potential deficiency in the employed dynamic models by introducing pseudo-stochastic parameterization.
The onboard GNSS technology represents the currently primary method to acquire the precise orbit of LEO satellites with the accuracy of a few centimeters (Peng and Wu Citation2009; Bock et al. Citation2011; Montenbruck et al. Citation2018b). Similar to Precise Point Positioning (PPP), the LEO Precise Orbit Determination (POD) also benefits from the Integer Ambiguity Resolution (IAR) processing (Jäggi et al. Citation2007; Allende-Alba and Montenbruck Citation2016; Li et al. Citation2018). In the early time, the ambiguities were usually resolved using the Double-Difference (DD) IAR method, which requires to form an inter-satellite or satellite-ground baseline. However, the implementation of this method usually need an additional LEO satellite flying in a stable formation with a short distance or a globally distributed ground network, which greatly restricts its application in orbit determination of LEO satellites. In recent years, the single-receiver IAR method using external bias products has been widely applied in POD of LEO satellites. Laurichesse et al. (Citation2009) realize the undifferenced IAR POD for Jason-1 using the Integer Recovery Clock (IRC) products, proving that the IAR can improve the orbit accuracy with the Satellite Laser Ranging (SLR) residuals reduced by 1.5 mm on average. Using the same products, Montenbruck, Hackel, and Jaggi (Citation2018a) apply the single-receiver IAR to the POD of Sentinel-3 and report an obvious decrease of 4 mm in the Standard Deviation (STD) of SLR residuals for high-grade stations. Guo et al. (Citation2019) propose an integrated IAR scheme of combining the single-receiver IAR and DD IAR for LEO POD and demonstrate that the method can achieve the highest absolute and relative orbit accuracies simultaneously. Li et al. (Citation2019c) perform the ambiguity-fixed kinematic POD for LEO satellites based on the estimated real-time Uncalibrated Phase Delay (UPD) products and the improvements of SLR residuals are over 20% compared with ambiguity-float solutions. With the support of the Observable Signal Bias (OSB) products, Arnold et al. (Citation2019) realize the IAR POD for several LEO satellites, which have better performance than ambiguity-float solutions. There are many other publications demonstrating the superior performance of the single-receiver IAR in LEO POD (Bertiger et al. Citation2010; Loyer et al. Citation2012).
The study presents a detailed performance assessment and analysis of LEO POD with single-receiver IAR and attempts to provide a reference for the future LEO constellation POD. Four LEO missions, including GRACE Follow-On (GRACE-FO), Swarm, Jason-3 and Sentinel-3, which consist of satellites moving in different orbital heights and inclinations, are selected (Vaze et al. Citation2010; Seitz et al. Citation2010; Ijssel et al. Citation2015; Landerer et al. Citation2020). The lessons learned from these Earth observation missions are helpful to the POD scheme design and processing for future LEO constellations. We employed the UPD products generated by GREAT (GNSS+ REsearch, Application and Teaching) software to achieve the ambiguity-fixed LEO POD. Meanwhile, OSB products from Center for Orbit Determination in Europe (CODE) and the IRC products from Centre National D’Etudes Spatiales (CNES) are also used to investigate the IAR with different products.
This article is organized as follows: The descriptions of GREAT software and GREAT products are given in Section 2. Then, the orbit determination settings and strategies are presented in Section 3. Thereafter, the LEO POD results of different solutions are evaluated and analyzed in Section 4. Finally, the summary and discussion are provided in Section 5.
2. GREAT software
In order to meet the demands of scientific and engineering applications in geodesy and navigation fields, GREAT software was designed and developed at the School of Geodesy and Geomatics of Wuhan University (WHU). Written in standard C++ 11 language following the Object-Oriented Principle, GREAT software can provide multiple applications such as multi-technique geodetic parameter estimation, GNSS real-time orbit and clock determination, LEO augmentation, PPP-RTK (Real-time kinematic), multi-GNSS bias estimation, multi-sensor integration navigation, etc. Each function module of GREAT software owns complete and explicit interfaces, which are convenient for developers to expand functions based on existing modules. The software can run on most mainstream operating systems such as Windows, Linux, and MacOS using the configuration file in standard Extensible Markup Language format. Currently, we have opened the source code of UPD estimation module called GREAT-UPD and the community can freely obtain the corresponding package at https://geodesy.noaa.gov/gps-toolbox (Li et al. Citation2021).
The GREAT software has been applied in International GNSS Monitoring and Assessment System (iGMAS) Innovation Center in WHU to generate precise orbit and multi-frequency clock products, UPD corrections of multi-GNSS satellites, and other featured products. These products are available at http://igmas.users.sgg.whu.edu.cn/, from which users can expediently batch download post-processed or real-time products.
In this study, we adopt the GNSS precise products generated by GREAT software. The LEO-POD module of GREAT software that integrates the kinematic POD and reduced-dynamic POD simultaneously has been applied in our study. The whole data processing for LEO POD is shown in . In the first step, we process the onboard observations using kinematic method to provide an initial orbit for the subsequent step. Then, based on the orbit elements derived from the kinematic orbit and dynamic models, the reduced-dynamic orbit of LEO satellite is estimated with ambiguity parameters as float values. In the third step, the IAR process is implemented using the float ambiguities from the previous POD solutions and the UPD products generated by GREAT software. The fixed ambiguities are subsequently used to offer a tight constraint to the ambiguity parameters. Usually, 2–3 iterations with ambiguity fixing are needed to get a final solution.
3. Data sets and POD strategies
3.1 Data sets
In this study, we focus on eight LEO satellites from four typical geodetic and oceanographic missions, including GRACE-FO, Swarm-A/B/C, Jason-3 and Sentinel-3A/3B, which are flying at the low Earth orbit with different inclinations and different altitudes of 400–1300 km. These satellites are equipped with the onboard Global Positioning System (GPS) receivers which are from different manufacturers for the purpose of POD. presents an overview of four LEO missions and the onboard receiver they carried.
Table 1. Overview of satellites employed
The onboard GNSS observations from 001 to 180 Day of Year (DOY) in 2020 are selected to investigate the performance of ambiguity-fixed POD. It should be mentioned that onboard GPS data of Swarm and Sentinel-3 satellites suffers from a similar problem of half-cycle bias in ambiguities due to the adopted signal tracking method, which certainly hampers the IAR (Mao, Visser, and Van Citation2019). But this issue can be coped with using the method in Montenbruck, Hackel, and Jaggi (Citation2018a). In this study, we use the latest release of Swarm and Sentinel-3 data with the half-cycle ambiguity removed. Besides GPS observations, we also use the attitude data collected onboard to provide accurate attitude information of LEO platforms. The data onboard GRACE-FO, Swarm, Jason-3 and Sentinel-3 are publicly available from ftp://isdcftp.gfz-potsdam.de, ftp://swarm-diss.eo.esa.int, ftp://ftp-access.aviso.altimetry.fr and https://scihub.copernicus.eu respectively.
3.2 POD strategies
In this study, the LEO POD is performed using the reduced-dynamic method due to its superior POD performance and the widest application in LEO missions. The detailed information about the dynamic models as well as the observation models are given in .
Table 2. Reduced-dynamic POD strategy of LEO satellites
Generally, the satellites in orbit are affected by a variety of perturbation forces, which can be classified into gravitational and non-gravitational forces. The high-accuracy models are usually available for the gravitational forces, while the situation is different for the non-gravitational forces, such as atmosphere drag and Solar Radiation Pressure (SRP). The computation of these surface forces is closely related to the geometry and surface character of the LEO spacecraft. In this study, we use the macro-model to provide a rough information about the LEO satellite shape, which assumes the satellite consists of a rectangular box and solar panels. Based on this model, the atmosphere drag is computed using the NRLMSISE00 thermosphere model. To make up for the shortcomings in drag models, a set of cycle-per-revolution scale factors are estimated. While SRP is modeled using macro-model with thermo-optical property information of each panel. In addition, a set of empirical accelerations in the along-track and cross-track directions per cycle are taken into account to compensate for the deficiency of dynamic models we employed.
We use undifferenced Ionosphere-Free (IF) code and phase observations to remove the first-order of ionospheric delay. The arc length is set to 30 h while the sampling is 10 s. The GREAT LEO-POD module employs a batch least squares estimator to process undifferenced GPS observations. Taking account of the errors in the process of signal propagation, the corrections as exhibited in are carried out during the establishment of observation equations.
The single-receiver IAR is applied in this study to determine ambiguity-fixed orbits for LEO satellites. In this method, the IF phase ambiguities are decomposed into Wide-Lane (WL) and Narrow-Lane (NL) ambiguities. After employing GNSS phase bias products, e.g. UPD and OSB products, the satellite UPD can be corrected, and the receiver UPD are removed by forming single difference ambiguity between GNSS satellites. Then, the obtained single-difference WL and NL ambiguities are close to integers and can be fixed to integers by a rounding strategy (Dong and Bock Citation1989). We can achieve the ambiguity-fixed LEO POD through imposing constraints of integer WL and NL ambiguities. In this study, we employ the UPD products generated by GREAT software to achieve the ambiguity-fixed POD. Additionally, OSB products from CODE and the IRC products from CNES are also used for the external comparison, and GNSS orbit and clock products from CODE and CNES are used to match with the bias products respectively in orbit determination processing on GREAT software. In subsequent analysis, we will focus on the comparison and evaluation of the POD results based on these three different products.
4. Results and analysis
In this section, the POD results of aforementioned eight LEO satellites are analyzed and discussed. All the results are evaluated by (1) ambiguity fixing performance, (2) comparison with precise science orbit (PSO), (3) phase residuals, (4) SLR validation, and (5) K/Ka-band Ranging (KBR) validation.
4.1 Ambiguity fixing performance
The ambiguity fixing rate is a good indicator to measure the fixing performance of the IAR method. Here, we mainly focus on the performance of NL ambiguities, since the WL fixing rates reach to 100% for most solutions. illustrates the fixing rates of NL ambiguities of three IAR solutions for each satellite. For all LEO satellites, the average NL ambiguity fixing rates can reach over 93.8%, and most of them are nearly close to 100%, which indicate a good ambiguity fixing performance. For the same LEO satellite, the fixing rates of the solutions using different products are almost similar. Only a slightly higher ambiguity fixing rate is observed in the OSB solutions compared with the other two solutions. The results indicate that the LEO ambiguity-fixed POD using GREAT UPD products can achieve almost the same performance as the IRC solutions and the OSB solutions in terms of the ambiguity fixing rates.
Figure 2. NL ambiguity fixing rates of POD IAR solutions for GRACE-FO, Swarm, Jason-3 and Sentinel-3 missions, in DOY 001–180, 2020. The red, green and blue colors indicate the fixed solutions computed with OSB, IRC and UPD products respectively. The average values are displayed in the top right corners in different colors. The data gap of GRACE-C covering DOY 038–042, GRACE-D covering DOY 018–022 and Jason-3 covering DOY 031–044, 168–171 in 2020 is due to the lack of observations.
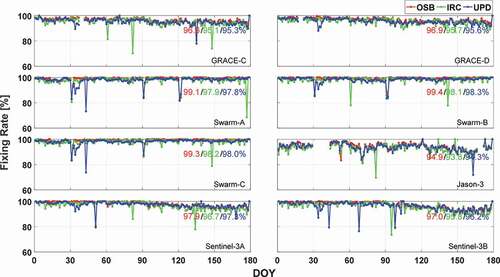
representatively presents the distributions of the WL and NL ambiguity residuals for GRACE-C satellite. Here, we compute the ambiguity residuals as the differences between the bias-corrected (both satellite and receiver sides) ambiguity and its nearest integer. It can be seen that the fractional parts of WL and NL ambiguities after removing biases are extremely close to zero, with more than 97.3% of WL ambiguity residuals within 0.25 cycles, and more than 94.9% of NL ambiguity residuals within 0.15 cycles. There are no evident differences in ambiguity residuals between the different IAR solutions, demonstrating the similar quality of these products. The ambiguity residuals of the other satellites exhibit a similar bell-shaped distribution as GRACE-C.
4.2 Comparison with PSOs
The comparison with the external orbit products provides an effective method to evaluate the accuracy of our POD results. In this study, we compare our results with PSO products generated by NASA Jet Propulsion Laboratory (JPL) for GRACE-FO, ESA for Swarm, CNES for Jason-3 and the Copernicus POD Service for Sentinel-3, respectively.
Statistics of the average 3D Root Mean Square (RMS) values of orbit differences with respect to the PSO products for these satellites POD solutions are summarized in . On the whole, the 3D orbit accuracy of ambiguity-float solutions is better than 2 cm for most LEO satellites, which demonstrates the good-quality of the models and products we used. A pronounced orbit accuracy improvement is visible for all satellites when the ambiguities are fixed. Compared with float solutions, the orbit differences of ambiguity-fixed solutions can be reduced by 1–5 mm in most cases. It indicates that the single-receiver IAR can significantly improve the orbit accuracy of LEO satellites. Among all ambiguity-fixed solutions, the OSB solutions show the best consistency with the PSO products, while the accuracy of our UPD solutions is slightly worse than that of OSB solutions. But we can find that the differences between these two solutions are marginal, which are confined to less than 0.5 mm for most satellites except for Jason-3. This implies that our UPD products can almost achieve a comparable performance to CODE OSB products. By contrast, it is visible that the IRC solutions exhibit a larger RMS value of 1–2 mm than that of OSB solutions, which may be attributed to the quality of GPS orbit and clock products.
Table 3. The average 3D RMS values of differences between PSO products and our orbits derived from different solutions (Unit: mm)
represents the average RMS values of orbit differences in three directions for eight satellites. Compared with ambiguity-float orbits, the accuracy of the ambiguity-fixed orbits of all satellites are generally improved in all directions. The improvements in along-track are the most conspicuous, which can reach 2.8 mm on average. By contrast, the accuracy improvement contributed by the ambiguity-fixing processing is limited in the radial direction. This is because that the radial direction of the satellite orbit is strongly constrained by the gravitational forces. In addition, we can find that the differences between the three orbit solutions for the same satellite become smaller after fixing the ambiguities.
Figure 4. Average RMS values of orbit differences of GRACE-FO, Swarm, Jason-3 and Sentinel-3 in along-track, cross-track, and radial directions (from top to bottom) in DOY 001–180, 2020. The red, green and blue colors indicate the OSB solutions, the IRC solutions and the UPD solutions respectively. The color bars without edges represent the ambiguity-float solutions, while the bars with black edges represent the ambiguity-fixed solutions.
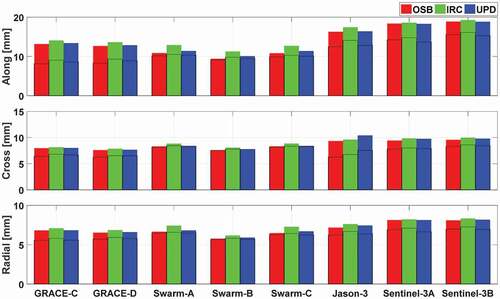
4.3 Phase residuals
In addition, we also calculate the RMS values of posteriori phase residuals for the float and fixed POD solutions, which are presented in . The results show that the average RMS values for ambiguity-float and ambiguity-fixed solutions using different products are all below 10 mm except for Jason-3. It indicates that the adopted models in the POD processing present a good consistency with the onboard observations. The comparison of ambiguity-float and ambiguity-fixed solutions indicates that the phase residuals for the ambiguity-fixed solutions are slightly larger than those for the ambiguity-float solutions, owing to the fact that part of the model errors absorbed by the float ambiguities can be reflected in residuals if the ambiguities are fixed.
Table 4. The average RMS values of phase residuals of our orbits for different solutions (Unit: mm)
4.4 SLR validation
Other than the comparison with PSO products, we also compare our POD solutions with SLR measurements collected by ILRS (Pearlman et al. Citation2019). We calculate the SLR residuals as the differences of measured distance and modeled distance between the ground stations and LEO satellites. Here we select a set of 12 high-performance stations to avoid the impact of SLR stations with poor tracking performance (Montenbruck, Hackel, and Jaggi Citation2018a). The outlier threshold of SLR residuals is set to ±20 cm and the minimum elevation is set to 10°.
From statistics of SLR residuals shown in , it can be found that the SLR residuals of the ambiguity-fixed solution exhibit evidently smaller STD values. The benefit of IAR is most prominent for the GRACE-FO mission, whose improvements can reach up to about 30%. However, it can be observed that there is a satellite-specific bias in the SLR residuals, which shows up in a similar way for both the ambiguity-float and ambiguity-fixed orbits. The bias is more evident for the GRACE-D and Swarm satellites. It may be associated with the LRA offset applied to correct the reference point from the optical reference point of LRA to the satellite center-of-mass, which cannot be eliminated by the ambiguity fixing processing.
Table 5. The mean and STD values of SLR residuals of our orbits for different solutions (Unit: mm)
illustrates the SLR residuals of GRACE-FO, Swarm, Jason-3 and Sentinel-3 orbits derived from three ambiguity-fixed solutions. The three different colored dots are highly concentrated within ±60 mm and hardly discernible. But more green discrete dots indicate that the orbits computed with IRC products may be not as stable as those computed with OSB or UPD products. Similar to the previous results, the LEO ambiguity-fixed orbits obtained from the UPD solutions have a similar level of SLR residuals to those from the OSB solutions. For some satellites, such as Jason-3 and Sentinel-3B, the UPD solutions even exhibit a slightly smaller STD value of SLR residuals.
Figure 5. SLR residuals of ambiguity-fixed orbits for GRACE-FO, Swarm, Jason-3 and Sentinel-3 satellites, in DOY 001–180, 2020. The red, green and blue dots indicate the ambiguity-fixed solutions computed with OSB, IRC, and UPD products respectively. The mean and STD values are displayed in the top right corners in different colors.
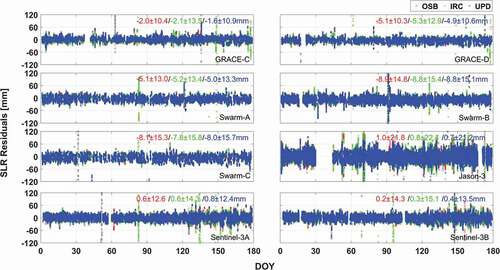
4.5 KBR validation
The twin satellites of GRACE-FO are equipped with KBR systems to make accurate measurements of the inter-satellite range at the micron level (Kang et al. Citation2020). Despite the KBR observations are mainly used for the recovery of Earth gravity, the differences between their measured and GPS‐derived intersatellite range can be regarded as a metric of the relative accuracy between the twin satellites.
shows the daily STD values of the KBR residuals of GRACE-FO satellite orbits, from which, we can find that most of daily STD values of KBR residuals for ambiguity-float solutions are less than 10 mm apart from a few outliers. As seen in the figure, the STD values of the ambiguity-fixed solutions are significantly lower than those of the ambiguity-float solutions by 2–3 mm, indicating that ambiguity-fixed solutions can perform better in precise baseline determination. Meanwhile, it is visible that the same relative accuracy has been achieved for the UPD and OSB solutions, which is consistent with their similar performance in previous results.
Figure 6. Daily KBR residuals STD of our GRACE-FO orbits for different solutions in DOY 001–180, 2020, with ambiguity fixed computed with OSB, IRC, and UPD products (from top to bottom) respectively. The blue and red colors indicate the ambiguity-float and ambiguity-fixed solutions respectively. The average values are displayed in the top right corners in different colors.
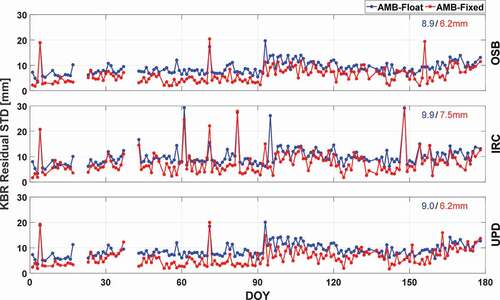
5. Summary and discussion
This study mainly focuses on the implementation of the single-receiver IAR in the precise orbit determination of LEO satellites. Onboard GNSS observations from eight different LEO satellites during DOY 001–180 in 2020 are processed. We adopt three bias products, the UPD products generated by GREAT software, OSB products from CODE and IRC products from CNES, to achieve ambiguity-fixed orbits for LEO satellites. All the data are processed on the GREAT software.
The result indicates that the single-receiver IAR can contribute to a notable improvement in both absolute accuracy of LEO orbits and the relative accuracy of space baseline. Taking GRACE-FO as an example, a reduction of 5 mm reflected in the 3D RMS of ambiguity-fixed orbit differences marks the accuracy improvement up to about 30% compared to ambiguity-float solutions. The higher consistency of the ambiguity-fixed solutions with the SLR measurements as well as an improved baseline determination precision in KBR validation also indicate the better quality of the ambiguity-fixed orbits.
Meanwhile, we find that there are roughly no pronounced differences observed between the UPD, OSB and IRC solutions. Our UPD products can achieve an equivalent performance to the CODE OSB products in the ambiguity fixing performance as well as the orbit accuracy, which is slightly better than IRC solutions. The result demonstrates the high-accuracy of products generated by GREAT software, which can be used to support the precise orbit recovery of future LEO constellations.
For the future large LEO constellations, the precise orbit determination for a large number of spacecraft is actually a great challenge. Generally, there are two typical optional strategies to achieve this goal. The first strategy is to adopt the same processing like GNSS constellations, which simultaneously determines all the satellite orbits in one common estimation. However, it should be noted that the modeling of non-gravitational forces acting on the LEO satellites is a more complicated issue than that of GNSS satellites, which usually demand additionally estimation of a lot of dynamic parameters. This will certainly increase the computational burden. On the other hand, the recovery of LEO orbit usually requires the onboard observations in a high sampling rate (such as 10 s) due to its high-speed move, while the ground observations with sampling interval of 300 s are sufficient to the GNSS POD. The dense onboard observations to be processed constitute another negative factor hindering the computational efficiency.
The second strategy is to estimate the orbit parameters of each LEO satellite separately, just like the procedure in this study. The orbits of the whole constellation can be obtained by the single-LEO POD in a parallel processing. This method is independent to the satellite number of LEO constellation and easy to implement. Considering both the accuracy and computational efficiency, we suggest to process LEO observations separately using ambiguity-fixed POD to obtain high-quality orbits of LEO constellation. The experience gained from this study will facilitate the successful implementation of POD for LEO constellations.
Acknowledgments
The numerical calculations in this study have been done on the supercomputing system in the Supercomputing Center of Wuhan University.
Disclosure statement
No potential conflict of interest was reported by the author(s).
Data availability statement
We are grateful to International GNSS Service (IGS) for providing the precise orbit and clock products of GPS as well as OSB and IRC correction products at ftp://cddis.gsfc.nasa.gov for free. The UPD products generated by GREAT software can be accessed at http://igmas.users.sgg.whu.edu.cn/. The data onboard GRACE-FO, Swarm, Jason-3 and Sentinel-3 are publicly available from ftp://isdcftp.gfz-potsdam.de, ftp://swarm-diss.eo.esa.int, ftp://ftp-access.aviso.altimetry.fr and https://scihub.copernicus.eu respectively.
Additional information
Funding
Notes on contributors
Xingxing Li
Xingxing Li is currently a professor at Wuhan University. He has completed his BSc degree at the School of Geodesy and Geomatics at Wuhan University. He obtained his PhD degree at the Department of Geodesy and Remote Sensing of the German Research Centre for Geosciences (GFZ). His current research mainly involves GNSS precise position and orbit determination, and multi-sensor integrated navigation.
Yujie Qin
Yujie Qin is currently a postgraduate student at Wuhan University. She received her BSc at the School of Geodesy and Geomatics at Wuhan University in 2019. Her research currently focuses on the precise orbit determination for the LEO satellites and the determination for the Earth orientation parameters.
Keke Zhang
Keke Zhang is currently a PhD candidate at Wuhan University. He has completed his BSc at the School of Geodesy and Geomatics in Wuhan University in 2016. His area of research currently focuses on the precise orbit determination for LEO satellites and the integrated processing of LEO and GNSS.
Jiaqi Wu
Jiaqi Wu is currently a PhD candidate at Wuhan University. He has completed his BSc at the School of Geodesy and Geomatics in Wuhan University in 2017. His area of research currently focuses on precise orbit determination for LEO and GNSS satellites and undifference ambiguity resolution.
Wei Zhang
Wei Zhang is currently a PhD candidate at Wuhan University. He has completed his BSc at the School of Geodesy and Geomatics in Wuhan University in 2018. His area of research currently focuses on integrated orbit determination of LEO and GNSS satellites.
Qian Zhang
Qian Zhang received her BSc at the School of Geodesy and Geomatics at Wuhan University in 2018 and obtained her Master’s degree at the School of Geodesy and Geomatics at Wuhan University in 2021. Her research focused on the determination for the Earth orientation parameters.
Hongmin Zhang
Hongmin Zhang is currently a postgraduate student at Wuhan University. She received her BSc at the School of Geodesy and Geomatics at Wuhan University in 2019. Her research currently focuses on the realization of reference frame based on GNSS and SLR.
References
- Allende-Alba, G., and O. Montenbruck. 2016. “Robust and Precise Baseline Determination of Distributed Spacecraft in LEO.” Advances in Space Research 57 (1): 46–63. doi:https://doi.org/10.1016/j.asr.2015.09.034.
- Arnold, D., S. Schaer, A. Villiger, R. Dach, and A. Jäggi 2019. “Single-receiver Ambiguity Fixing for GPS-based Precise Orbit Determination of Low Earth Orbiters Using CODE’s New Clock and Phase Bias Products.” Abstract presented on EGU General Assembly Conference, Vienna, April 7–12.
- Bertiger, W., S.D. Desai, B. Haines, N. Harvey, A.W. Moore, S. Owen, and J.P. Weiss. 2010. “Single Receiver Phase Ambiguity Resolution with GPS Data.” Journal of Geodesy 84 (5): 327–337. doi:https://doi.org/10.1007/s00190-010-0371-9.
- Bisnath, S.B., and R.B. Langley. 1999. “Precise A Posteriori Geometric Tracking of Low Earth Orbiters with GPS.” Canadian Aeronautics and Space Journal 45: 245–252.
- Bock, H., A. Jaggi, U. Meyer, P. Visser, J. van Den Ijssel, T. van Helleputte, M. Heinze, and U. Hugentobler. 2011. “GPS-derived Orbits for the GOCE Satellite.” Journal of Geodesy 85 (11): 807–818. doi:https://doi.org/10.1007/s00190-011-0484-9.
- Dong, D., and Y. Bock. 1989. “Global Positioning System Network Analysis with Phase Ambiguity Resolution Applied to Crustal Deformation Studies in California.” Journal of Geophysical Research Atmosphere 94 (B4): 3949–3966. doi:https://doi.org/10.1029/JB094iB04p03949.
- Förste, C., S. Bruinsma, O. Abrikosov, S. Rudenko, and R. Biancale. 2016. “EIGEN-6S4 A Time-variable Satellite-only Gravity Field Model to D/o 300 Based on LAGEOS, GRACE and GOCE Data from the Collaboration of GFZ Potsdam and GRGS Toulouse.” doi: https://doi.org/10.5880/icgem.2016.008.
- Friis-Christensen, E., H. Lühr, D. Knudsen, and R. Haagmans. 2008. “Swarm - an Earth Observation Mission Investigating Geospace.” Advances in Space Research 41 (1): 210–216. doi:https://doi.org/10.1016/j.asr.2006.10.008.
- Ge, H., B. Li, S. Jia, L. Nie, T. Wu, Z. Yang, J. Shang, Y. Zheng, and M. Ge. 2021. “LEO Enhanced Global Navigation Satellite System (Legnss): Progress, Opportunities, and Challenges.” Geo-spatial Information Science 1–13. doi:https://doi.org/10.1080/10095020.2021.1978277.
- Guo, X., J. Geng, X. Chen, and Q. Zhao. 2019. “Enhanced Orbit Determination for Formation-flying Satellites through Integrated Single- and Double-difference GPS Ambiguity Resolution.” GPS Solutions 24 (1). doi:https://doi.org/10.1007/s10291-019-0932-1.
- Ijssel, J., J. Encarnacao, E. Doornbos, and P. Visser. 2015. “Precise Science Orbits for the Swarm Satellite Constellation.” Advances in Space Research 56 (6): 1042–1055. doi:https://doi.org/10.1016/j.asr.2015.06.002.
- Jäggi, A., U. Hugentobler, H. Bock, and G. Beutler. 2007. “Precise Orbit Determination for GRACE Using Undifferenced or Doubly Differenced GPS Data.” Advances in Space Research 39 (10): 1612–1619. doi:https://doi.org/10.1016/j.asr.2007.03.012.
- Kang, Z., S. Bettadpur, P. Nagel, H. Save, S. Poole, and N. Pie. 2020. “GRACE-FO Precise Orbit Determination and Gravity Recovery.” Journal of Geodesy 94 (9): 1–17. doi:https://doi.org/10.1007/s00190-020-01414-3.
- Landerer, F.W., F.M. Flechtner, H. Save, F.H. Webb, T. Bandikova, W.I. Bertiger, S.V. Bettadpur, et al. 2020. “Extending the Global Mass Change Data Record: GRACE Follow-on Instrument and Science Data Performance.” Geophysical Research Letters 47 (12). doi:https://doi.org/10.1029/2020GL088306.
- Laurichesse, D., F. Mercier, J. Berthias, P. Broca, and L. Cerri. 2009. “Integer Ambiguity Resolution on Undifferenced GPS Phase Measurements and Its Application to PPP and Satellite Precise Orbit Determination.” Navigation 56 (2): 135–149. doi:https://doi.org/10.1002/j.2161-4296.2009.tb01750.x.
- Li, X., X. Han, X. Li, G. Liu, G. Feng, B. Wang, and H. Zheng. 2021. “Great-UPD: An Open-source Software for Uncalibrated Phase Delay Estimation Based on multi-GNSS and Multi-frequency Observations.” GPS Solutions 25 (2). doi:https://doi.org/10.1007/s10291-020-01070-2.
- Li, X., X. Li, F. Ma, Y. Yuan, K. Zhang, F. Zhou, and X. Zhang. 2019b. “Improved PPP Ambiguity Resolution with the Assistance of Multiple LEO Constellations and Signals.” Remote Sensing 11 (4): 408. doi:https://doi.org/10.3390/rs11040408.
- Li, X., F. Ma, X. Li, H. Lv, L. Bian, Z. Jiang, X. Zhang. 2019a. “LEO Constellation-augmented multi-GNSS for Rapid PPP Convergence.” Journal of Geodesy 93 (5): 749–764. doi:https://doi.org/10.1007/s00190-018-1195-2.
- Li, X., J. Wu, K. Zhang, X. Li, Y. Xiong, and Q. Zhang. 2019c. “Real-time Kinematic Precise Orbit Determination for LEO Satellites Using Zero-differenced Ambiguity Resolution.” Remote Sensing 11 (23): 2815. doi:https://doi.org/10.3390/rs11232815.
- Li, X., K. Zhang, Q. Zhang, W. Zhang, Y. Yuan, and X. Li. 2018. “Integrated Orbit Determination of FengYun‐3C, BDS, and GPS Satellites.” Journal of Geophysical Research: Solid Earth 123: 8143–8160. doi:https://doi.org/10.1029/2018JB015481.
- Loyer, S., F. Perosanz, F. Mercier, H. Capdeville, and J.C. Marty. 2012. “Zero-difference GPS Ambiguity Resolution at CNES–CLS IGS Analysis Center.” Journal of Geodesy 86 (11): 991–1003. doi:https://doi.org/10.1007/s00190-012-0559-2.
- Lyard, F., T. Lefevre, F. Letellier, and O. Francis. 2004. “Modelling the Global Ocean Tides: Modern Insights from FES2004.” Journal of Geophysical Research Atmospheres 56 (5): 394–415. doi:https://doi.org/10.1007/s10236-006-0086-x.
- Mao, X., P. Visser, and D. Van. 2019. “High-dynamic Baseline Determination for the Swarm Constellation.” Aerospace Science Van, and Technology 88 (MAY): 329–339. doi:https://doi.org/10.1016/j.ast.2019.03.031.
- Montenbruck, O., S. Hackel, and A. Jaggi. 2018a. “Precise Orbit Determination of the Sentinel-3A Altimetry Satellite Using Ambiguity-fixed GPS Carrier Phase Observations.” Journal of Geodesy 92 (7): 711–726. doi:https://doi.org/10.1007/s00190-017-1090-2.
- Montenbruck, O., S. Hackel, J. van Den Ijssel, and A. Daniel. 2018b. “Reduced Dynamic and Kinematic Precise Orbit Determination for the Swarm Mission from 4 Years of GPS Tracking.” GPS Solutions 22 (3): 79. doi:https://doi.org/10.1007/s10291-018-0746-6.
- Pearlman, M.R., C.E. Noll, E.C. Pavlis, F.G. Lemoine, L. Combrink, J.J. Degnan, G. Kirchner, and U. Schreiber. 2019. “The ILRS: Approaching 20 Years and Planning for the Future.” Journal of Geodesy 93 (11): 2161–2180. doi:https://doi.org/10.1007/s00190-019-01241-1.
- Peng, D.J., and B. Wu. 2009. “Precise Orbit Determination for Jason-1 Satellite Using On-board GPS Data with Cm-level Accuracy.” Chinese Science Bulletin 54 (2): 196–202. doi:https://doi.org/10.1007/s11434-008-0513-0.
- Petit, G., and B. Luzum 2010. “IERS Conventions.” IERS Technical Note.
- Picone, J.M., A.E. Hedin, D.P. Drob, and A.C. Aikin. 2002. “NRLMSISE-00 Empirical Model of the Atmosphere: Statistical Comparisons and Scientific Issues.” Journal of Geophysical Research: Space Physics 107 (A12): SIA 15-1-SIA 15–16. doi:https://doi.org/10.1029/2002ja009430.
- Rebischung, P., and R. Schmid. 2016. “IGS14/igs14.atx: A New Framework for the IGS Products.” AGU Fall Meeting, 12-16 December, 2016, San Francisco, USA.
- Reid, T.G.R., A.M. Neish, T. Walter, and P.K. Enge. 2018. “Broadband LEO Constellations for Navigation.” Navigation 65 (2): 205–220. doi:https://doi.org/10.1002/navi.234.
- Seitz, B., C. Mavrocordatos, H. Rebhan, J. Nieke, and B. Berruti. 2010. “The Sentinel-3 Mission Overview.” Proceedings IEEE International Geoscience & Remote Sensing Symposium, IGARSS 2010, July 25-30, 2010, Honolulu, Hawaii, USA. IEEE.
- Standish, E.M. 1998. “JPL Planetary and Lunar Ephemerides.”
- Tapley, B., S. Bettadpur, J.C. Ries, P. Thompson, and M. Watkins. 2004. “GRACE Measurements of Mass Variability in the Earth System.” Science (New York, N.Y.) 305 (5683): 503–505. doi:https://doi.org/10.1126/science.1099192.
- Vaze, P., S. Neeck, W. Bannoura, J. Green, and F. Parisot. 2010. “The Jason-3 Mission: Completing the Transition of Ocean Altimetry from Research to Operations.” Proceedings of SPIE - the International Society for Optical Engineering 7826 (6): 1351–1359. doi:https://doi.org/10.1117/12.868543.
- Wu, J.T., S.C. Wu, G.A. Hajj, W.I. Bertiger, and S.M. Lichten. 1992. “Effects of Antenna Orientation on GPS Carrier Phase.” Astrodynamics 1991.
- Wu, S.C., T.P. Yunck, and C.L. Thornton. 1988. “Reduced-dynamic Technique for Precise Orbit Determination of Low Earth Satellites.” Journal of Guidance, Control, and Dynamics. doi:https://doi.org/10.2514/3.20600.
- Yunck, T.P., S.C. Wu, J.T. Wu, and C.L. Thorrnton. 1990. “Precise Tracking of Remote Sensing Satellites with the Global Positioning System.” IEEE Transactions on Geoscience Thornton, and Remote Sensing 28 (1): 108–116. doi:https://doi.org/10.1109/36.45753.