Abstract
Estradiol potentiates hypothalamic-pituitary-adrenal activity and delays the return of glucocorticoid secretion to baseline after a stressor exposure in female rats; we investigated whether estradiol effects involve actions on glucocorticoid receptor (GR) translocation and expression of receptor co-chaperones. In Experiment 1 intact females and ovariectomized (OVX) females were treated for four days with vehicle (VEH), 17β-estradiol benzoate (EB), or EB and progesterone (EB + P). Samples were taken from rats in the home cage (baseline) or after 30 min of restraint stress in a plastic restrainer (post-restraint) (n = 10/group). OVX-VEH treatment reduced baseline and post-restraint plasma concentrations of corticosterone versus all other treatments. Western blots indicated that OVX-VEH treated rats had greater hippocampal cytosolic GR expression than other treatments. Stress increased hippocampal nuclear GR expression, but without treatment differences. In Experiment 2 OVX rats were treated daily with VEH, EB, or EB + P (n = 8/group). OVX-VEH rats showed a lower stimulation of corticosterone secretion by restraint stress than other treatments and OVX-EB + P treated rats had lower concentrations than the OVX-EB group, suggesting progesterone mitigated estradiol effects. Quantitative polymerase chain reaction experiments indicated that stress increased Fkbp5 mRNA in the ventral hippocampus, with no effect of stress or treatment on Nr3c1 (GR), Nr3c2 (MR), Fkbp4, Bag1, or Ncoa1 (SRC-1) expression. Thus, the hypothesis is that estradiol effects on negative feedback are mediated by altered expression of receptor co-chaperones or co-modulators in the hippocampus was not supported. Estradiol may blunt feedback by limiting the availability of cytosolic GR protein.
Introduction
The hypothalamic-pituitary-adrenal (HPA) axis regulates the release of glucocorticoids (primarily corticosterone in rats). In response to a stressor, increased HPA signaling leads to greater release of glucocorticoids, which act throughout the body on glucocorticoid and mineralocorticoid receptors (GR and MR, respectively). Increased binding of glucocorticoids at receptor targets alters energy metabolism, immune function, and cognitive processes to cope with the stressor (Sapolsky, Romero, & Munck, Citation2000). Moreover, increased GR binding at each level of the axis and in upstream brain regions (e.g. hippocampus and prefrontal cortex) results in negative feedback, whereby HPA activity is suppressed, facilitating a return to baseline concentrations of glucocorticoids (Herman, McKlveen, Solomon, Carvalho-Netto, & Myers, Citation2012). Whereas brief and infrequent exposures to glucocorticoids can be adaptive for coping with stressors, too much exposure is associated with “wear and tear” on the body (e.g. cardiovascular disease and immune dysfunction) and an increased risk for psychopathologies such as depression (Juster, McEwen, & Lupien, Citation2010; McCormick & Green, Citation2013).
In adult rodents, females have higher baseline and stressor-induced concentrations of corticosterone in the circulation when compared with males (Babb, Masini, Day, & Campeau, Citation2013; Iwasaki-Sekino, Mano-Otagiri, Ohata, Yamauchi, & Shibasaki, Citation2009; Kitay, Citation1961; Seale, Wood, Atkinson, Bate, et al., Citation2004; Viau, Bingham, Davis, Lee, & Wong, Citation2005). Furthermore, after a stressor, females take longer to return to baseline glucocorticoid concentrations than do males, which suggests that females have reduced HPA feedback (Iwasaki-Sekino et al., Citation2009; Kitay, Citation1961; Weinstock, Razin, Schorer-Apelbaum, Men, & McCarty, Citation1998). These sex differences are attributed foremost to circulating concentrations of gonadal hormones; after ovariectomy females tend to respond like gonadally-intact males and estradiol replacement potentiates HPA activity and reduces negative feedback (Burgess & Handa, Citation1992; Kalil, Leite, Carvalho-Lima, & Anselmo-Franci, Citation2013; Kitay, Citation1963; Lunga & Herbert, Citation2004; McCormick, Linkroum, Sallinen, & Miller, Citation2002; Seale, Wood, Atkinson, Bate, et al., Citation2004; Viau & Meaney, Citation1991; Weiser & Handa, Citation2009; Young, Citation1996). The stimulatory effects of estradiol are also observed during ovulatory cycles in females, which have more pronounced and prolonged stress responses during proestrus when concentrations of circulating estradiol are highest, compared with other phases of the cycle (Atkinson & Waddell, Citation1997; Carey, Deterd, de Koning, Helmerhorst, & de Kloet, Citation1995; Viau & Meaney, Citation1991). Estradiol may reduce negative feedback by altering the expression of corticosteroid receptors. For example, estradiol decreases the expression of GR messenger ribonucleic acid (mRNA) in the hypothalamus and hippocampus of ovariectomized rats (Burgess & Handa, Citation1993; Peiffer, Lapointe, & Barden, Citation1991; Seale, Wood, Atkinson, Bate, et al., Citation2004). Moreover, ovariectomy increases the number of cytosolic binding sites for dexamethasone in the hippocampus, hypothalamus, and pituitary of adrenalectomized rats (Turner, Citation1990; Turner & Weaver, Citation1985). Other studies, however, found no effect of estradiol on binding in stress-related brain regions (Burgess & Handa, Citation1992; Ferrini, Magariños, & de Nicola, Citation1990).
The dampening effects of estradiol on HPA feedback may extend beyond the number of available corticosteroid receptors to factors that modulate their assembly and activity. In support of this hypothesis, administration of a GR agonist reduces GR mRNA expression in the hippocampus of ovariectomized rats, but not in females given estradiol replacement, indicating that estradiol interferes with GR autoregulation (Burgess & Handa, Citation1992, Citation1993). A mechanism by which estradiol may alter GR signaling is by reducing transcriptional activity of GR via dephosphorylation (Zhang, Leung, Nordeen, & Goleva, Citation2009) or changing the expression of enhancers/repressors (e.g. SRC-1; Mitev, Wolf, Almeida, & Patchev, Citation2003). There is also evidence that ovarian hormones affect the localization of GR; ovariectomized rats have more nuclear GR in the hippocampus than sham controls when tested at baseline (Sheng et al., Citation2003). Another study found that estradiol produced an anti-translocation profile in hippocampal cell culture; mRNA expression of Fkbp5 and Ppid were up- and down- regulated by estradiol, respectively (Malviya et al., Citation2013). Fkbp5 codes for the FK506-binding protein 51 (Fkbp51), which reduces both GR affinity for its ligands and its ability to translocate to the nucleus, whereas peptidylprolyl isomerase D (Ppid) promotes translocation (Binder, Citation2009; Ratajczak, Ward, Cluning, & Allan, Citation2009; Wochnik et al., Citation2005). Nevertheless, no studies have examined the effects of ovariectomy or of ovarian hormone replacement on GR localization changes in response to an acute stressor, nor have their effects on co-chaperones been investigated in vivo.
To characterize the actions of estradiol on HPA function and GR signaling, we investigated its effects on plasma corticosterone concentration, GR translocation and the expression of genes that code for corticosteroid receptors, co-chaperones, and a co-activator. We administered estradiol alone or in combination with progesterone to ovariectomized females and measured protein and mRNA expression in the hippocampus because of its involvement in negative feedback (Herman et al., Citation2012). Progesterone treatment was included because it was found to mitigate the effects of estradiol on HPA activity and feedback (Viau & Meaney, Citation1991) and can affect corticosteroid receptor binding (Carey et al., Citation1995). We hypothesized that estradiol, alone or in combination with progesterone, would increase baseline and post-stress corticosterone, consistent with past studies, and reduce expression of cytosolic GR in the hippocampus as well as stressor-induced GR translocation. Further, we hypothesized that baseline mRNA expression of corticosteroid receptors would be concomitantly reduced, whereas genes coding for anti-translocation (e.g. Fkbp5 and Bag1 [codes for BCL-2 associated athanogene 1]) and pro-translocation (Fkbp4 [codes for FK506 binding protein 52]) co-chaperones may be augmented and dampened by estradiol treatment, respectively.
Methods
Experiment 1: effects of ovarian hormones on HPA responses and GR translocation
Animals
Adult female Long-Evans rats (n = 100) were obtained from Charles River at approximately postnatal day 60 (P60). Rats remained gonadally-intact (non-ovariectomized [OVX], n = 20) or were bilaterally OVX at P55 by the supplier (n = 80), which involved a small dorsal midline incision and the removal of the ovary and fat pad from the abdominal cavity. The fallopian tubes were cauterized, the ovaries dissected, and the fat pads were returned to the abdominal cavity. Incision sites were closed with surgical staples and surgery was performed under surgical anesthesia with a ketamine/xylazine cocktail. Rats were administered buprenorphine for post-surgical pain relief. Upon arrival, rats were housed in same treatment pairs (described below) and were acclimated to the colony room for one week. All rats were maintained on a 12 h light-dark cycle (lights on at 09:00 am off at 21:00 pm) with food and water available ad libitum. Procedures were in keeping with the National Institutes of Health Guide for Care and Use of Laboratory Animals (Publication No. 85-23, revised 1996) and the Canadian Council on Animal Care guidelines and were approved by the Brock University Animal Care and Use Committee.
Experimental manipulations and sample collection
Although all females were obtained at the same time, they were tested in batches and were 1–2 months post-OVX at the time. Females were assigned randomly to one of five treatments (n = 20 per treatment). OVX females received four days of daily subcutaneous injections of vehicle (VEH), 17β-estradiol benzoate (EB, 25 µg/kg in ∼250 µl of sesame oil vehicle, dosing regimen based on Weiser & Handa (Citation2009), or EB and progesterone (EB + P) (P: .5 mg in 200 µl of sesame oil vehicle, based on McCormick & Singh (Citation1996)) and one group received vehicle on days 1 and 3, EB on day 2, and P on day 4 (EB + P [FD: fewer doses]), which was done to better match the hormonal profile of naturally cycling female. The EB + P(FD) treatment regimen is comparable to that used to produce behavioral estrus in OVX rats (McCormick et al., Citation2013). The non-OVX females received a daily vehicle injection to control for any effects of handling and injection stress. Injections before the test day were given during the lights-on phase (between 11:00 am and 17:00 pm) and on the day of testing, injections and sample collection occurred from 08:00–09:00 am and from 11:00 am–12:00 pm, respectively (restraint stress started as early as 10:30 am).
Trunk blood and brain tissue was collected via rapid decapitation without anesthetic either directly from the home cage (baseline) or after 30 min of restraint (post-stress) in ventilated Plexiglas® flat-bottomed restrainers (8.2 cm diameter x 20.3 cm length; n = 10 per group). Blood samples were collected in ice-chilled glass tubes containing 100 µl of supersaturated EDTA in water and were centrifuged at 4 °C (3000 RCF for 15 min) for the collection of plasma, which was kept at −20 °C until assayed. Brains were extracted rapidly, sliced into 1 mm thick coronal sections using a brain slicing block and placed onto ice-chilled slides, which were immediately frozen on dry-ice. Brain sections were stored at −80 °C until tissue processing.
Hormone assay
Corticosterone and progesterone were extracted from baseline and post-stress plasma samples using diethyl ether and reconstituted in buffer provided in the enzyme-linked immunosorbent assay kits (Neogen, Lansing, MI), which were run according to the kit instructions and quantified using a Biotek Synergy plate reader(Biotek,Winooski, VT). Assay sensitivity was 0.05 ng/mL for corticosterone and 0.4 ng/mL for progesterone. Intra- and inter-assay variabilities were <5 and 12%, respectively for both hormones.
Protein extraction
To determine GR expression in the cytosolic and nuclear compartments, punches were obtained from the hippocampus while on dry-ice, using a tissue puncher (1 mm inner diameter) and tissue from two females of the same treatment and stress condition were pooled together (n = 5 samples per group). Throughout the protein extraction procedure, samples were kept on ice or at 4 °C. An appropriate volume of cold S1 buffer (10 mM HEPES pH 7.5, 1.5 mM MgCl2, 10 mM KCl, 2 mM DDT, 1 mM EDTA, 1 mM EGTA, and 0.5% Triton-X) was added to the samples, which were homogenized in a Bullet Blender® and centrifuged (17,200 RCF for 3 min). The pellet was retained for preparation of the nuclear fraction and the supernatant was collected and re-centrifuged (16,000 RCF for 20 min) and the resulting supernatant was retained as the final cytosolic fraction. To wash the nuclear pellets, samples were re-suspended in the same volume of S1 buffer using a 26.5-gauge needle, vortexed, and centrifuged (17,200 RCF for 3 min). The supernatant was discarded and the pellets were re-suspended and homogenized in ∼1.2 pellet volumes of cold S2 buffer (20 mM HEPES pH 7.5, 400 mM NaCl, 1.5 mM MgCl2, 0.1 mM EDTA, 20% glycerol, and 2 mM DTT), then incubated on ice for one hour during which time the samples were vortexed for 10 s every 10 min. After the incubation, the samples were centrifuged (17,200 RCF for 30 minutes) and the supernatant was retained as the final nuclear fraction. S1 and S2 buffer included a protease inhibitor cocktail (Sigma-Aldrich, St. Louis, MO, 5892791001). The protease inhibitor tablet was prepared as a 2x concentration according to packaging instructions and constituted half of the volume of the buffer needed to bring it to a 1x concentration. Protein concentrations were determined for cytosolic and nuclear fractions using the Bio-Rad Protein Assay procedure (Bio-Rad, Hercules, CA) and a Biotek Synergy plate reader. Samples were brought to an equal concentration using the appropriate buffer and an equal volume of 2x Lammaeli buffer was added. Afterwards, samples were heated at 70 °C for 5 min, centrifuged (16,000 RCF for 10 min), and then stored at −20 °C until Western blot experiments were conducted.
Western blotting
To measure GR translocation, cytosolic and nuclear samples (30 µg of protein each) were resolved on 6% acrylamide gels and transferred to a PVDF membrane. Total protein was measured via Ponceau stain and this was also used to normalize GR values. Membranes were reactivated, washed (3 x 5 min) in Tris-buffered saline with 1% Tween 20 (TBS-T) and blocked for one hour with 5% nonfat milk powder dissolved in TBS-T. Membranes incubated overnight at 4 °C in TBS-T containing 1% milk block and rabbit anti-GR (M20, Santa Cruz; 1:500 and 1:200 for cytosolic and nuclear samples, respectively). Next day, membranes were washed and then incubated for 45 min at room temperature in TBS-T containing 1% milk block and goat fluorescent anti-rabbit antibody (Life Technologies, Carlsbad, CA AlexaFluor 488; 1:5000), washed again and then were imaged using a Bio-Rad VersaDoc (MP 4000). Samples from each condition and stress condition (i.e. baseline or post-stress) were counterbalanced within and across gels.
To validate the subcellular fractionation protocol, cytosolic, and nuclear samples (10 µg of protein each) were resolved on a 12% acrylamide gels, blocked with 5% BSA, and probed with rabbit anti-histone H3 antibody (D1H2; Cell Signaling; 1:1000) and rabbit anti-GAPDH antibody (14C10; Cell Signaling; 1:1000) in TBS-T with 1% BSA. Next day, membranes were washed and then incubated for 45 min at room temperature in TBS-T containing 1% milk block and goat anti-rabbit fluorescent antibody (Life Technologies, AlexaFluor 488; 1:5000), washed again and then were imaged using a Bio-Rad VersaDoc (MP 4000).
Experiment 2: effects of ovarian hormones on HPA responses and gene expression
Animals
Female Long-Evans rats (N = 48) were OVX by the supplier (Charles River), as described above for Experiment 1 and arrived approximately nine days later at P60 ± 2 days. Rats were housed in same-treatment pairs (described below), kept on a 12 h light-dark cycle (lights on at 09:00 am, off at 21:00 pm) and were given free access to food and water. All procedures were in keeping with the National Institutes of Health Guide for Care and Use of Laboratory Animals (Publication No. 85-23, revised 1996) and the Canadian Council on Animal Care guidelines, and were approved by the Brock University Animal Care and Use Committee.
Experimental manipulations and sample collection
Seven days after the OVX females arrived, they were randomly assigned to one of three treatments (n = 16 per treatment), which involved four days of daily subcutaneous injections of vehicle (VEH), 17β-estradiol benzoate (EB), or EB and progesterone (EB + P); doses were the same as used in Experiment 1. Thus, females had been ovariectomized for 20 days at the time of testing. Experiment 2 did not include non-OVX and OVX-EB + P(FD) females, because these groups did not differ significantly from the OVX-EB + P group for corticosterone concentrations in Experiment 1. Injections before the test day were given during the lights-on phase (between 11:00 am and 17:00 pm) and on the day of testing, injections and sample collection occurred from 08:00–09:00 am and from 11:00 am–14:00 pm (stress started as early as 10:30 am), respectively.
Half of the females in each treatment group were rapidly decapitated directly from the home cage (baseline) for the collection of trunk blood and brain tissue, as in Experiment 1. The other half of the rats underwent 30 min of restraint in Plexiglas® restrainers. Blood was collected from these females at 0, 45, and 90 min, respectively after the 30 min of restraint stress ended (first two samples from the tail vein, 90 min sample from trunk blood; brains were also collected); rats were returned to home cages between collections. The first tail vein sample (0 min post-stress) was obtained by nicking the end of the tail ( ∼1–2 mm) using surgical scissors, and the second sample (45 min post-stress) was obtained by dislodging the clot that formed after the tail nick (handling and collection of tail vein samples occurred within 2 min of removal from the restrainer and the home-cage for the first and second post-stress collection time-points, respectively). Blood was collected into ice-chilled tubes containing EDTA, centrifuged at 4 °C (3000 RCF for 10 min) and plasma was stored until assayed (see methods Experiment 1). These time-points were selected based on previous evidence that adult OVX females have intermediate circulating concentrations of corticosterone 45 min after termination of the stressor was compared with baseline and post-stress concentrations, which reflects partial recovery at this time-point (Romeo, Lee, & McEwen, Citation2004).
RT-qPCR
Approximately 6–8 punches (1 mm inner diameter) from 1 mm coronal sections were collected on dry-ice from the ventral hippocampus and total RNA was extracted using a Fatty Tissue RNA Purification Kit (Norgen Biotek Corp., Canada) as per kit instructions. RNA concentration and quality was assessed using a Nanodrop and samples (500 ng of RNA) were reverse transcribed using an iScript cDNA Synthesis Kit (Bio-Rad Laboratories). Primers were designed to measure mRNA expression for genes of interest (). Samples were run in triplicate using a CFX Connect Real-Time System and SYBR green (Bio-Rad Laboratories) and were counterbalanced within and across plates. Gene expression was normalized to ubiquitin C (Ubc), hypoxanthine phosphoribosyltransferase 1 (Hprt1), and peptidylprolyl isomerase A (Ppia), which demonstrated good stability and did not differ with stress condition or treatment. Three baseline (2 VEH, 1 EB) and three post-stress (2 VEH, 1 EB) brains were compromised during collection and were thus excluded from the gene expression studies (resulting in n = 6–8 per group).
Table 1. Sequences of primers used in RT-qPCR reactions.
Statistical analyses
For Experiment 1, we used between-subjects (five treatment x two stress condition) ANOVAs on concentrations of corticosterone and progesterone and on cytosolic and nuclear GR expression in the hippocampus. For Experiment 2, between-subjects (three treatment x two stress condition) ANOVAs were used for hippocampal mRNA expression of various stress-related genes. Baseline corticosterone concentrations were analyzed with a one-way (three treatment) ANOVA. Post-restraint corticosterone concentrations were analyzed with a mixed-model (three treatment x three time-point) ANOVA. Significant interactions were followed up with simple effects analyses where appropriate and post-hoc tests were Bonferroni-corrected with statistical significance set at p < .05.
Results
Experiment 1: effects of ovarian hormones on HPA responses and GR translocation
Corticosterone
There was a significant effect of treatment (F4,90 = 4.7, p = .002) and stress (F1,90 = 335.2, p < .0001) on plasma corticosterone concentrations, and no interaction of the two factors (F4,90 = 1.4, p = .26). As expected, stress increased corticosterone concentrations across all treatments. Moreover, OVX-VEH females had lower plasma concentrations of corticosterone compared with non-OVX females and with OVX-EB, OVX-EB + P, and OVX-EB + P(FD) females (all p < .05, ). The lower corticosterone concentration in OVX-VEH females was irrespective of stress condition and consistent with past evidence of a stimulatory effect of estradiol on baseline and post-stress concentrations.
Figure 1. Mean (± S.E.M.) plasma concentrations of (A) corticosterone and (B) progesterone and of hippocampal expression of GR in (C) cytosol and (D) nucleus at baseline (light-gray bars) or post-stress (dark-gray bars) in gonadally-intact (non-ovariectomized [OVX]) females and in OVX females from different treatment groups; OVX females received four daily injections of either vehicle (VEH), estradiol benzoate (EB), EB and progesterone (EB + P), or EB + P (FD; fewer doses; to mimic release in naturally cycling females). Analyses involved between-subjects (five treatment x two stress condition) ANOVAs. Representative western blots are shown below the graphs in (C) and (D). (A) indicates less than all other treatment groups irrespective of stress condition (all p < 0.05 for OVX-VEH compared with all other groups). (B) gray symbols indicate within baseline comparisons of treatment effects and black symbols indicate post-stress comparisons of treatment effects; * indicates less than non-OVX rats; @ indicates greater than OVX-VEH rats; # indicates greater than OVX-EB rats (only statistically significant groups differences are pointed out, all p < 0.05). (C) ^ indicates a significant difference between baseline and post-stress within a treatment group (p = 0.026), and the lines above bars indicate differences between OVX-VEH and OVX-EB or OVX-EB + P (all p < 0.05). (D) + indicates an effect of stress irrespective of treatment group (p = 0.001). For (A) and (B), n = 10 per group. For (C) and (D), n = 5 per group, except for baseline OVX-EB + P and post-stress non-OVX [n = 4], because two samples were lost during extraction).
![Figure 1. Mean (± S.E.M.) plasma concentrations of (A) corticosterone and (B) progesterone and of hippocampal expression of GR in (C) cytosol and (D) nucleus at baseline (light-gray bars) or post-stress (dark-gray bars) in gonadally-intact (non-ovariectomized [OVX]) females and in OVX females from different treatment groups; OVX females received four daily injections of either vehicle (VEH), estradiol benzoate (EB), EB and progesterone (EB + P), or EB + P (FD; fewer doses; to mimic release in naturally cycling females). Analyses involved between-subjects (five treatment x two stress condition) ANOVAs. Representative western blots are shown below the graphs in (C) and (D). (A) indicates less than all other treatment groups irrespective of stress condition (all p < 0.05 for OVX-VEH compared with all other groups). (B) gray symbols indicate within baseline comparisons of treatment effects and black symbols indicate post-stress comparisons of treatment effects; * indicates less than non-OVX rats; @ indicates greater than OVX-VEH rats; # indicates greater than OVX-EB rats (only statistically significant groups differences are pointed out, all p < 0.05). (C) ^ indicates a significant difference between baseline and post-stress within a treatment group (p = 0.026), and the lines above bars indicate differences between OVX-VEH and OVX-EB or OVX-EB + P (all p < 0.05). (D) + indicates an effect of stress irrespective of treatment group (p = 0.001). For (A) and (B), n = 10 per group. For (C) and (D), n = 5 per group, except for baseline OVX-EB + P and post-stress non-OVX [n = 4], because two samples were lost during extraction).](/cms/asset/d15fa5e6-8ba9-40a9-9245-8ffb9d441399/ists_a_1409719_f0001_b.jpg)
Progesterone
There was a main effect of treatment (F4,90 = 39.2, p < .001) and stress (F1,90 = 55.0, p < .001) on progesterone concentrations, and an interaction between the two factors (F4,90 = 2.5, p = .046). Follow up analyses indicated that concentrations of progesterone were lower at baseline compared with post-stress in all groups (all p < .05), except for OVX-EB + P (p = .18). In baseline samples, non-OVX females had higher progesterone concentrations than did all other groups (all p < .05, ) except OVX-EB + P (p = 1.0). Moreover, OVX-EB + P females did not differ from OVX-EB + P(FD) (p = .66), but they did have higher concentrations than OVX-EB (p = .04) and OVX-VEH (p < .001) females. OVX-EB + P(FD) also had higher concentrations than did OVX-VEH females (p = .007), but did not differ from OVX-EB females (p = 1.0). For post-stress samples, the same treatment differences were observed as at baseline (all p < .05), except non-OVX females had higher concentrations of progesterone than did all other groups (all p < .05).
GR translocation
There was a significant effect of treatment (F4,38 = 3.2, p = .02) on cytosolic GR expression in the hippocampus, but no effect of stress (F1,38 = 1.2, p = .28), nor an interaction between the two factors (F4,38 = 1.7, p = .18). Post hoc tests indicated that OVX-VEH treated females had more cytosolic GR than OVX-EB and OVX-EB + P treated females (all p < .05; ). Although the omnibus interaction was not significant, likely because of reduced power from small group sizes (n = 4–5 per treatment and stress condition) and the 5 x 2 design, separate analyses for each stress condition indicated the effect of treatment was driven by differences at baseline (F4,19 = 6.2, p = .002) rather than post-stress (F4,19 = 0.2, p = .9). Moreover, compared with baseline, stress decreased cytosolic GR in OVX-VEH females only (t8 = 2.7, p = .03). There was evidence of GR translocation in all groups on the basis of increased nuclear GR expression in post-stress compared with baseline samples (main effect of stress, F1,38 = 14.3, p = .001; ). The effect of treatment (F4,38 = 0.3, p = .9) and the interaction of treatment and stress (F4,38 = 0.4, p = .83) were not significant.
Experiment 2: effects of ovarian hormones on HPA responses and gene expression
Corticosterone
Although the pattern of differences paralleled those in experiment 1, the effect of treatment on plasma corticosterone concentrations at baseline was not statistically significant (F2,21 = 2.7, p = .09). For post-stress samples, based on previous studies, we predicted that estradiol treatment would increase corticosterone concentrations in response to stress and would slow the recovery to baseline compared to vehicle treatment and that progesterone would mitigate the effect of estradiol (Burgess & Handa, Citation1992; Viau & Meaney, Citation1991; Weiser & Handa, Citation2009). There was an effect of treatment (F2,21 = 5.8, p = .01) and of time-point (F2,42 = 221.3, p < .0001) on corticosterone concentrations after restraint, as well as an interaction between the two factors (F4,42 = 9.6, p < .001). Follow-up analyses at each time-point indicated that at 0 min post-stress, OVX-VEH treated females had lower concentrations of corticosterone than the OVX-EB (p < .001) and OVX-EB + P (p = .034) groups, and the OVX-EB + P group had lower concentrations than the OVX-EB group (p = .012; ). Thus, progesterone mitigated some of the stimulatory effects of EB. There was no effect of treatment at 45 or 90 min post-restraint (only the higher corticosterone concentration 45 min after restraint in OVX-EB + P females compared with OVX-VEH females was marginally significant, p = .053).
Figure 2. Mean (± S.E.M.) plasma concentrations of corticosterone collected immediately on removal from the home cage (baseline, left), or in separate groups of rats from repeated sampling taken at 0, 45, and 90 min after 30 min of restraint stress. Rats were all ovariectomized (OVX) females in different treatment groups; OVX females received four daily injections of vehicle (VEH), estradiol benzoate (EB), or EB and progesterone (EB + P). Data were analyzed by one-way ANOVA on baseline concentrations (n = 8 per group) and a mixed-model (three treatment [between-subjects] × three time-point [repeated measures]) ANOVA for post-stress concentrations (n = 8 per group). * indicates a significant effect of treatment within that time-point and the bars indicate which groups differ; at 0 min post-stress, OVX-VEH had lower concentrations than OVX-EB + P (p = .034) and OVX-EB (p < .001), and OVX-EB + P had lower concentrations than OVX-EB (p = .012).
![Figure 2. Mean (± S.E.M.) plasma concentrations of corticosterone collected immediately on removal from the home cage (baseline, left), or in separate groups of rats from repeated sampling taken at 0, 45, and 90 min after 30 min of restraint stress. Rats were all ovariectomized (OVX) females in different treatment groups; OVX females received four daily injections of vehicle (VEH), estradiol benzoate (EB), or EB and progesterone (EB + P). Data were analyzed by one-way ANOVA on baseline concentrations (n = 8 per group) and a mixed-model (three treatment [between-subjects] × three time-point [repeated measures]) ANOVA for post-stress concentrations (n = 8 per group). * indicates a significant effect of treatment within that time-point and the bars indicate which groups differ; at 0 min post-stress, OVX-VEH had lower concentrations than OVX-EB + P (p = .034) and OVX-EB (p < .001), and OVX-EB + P had lower concentrations than OVX-EB (p = .012).](/cms/asset/2d7c3035-1d4f-4e0e-b40e-80e3f1ae97e3/ists_a_1409719_f0002_b.jpg)
Gene expression
Contrary to our predictions, there was no effect of treatment on mRNA expression of Nr3c1 (GR), Nr3c2 (MR), Ncoa1 (SRC-1), Fkbp5, Fkbp4, or Bag1 (all p > .25; ). Moreover, treatment did not interact with stress on the expression of the genes of interest (all p > .5). There was, however, a greater expression of Fkbp5 post-stress compared with baseline that was irrespective of treatment (F2,36 = 4.8, p = .035; ; see for an example of amplification and melt curve data).
Figure 3. Relative mRNA expression (S.E.M.) in the hippocampus of selected stress-related genes at baseline (light-gray bars) and 90 min after 30 min of restraint stress (post-stress) with 1.0 set to OVX-VEH (ovariectomized + vehicle injections) at baseline. Analyses for each gene of interest involved a between-subjects (three treatment x two stress condition) ANOVA (for both baseline and post-stress conditions, OVX-VEH: n = 6; OVX-EB: ovariectomized + estradiol benzoate n = 7; and OVX-EB + P (progesterone): n = 8). OVX females received treatments as four daily injections. * indicates a significant effect of stress (p = .035) irrespective of treatment.
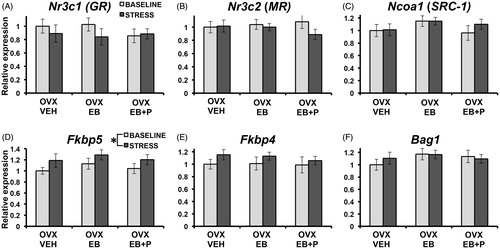
Figure 4. Amplification of Fkbp5 cDNA and corresponding melt curve data. We ran three no reverse transcriptase controls and three no template controls, which did not amplify, indicating primer specificity for the amplicon and an absence of primer dimerization. RFU: relative fluorescent units; -d(RFU)dt: change in fluorescence with temperature.
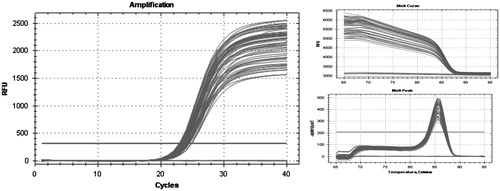
Discussion
We hypothesized that estradiol would increase HPA activity and that we would see a corresponding reduction in GR expression and activity, which we assessed by measuring cytosolic and nuclear GR in the hippocampus before and after acute restraint stress. Consistent with the literature (Handa & Weiser, Citation2014) and with our hypothesis, ovariectomy reduced baseline and post-stress concentrations of corticosterone relative to gonadally-intact females and treatment of estradiol reversed the effect of ovariectomy. Moreover, ovariectomy increased cytosolic GR relative to several estradiol-treated groups and this group was the only one to have a stress-induced decrease in cytosolic GR, which supported our hypothesis that estradiol restricts GR expression and activity. As expected, stress increased nuclear GR expression, but contrary to our hypothesis we did not see an effect of estradiol treatment. Therefore, we found partial evidence that estradiol reduces feedback by restricting GR expression and activity. Contrary to our hypotheses, there were no differences in the expression of genes that code for corticosteroid receptors or co-chaperones and co-activators known to modulate corticosteroid receptor activity.
Although this study and many others found that estradiol potentiated HPA activity, some studies have yielded contradictory results. For example, some reported no effect of estrous cycle phase on circulating adrenocorticotropic hormone (ACTH) or on corticosterone concentrations after stress (Babb et al., Citation2013; Rivier, Citation1999). Others found that estradiol replacement had no effect (Serova, Harris, Maharjan, & Sabban, Citation2010) or reduced (Ferreira-Silva, Helena, Franci, Lucion, & Anselmo-Franci, Citation2009) concentrations of corticosterone in ovariectomized rats. The most conflicting results, however, are related to post-stress concentrations of ACTH, with several studies reporting increases (Burgess & Handa, Citation1992; Carey et al., Citation1995; Leśniewska, Miśkowiak, Nowak, & Malendowicz, Citation1990; Seale, Wood, Atkinson, Harbuz, & Lightman, Citation2004; Viau & Meaney, Citation1991; Weiser & Handa, Citation2009) and decreases (Babb et al., Citation2013; Figueiredo, Ulrich-Lai, Choi, & Herman, Citation2007; Larkin et al., Citation2010; Redei et al., Citation1994; Young, Altemus, Parkinson, & Shastry, Citation2001) in rats given estradiol. Discrepancy in results may involve the time of sample collection after stress given that ACTH is released in pulses and is rapidly metabolized after its release (Reith & Neidle, 1981). Additional variability may stem from differences in the time between ovariectomy and treatment, as well as the dosage and duration of hormone treatment. For example, some studies administered estradiol at the time of surgery (Babb et al., Citation2013; Burgess & Handa, Citation1992; Seale, Wood, Atkinson, Harbuz, et al., Citation2004), whereas others waited until 6 weeks after ovariectomy (Redei et al., Citation1994). Further, some studies used doses that would exceed levels typically found in proestrus (Carey et al., Citation1995; Viau & Meaney, Citation1991) or utilized long treatment durations (Burgess & Handa, Citation1992). One strength of the current study is that we compared stress responses of gonadally-intact females with those of ovariectomized rats that received one of several ovarian replacement regimens, which were delivered over a brief period (4 days). Consistent with the general literature, we found that estradiol increased the stress response whether it was administered daily or once only 48 hours before sample collection.
Actions of estradiol on HPA activity occur in the periphery as well as in the brain. Estradiol increases adrenal content and weight (Kitay, Citation1963; Leśniewska et al., Citation1990; Lunga & Herbert, Citation2004; McCormick et al., Citation2002) as well as adrenal sensitivity to ACTH (Atkinson & Waddell, Citation1997; Figueiredo et al., Citation2007). Stimulatory effects of estradiol on HPA function are observed when administered to the paraventricular nucleus (PVN) (Liu et al., Citation2012; Weiser & Handa, Citation2009) or to the medial preoptic area (McCormick et al., Citation2002). When selective estrogen receptor agonists were administered to the PVN of the hypothalamus, ERα agonists increased, whereas ERβ agonists decreased, HPA activity (Liu et al., Citation2012; Weiser & Handa, Citation2009). Thus, the stimulatory effects of estradiol on HPA activity seem to be mediated by ERα, possibly in GABAergic neurons of the peri-PVN (given that the parvocellular portion is essentially devoid of ERα (Handa & Weiser, Citation2014; Miller W.J., Suzuki, Miller, L.K., Handa, & Uht, Citation2004) and in upstream brain regions.
In addition to potentiating HPA activity, there are many reports that estradiol impaired negative feedback as evidenced by delayed recovery after stress and reduced suppression of corticosterone secretion after treatment with a GR agonist (Burgess & Handa, Citation1992; Carey et al., Citation1995; Viau & Meaney, Citation1991; Weiser & Handa, Citation2009). Dampening of HPA feedback by estradiol also appears to be mediated by ERα, at least near the PVN, and occurs at baseline and after stress (Weiser & Handa, Citation2009), which means that the stimulatory effects of estradiol in the brain may involve reduced suppression rather than enhanced drive. Little is known, however, regarding the downstream mechanisms of estradiol-induced suppression or its actions in other brain regions important for negative feedback, but changes in corticosteroid receptors or their activity may be involved. Unexpectedly, we found no evidence of estradiol-induced suppression of HPA feedback in the current study, perhaps because samples were obtained at 45 min after acute stress; at an earlier recovery time-point, differences may have emerged. We did, however, find that ovariectomy increased the expression of cytosolic GR in the hippocampus, which may allow for enhanced GR-mediated feedback. The greater baseline densities of cytosolic GR in the hippocampus may be because of the lower baseline plasma corticosterone concentrations in the ovariectomized, non-replaced females compared with the other groups. Although one study showed a trend for ovariectomy to increase GR binding capacity for corticosterone in the hippocampus (Turner & Weaver, Citation1985), others found no effect of estradiol on binding capacity for GR agonists (Burgess & Handa, Citation1992) or an increase in the septum and hypothalamus (Ferrini & de Nicola, Citation1991).
Reports that ovariectomy increased nuclear GR (Sheng et al., Citation2003) and that estradiol treatment produced an anti-translocation profile of co-chaperones in cell culture (Malviya et al., Citation2013) led us to investigate GR translocation. Consistent with our predictions, only the ovariectomized, non-replaced group showed a stress-induced reduction of cytosolic GR in the hippocampus, which indicates that estradiol may restrict GR translocation. Thus, when we examined nuclear GR, we expected to find a concomitant increase in stress-induced translocation in ovariectomized, non-replaced females compared with the other conditions, but the results indicated a similar increase across groups. One possible explanation for why ovariectomized females had greater stress-induced GR translocation than the other groups based on differences in the cytosol and not in the nucleus is that ovariectomy may have promoted GR trafficking to other cellular compartments (e.g. the cell membrane). Although corticosterone treatment increased membrane-bound GR in a hypothalamic cell line (Deng, Waxse, Riquelme, Zhang, & Aguilera, Citation2015), it is unclear what role estradiol plays, if any, in affecting GR trafficking to the membrane. Another possibility is that our experiment was sensitive to detect group differences in stress-induced changes in the cytosol and not in the nucleus because GR is predominantly located in the nucleus even at baseline (Sheng et al., Citation2003). Nevertheless, our study seems to be the first to examine effect of estradiol on GR translocation following acute challenge in vivo, but our translocation experiment involved small group sizes and as such warrants replication.
Actions of estradiol on HPA stress responses may involve MR; for example, one study found that estradiol reduced MR binding capacity (Carey et al., Citation1995). Unlike GR, however, MR are mostly bound under basal corticosterone conditions and thus we did not expect to see prominent stress-induced changes in MR that would be affected by gonadal hormones. Moreover, others have found more evidence of actions of estradiol on GR than on MR (Burgess & Handa, Citation1992, Citation1993). It would be worthwhile, however, to investigate phosphorylation of corticosteroid receptors, which can induce translocation and increase their transcriptional activity when the receptors are unbound (Hapgood, Avenant, & Moliki, Citation2016). In support of this, a previous study found that estradiol dephosphorylates GR in breast cancer cell lines (Zhang et al., Citation2009). Thus, it is unclear whether the stress-induced decrease in cytosolic GR in ovariectomized females without sex steroid replacement reflects greater GR binding, phosphorylation, or both. Given that ovariectomized females also released less corticosterone than gonadally-intact females and ovariectomized females treated with estradiol alone or in combination with progesterone, we may have seen a greater difference had the untreated ovariectomized rats been given corticosterone to simulate concentrations in the other groups.
Based on the greater amount of GR protein in the hippocampus of ovariectomized rats, not given estradiol or progesterone, relative to the other groups, we expected to see a concomitant increase in corticosteroid receptor mRNA at baseline in ovariectomized rats, without replacement, relative to estradiol replaced rats. Nevertheless, there were no group differences in either GR or MR mRNA expression at baseline. Previous studies have yielded mixed results with some finding that estradiol treatment reduced MR, but not GR, in the hippocampus (Carey et al., Citation1995; Peiffer et al., Citation1991) and others found a decrease in both MR and GR (Burgess & Handa, Citation1993). Moreover, one study found that, relative to sham-operated controls, ovariectomy reduced GR, but not MR mRNA in the hippocampus (Patchev & Almeida, Citation1996). Thus, effects of ovariectomy and of estradiol replacement on corticosteroid receptor transcript remain inconsistent and may not be a good measure for actions on receptor protein levels or activity.
The decrease in cytosolic GR post-stress compared with baseline in ovariectomized females, which we interpreted as evidence of translocation, was not accompanied by differences in baseline mRNA expression of co-chaperones (Fkbp5, Fkbp4, and Bag1) that are known to modulate GR assembly and trafficking. This lack of difference contradicts previous work in hippocampal cell culture that found estradiol produced a GR anti-translocation profile of gene expression (Malviya et al., Citation2013). Thus, more investigation into actions of estradiol on protein expression of co-chaperones and their interactions with corticosteroid receptors in vivo is required to elucidate their role, if any, on receptor dynamics and negative feedback. Unexpectedly, we did not find that estradiol altered the effect of acute stress on the expression of any of the genes we investigated. This result conflicts with a previous report that estradiol prevented the reduction in hippocampal GR mRNA and protein (as evidenced by binding assay, which could reflect receptor densities and/or changes in their affinity for agonists) that was found in ovariectomized, non-replaced females treated with a GR agonist (Burgess & Handa, Citation1992, Citation1993). One possible explanation is that our post-stress time-point (90 min after restraint ended) was too early or too late to observe an interaction between stress and estradiol treatment. This time-point was selected based on findings that acute forced swim stress tended to increase similar measures in the hippocampus between 30 and 120 min after exposure (Bourke et al., Citation2013). Although estradiol has been shown to alter the expression of the steroid receptor co-activator, Ncoa1 (SRC-1), in a region-specific manner (Mitev et al., Citation2003), we did not observe any group differences, consistent with a previous study showing that ovariectomy did not affect its expression in the hippocampus (Zhang et al., Citation2011).
Although the effects of ovariectomy are attributed to the reduction of circulating estradiol in females, progesterone is also released from the ovaries and may mitigate some of the effects of estradiol on HPA activity (Viau & Meaney, Citation1991) or have suppressing actions on its own (Figueiredo et al., Citation2007). Hence, we examined the effects of progesterone co-treatment with estradiol compared with no replacement or estradiol alone. The results from Experiment 2 supported past reports that progesterone partially mitigates stimulatory effects of estradiol. In Experiment 1, however, progesterone co-treatment had no effect. Others found that progesterone had no effect on HPA responses to stress (Ferreira-Silva et al., Citation2009; Young et al., Citation2001). Thus, it remains unclear whether progesterone treatment buffers the stress response in females and if so, what mechanisms are involved. One study found that, in adrenalectomized and ovariectomized females given corticosterone replacement, progesterone treatment increased GR mRNA in the hippocampus, which may promote negative feedback (Patchev & Almeida, Citation1996). In the current study, however, progesterone did not alter GR mRNA or protein expression in this region. We did find, however, that stress increased the release of progesterone, which is consistent with other studies (Kalil et al., Citation2013; Simone & McCormick, Citation2017). Moreover, although the difference was not statistically significant (likely because of the number of comparisons), estradiol treatment tended to increase progesterone concentrations relative to ovariectomized females without estradiol, possibly by enhancing synthesis and decreasing degradation (Munabi, Cassorla, Pfeiffer, Albertson, & Loriaux, Citation1983). Thus, estradiol-induced increases in progesterone may mitigate the direct stimulatory effects of estradiol in gonadally-intact females. Others, however, have found no effect of ovariectomy or estradiol replacement on progesterone release (Kalil et al., Citation2013).
Estradiol has been found to suppress estrogen receptor expression in the brain, although the effects are likely region-specific (Blaustein, Citation1993; Lauber, Mobbs, Muramatsu, & Pfaff, Citation1991; Osterlund, Kuiper, Gustafsson, & Hurd, Citation1998), and estradiol and progesterone can regulate expression of each other’s receptor (Blaustein & Brown, Citation1984; Romano, Krust, & Pfaff, Citation1989). Thus, the prolonged delay between ovariectomy and the administration of ovarian hormones (1–2 months in Experiment 1 and 2–3 weeks in Experiment 2), as well as the four daily injections of estradiol alone or in combination with progesterone, may have altered estrogen receptor expression in the brain compared with intact females. This may be a potential limitation of Experiment 2, which did not include intact females on the basis that their corticosterone concentrations were similar to those that received estradiol or estradiol and progesterone in Experiment 1. This may explain why an acute effect of stress was only observed for Fkbp5 mRNA expression and not for any other genes of interest (e.g. Nr3c1 (GR), Bag1, and Ncoa1 (SRC-1), which are up-regulated by acute stress in intact females (Bourke et al., Citation2013). Consistent with our Fkbp5 result in females, other studies have found evidence of restraint- or dexamethasone-induced increases in Fkbp5 mRNA in stress-related brain regions (e.g. hippocampus, PVN, and amygdala) of male rodents (Green, Nottrodt, Simone, & McCormick, Citation2016; Scharf, Liebl, Binder, Schmidt, & Müller, Citation2011).
In summary, our results show that as little as one administration of estradiol 48 h before sample collection can increase stress-induced corticosterone release. Although we provide evidence that ovariectomy increases the amount of cytosolic GR receptors in the hippocampus, we found no effect of ovariectomy or of ovarian hormone replacement on changes in nuclear GR expression in response to an acute stressor or on mRNA expression of co-chaperones that are known to modulate ligand binding and receptor trafficking within the hippocampus. Although effects of estradiol on increasing corticosterone release are attributable to actions at ERα (Liu et al., Citation2012; Weiser & Handa, Citation2009), the downstream mechanisms for the effects on stress-induced increases and recovery remain to be elucidated.
Disclosure statement
The authors report no conflict of interest.
Additional information
Funding
References
- Atkinson, H.C., & Waddell, B.J. (1997). Circadian variation in basal plasma corticosterone and adrenocorticotropin in the rat: Sexual dimorphism and changes across the estrous cycle. Endocrinology, 138, 3842–3848. doi:10.1210/endo.138.9.5395
- Babb, J.A., Masini, C.V., Day, H.E., & Campeau, S. (2013). Sex differences in activated corticotropin-releasing factor neurons within stress-related neurocircuitry and hypothalamic-pituitary-adrenocortical axis hormones following restraint in rats. Neuroscience, 234, 40–52. doi:10.1016/j.neuroscience.2012.12.051
- Binder, E.B. (2009). The role of FKBP5, a co-chaperone of the glucocorticoid receptor in the pathogenesis and therapy of affective and anxiety disorders. Psychoneuroendocrinology, 34, S186–S195. doi:10.1016/j.psyneuen.2009.05.021
- Blaustein, J.D. (1993). Estrogen receptor immunoreactivity in rat brain: Rapid effects of estradiol injection. Endocrinology, 132, 1218–1224. doi:10.1210/en.132.3.1218
- Blaustein, J.D., & Brown, T.J. (1984). Progesterone decrease the concentration of hypothalamic and anterior pituitary estrogen receptors in ovariectomized rats. Brain Research, 304, 225–236. doi:10.1016/0006-8993(84)90325-1
- Bourke, C.H., Raees, M.Q., Malviya, S., Bradburn, C.A., Binder, E.B., & Neigh, G.N. (2013). Glucorticoid sentisizers Bag1 and Ppid are regulated by adolescent stress in a sex-dependent manner. Psychoneuroendocrinology, 38, 84–93. doi:10.1016/j.psyneuen.2012.05.001
- Burgess, L.H., & Handa, R.J. (1992). Chronic estrogen-induced alterations in adrenocorticotropin and corticosterone secretion, and glucocorticoid receptor-mediated functions in female rats. Endocrinology, 131, 1261–1269. doi:10.1210/endo.131.3.1324155
- Burgess, L.H., & Handa, R.J. (1993). Estrogen-induced alterations in the regulation of mineralocorticoid and glucocorticoid receptor messenger RNA expression in the female rat anterior pituitary gland and brain. Molecular and Cellular Neuroscience, 4, 191–198. doi:10.1006/mcne.1993.1023
- Carey, M.P., Deterd, C.H., de Koning, J., Helmerhorst, F., & de Kloet, E.R. (1995). The influence of ovarian steroids on hypothalamic-pituitary-adrenal regulation in the female rat. Journal of Endocrinology, 144, 311–321. doi:10.1677/joe.0.1440311
- Deng, Q., Waxse, B., Riquelme, D., Zhang, J., & Aguilera, G. (2015). Helix 8 of the ligand binding domain of the glucocorticoid receptor (GR) is essential for ligand binding. Molecular and Cellular Endocrinology, 408, 23–32. doi:10.1016/j.mce.2015.01.044
- Ferreira-Silva, I.A., Helena, C.V., Franci, C.R., Lucion, A.B., & Anselmo-Franci, J.A. (2009). Modulatory role of locus coeruleus and estradiol on the stress response of female rats. Endocrine, 35, 166–176. doi:10.1007/s12020-008-9139-x
- Ferrini, M., & de Nicola, A.F. (1991). Estrogens up-regulate type I and type II glucocorticoid receptors in brain regions from ovariectomized rats. Life Science, 48, 2593–2601. doi:10.1016/0024-3205(91)90617-K
- Ferrini, M., Magariños, A.M., & de Nicola, A.F. (1990). Oestrogens down-regulate type I but not type II adrenal corticoid receptors in rat anterior pituitary. Journal of Steroid Biochemistry, 35, 671–677. doi:10.1016/0022-4731(90)90307-E
- Figueiredo, H.F., Ulrich-Lai, Y.M., Choi, D.C., & Herman, J.P. (2007). Estrogen potentiates adrenocortical responses to stress in female rats. American Journal of Physiology-Endocrinology and Metabolism, 292, E1173–E1182. doi:10.1152/ajpendo.00102.2006
- Green, M.R., Nottrodt, R.E., Simone, J.J., & McCormick, C.M. (2016). Glucocorticoid receptor translocation and expression of relevant genes in the hippocampus of adolescent and adult male rats. Psychoneuroendocrinology, 73, 32–41. doi:10.1016/j.psyneuen.2016.07.210
- Handa, R.J., & Weiser, M.J. (2014). Gonadal steroid hormones and the hypothalamo-pituitary-adrenal axis. Frontiers in Neuroendocrinology, 35, 197–220. doi:10.1016/j.yfrne.2013.11.001
- Hapgood, J.P., Avenant, C., & Moliki, J.M. (2016). Glucocorticoid-independent modulation of GR activity: Implications for immunotherapy. Pharmacology & Therapeutics, 165, 93–113. doi:10.1016/j.pharmthera.2016.06.002
- Herman, J.P., McKlveen, J.M., Solomon, M.B., Carvalho-Netto, E., & Myers, B. (2012). Neural regulation of the stress response: Glucocorticoid feedback mechanisms. Brazilian Journal of Medical and Biological Research, 45, 292–298. doi:10.1590/S0100-879X2012007500041
- Iwasaki-Sekino, A., Mano-Otagiri, A., Ohata, H., Yamauchi, N., & Shibasaki, T. (2009). Gender differences in corticotropin and corticosterone secretion and corticotropin-releasing factor mRNA expression in the paraventricular nucleus of the hypothalamus and the central nucleus of the amygdala in response to footshock stress or psychological stress in rats. Psychoneuroendocrinology, 34, 226–237. doi:10.1016/j.psyneuen.2008.09.003
- Juster, R.P., McEwen, B.S., & Lupien, S.J. (2010). Allostatic load biomarkers of chronic stress and impact on health and cognition. Neuroscience and Biobehavioral Reviews, 35, 2–16. doi:10.1016/j.neubiorev.2009.10.002
- Kalil, B., Leite, C.M., Carvalho-Lima, M., & Anselmo-Franci, J.A. (2013). Role of sex steroids in progesterone and corticosterone response to acute restraint stress in rats: Sex differences. Stress, 16, 452–460. doi:10.3109/10253890.2013.777832
- Kitay, J.I. (1961). Sex differences in adrenal cortical secretion in the rat. Endocrinology, 68, 818–824. doi:10.1210/endo-68-5-818
- Kitay, J.I. (1963). Pituitary-adrenal function in the rat after gonadectomy and gonadal hormone replacement. Endocrinology, 73, 253–260. doi:10.1210/endo-73-2-253
- Larkin, J.W., Binks, S.L., Li, Y., & Selvage, D. (2010). The role of oestradiol in sexually dimorphic hypothalamic-pituitary-adrena axis responses to intracerebroventricular ethanol administration in the rat. Journal of Neuroendocrinology, 22, 24–32. doi:10.1111/j.1365-2826.2009.01934.x
- Lauber, A.H., Mobbs, C.V., Muramatsu, M., & Pfaff, D.W. (1991). Estrogen receptor messenger RNA expression in rat hypothalamus as a function of genetic sex and estrogen dose. Endocrinology, 129, 3180–3186. doi:10.1210/endo-129-6-3180
- Leśniewska, B., Miśkowiak, B., Nowak, M., & Malendowicz, L.K. (1990). Sex differences in adrenocortical structure and function. XXVII. The effect of ether stress on ACTH and corticosterone in intact, gonadectomized, and testosterone- or estradiol-replaced rats. Research in Experimental Medicine, 190, 95–103. doi:10.1007/PL00020011
- Liu, J., Bisschop, P.H., Eggels, L., Foppen, E., Fliers, E., Zhou, J.N., & Kalsbeek, A. (2012). Intrahypothalamic estradiol modulates hypothalamus-pituitary-adrenal-axis activity in female rats. Endocrinology, 153, 3337–3344. doi:10.1210/en.2011-2176
- Lunga, P., & Herbert, J. (2004). 17Beta-oestradiol modulates glucocorticoid, neural and behavioural adaptations to repeated restraint stress in female rats. Journal of Neuroendocrinology, 16, 776–785. doi:10.1111/j.1365-2826.2004.01234.x
- Malviya, S.A., Kelly, S.D., Greenlee, M.M., Eaton, D.C., Duke, B.J., Bourke, C.H., & Neigh, G.N. (2013). Estradiol stimulates an anti-translocation expression pattern of glucocorticoid co-regulators in a hippocampal cell model. Physiology & Behavior, 122, 187–192. doi:10.1016/j.physbeh.2013.03.018
- McCormick, C.M., & Green, M.R. (2013). From the stressed adolescent to the anxious and depressed adult: investigations in rodent models. Neuroscience, 249, 242–257. doi:10.1016/j.neuroscience.2012.08.063
- McCormick, C.M., Green, M.R., Cameron, N.M., Nixon, F., Levy, M.J., & Clark, R.A. (2013). Deficits in male sexual behavior in adulthood after social instability stress in adolescence in rats. Hormones and Behavior, 63, 5–12. doi:10.1016/j.yhbeh.2012.11.009
- McCormick, C.M., Linkroum, W., Sallinen, B.J., & Miller, N.W. (2002). Peripheral and central sex steroids have differential effects on the HPA axis of male and female rats. Stress, 5, 235–247. doi:10.1080/1025389021000061165
- McCormick, C.M., & Singh, P. (1996). Asymmetry in progestin receptor levels and sexual behavior in female rats. Physiology & Behavior, 59, 349–354. doi:10.1016/0031-9384(95)02100-0
- Miller, W.J., Suzuki, S., Miller, L.K., Handa, R., & Uht, R.M. (2004). Estrogen receptor (ER)beta isoforms rather than ERalpha regulate corticotropin-releasing hormone promoter activity through an alternate pathway. Journal of Neuroscience, 24, 10628–10635. doi:10.1523/JNEUROSCI.5540-03.2004
- Mitev, Y.A., Wolf, S.S., Almeida, O.F., & Patchev, V.K. (2003). Developmental expression profiles and distinct regional estrogen responsiveness suggest a novel role for the steroid receptor coactivator SRC-1 as discriminative amplifier of estrogen signaling in the rat brain. The FASEB Journal, 17, 518–519. doi:10.1096/fj.02-0513fje
- Munabi, A.K., Cassorla, F.G., Pfeiffer, D.G., Albertson, B.D., & Loriaux, D.L. (1983). The effects of estradiol and progesterone on rat ovarian 17-hydroxylase and 3 beta-hydroxysteroid dehydrogenase activities. Steroids, 41, 95–98. doi:10.1016/0039-128X(83)90019-3
- Osterlund, M., Kuiper, G.G., Gustafsson, J.A., & Hurd, Y.L. (1998). Differential distribution and regulation of estrogen receptor-alpha and -beta mRNA within the female rat brain. Brain Research. Molecular Brain Research, 54, 175–180. doi:10.1016/S0169-328X(97)00351-3
- Patchev, V.K., & Almeida, O.F. (1996). Gonadal steroids exert facilitating and “buffering” effects on glucocorticoid-mediated transcriptional regulation of corticotropin-releasing hormone and corticosteroid receptor genes in rat brain. Journal of Neuroscience, 16, 7077–7084.
- Peiffer, A., Lapointe, B., & Barden, N. (1991). Hormonal-regulation of type II glucocorticoid receptor messenger ribonucleic acid in rat brain. Endocrinology, 129, 2166–2174. doi:10.1210/endo-129-4-2166
- Ratajczak, T., Ward, B.K., Cluning, C., & Allan, R.K. (2009). Cyclophilin 40: An Hsp90-cochaperone associated with apo-steroid receptors. The International Journal of Biochemistry & Cell Biology, 41, 1652–1655. doi:10.1016/j.biocel.2009.03.006
- Redei, E., Li, L., Halasz, I., McGivern, R.F., & Aird, F. (1994). Fast glucocorticoid feedback inhibition of ACTH secretion in the ovariectomized rat: Effect of chronic estrogen and progesterone. Neuroendocrinology, 60, 113–123. doi:10.1159/000126741
- Reith, M. E. A.,& Neidle, A., ( (1981). Breakdown and fate of ACTH and MSH. Pharmacology & Therapeutics, 12, 449–461. doi:10.1016/0163-7258(81)90092-9
- Rivier, C. (1999). Gender, sex steroids, corticotropin-releasing factor, nitric oxide, and the HPA response to stress. Pharmacology, Biochemistry and Behavior, 64, 739–751. doi:10.1016/S0091-3057(99)00148-3
- Romano, G.J., Krust, A., & Pfaff, D.W. (1989). Expression and estrogen regulation of progesterone receptor mRNA in neurons of the mediobasal hypothalamus: an in situ hybridization study. Molecular Endocrinology, 3, 1295–1300. doi:10.1210/mend-3-8-1295
- Romeo, R.D., Lee, S.J., & McEwen, B.S. (2004). Differential stress reactivity in intact and ovariectomized prepubertal and adult female rats. Neuroendocrinology, 80, 387–393. doi:10.1159/000084203
- Sapolsky, R.M., Romero, L.M., & Munck, A.U. (2000). How do glucocorticoids influence stress responses? Integrating permissive, suppressive, stimulatory, and preparative actions. Endocrine Reviews, 21, 55–89. doi:10.1210/edrv.21.1.0389
- Scharf, S.H., Liebl, C., Binder, E.B., Schmidt, M.V., & Müller, M.B. (2011). Expression and regulation of the Fkbp5 gene in the adult mouse brain. PLoS One, 6, e16883. doi:10.1371/journal.pone.0016883
- Seale, J.V., Wood, S.A., Atkinson, H.C., Bate, E., Lightman, S.L., Ingram, C.D., … Harbuz, M.S. (2004). Gonadectomy reverses the sexually diergic patterns of circadian and stress-induced hypothalamic-pituitary-adrenal axis activity in male and female rats. Journal of Neuroendocrinology, 16, 516–524. doi:10.1111/j.1365-2826.2004.01195.x
- Seale, J.V., Wood, S.A., Atkinson, H.C., Harbuz, M.S., & Lightman, S.L. (2004). Gonadal steroid replacement reverses gonadectomy-induced changes in the corticosterone pulse profile and stress-induced hypothalamic-pituitary-adrenal axis activity of male and female rats. Journal of Neuroendocrinology, 16, 989–998. doi:10.1111/j.1365-2826.2004.01258.x
- Serova, L.I., Harris, H.A., Maharjan, S., & Sabban, E.L. (2010). Modulation of responses to stress by estradiol benzoate and selective estrogen receptor agonists. Journal of Endocrinology, 205, 253–262. doi:10.1677/JOE-10-0029
- Sheng, Z., Yanai, A., Fujinaga, R., Kawano, J., Tanaka, M., Watanabe, Y., & Shinoda, K. (2003). Gonadal and adrenal effects on the glucocorticoid receptor in the rat hippocampus, with special reference to regulation by estrogen from an immunohistochemical view-point. Journal of Neuroscience Research, 46, 205–218. doi:10.1016/S0168-0102(03)00056-7
- Simone, J.J., & McCormick, C.M. (2017). Intracellular signalling and plasma hormone profiles associated with the expression of unconditioned and conditioned fear and anxiety in female rats. Physiology & Behavior, 169, 234–244. doi:10.1016/j.physbeh.2016.12.002
- Turner, B.B. (1990). Sex difference in glucocorticoid binding in rat pituitary is estrogen dependent. Life Sciences, 46, 1399–1406. doi:10.1016/0024-3205(90)90340-W
- Turner, B.B., & Weaver, D.A. (1985). Sexual dimorphism of glucocorticoid binding in rat brain. Brain Research, 343, 16–23. doi:10.1016/0006-8993(85)91153-9
- Viau, V., Bingham, B., Davis, J., Lee, P., & Wong, M. (2005). Gender and puberty interact on the stress-induced activation of parvocellular neurosecretory neurons and corticotropin-releasing hormone messenger ribonucleic acid expression in the rat. Endocrinology, 146, 137–146. doi:10.1210/en.2004-0846
- Viau, V., & Meaney, M.J. (1991). Variations in the hypothalamic-pituitary-adrenal response to stress during the estrous cycle in the rat. Endocrinology, 129, 2503–2511. doi:10.1210/endo-129-5-2503
- Weinstock, M., Razin, M., Schorer-Apelbaum, D., Men, D., & McCarty, R. (1998). Gender differences in sympathoadrenal activity in rats at rest and in response to footshock stress. International Journal of Developmental Neuroscience, 16, 289–295. doi:10.1016/S0736-5748(98)00021-5
- Weiser, M.J., & Handa, R.J. (2009). Estrogen impairs glucocorticoid dependent negative feedback on the hypothalamic-pituitary-adrenal axis via estrogen receptor alpha within the hypothalamus. Neuroscience, 159, 883–895. doi:10.1016/j.neuroscience.2008.12.058
- Wochnik, G.M., Ruegg, J., Abel, G.A., Schmidt, U., Holsboer, F., & Rein, T. (2005). FK506-binding proteins 51 and 52 differentially regulate dynein interaction and nuclear translocation of the glucocorticoid receptor in mammalian cells. Journal of Biological Chemistry, 280, 4609–4616. doi:10.1074/jbc.M407498200
- Young, E.A. (1996). Sex differences in response to exogenous corticosterone: a rat model of hypercortisolemia. Molecular Psychiatry, 1, 313–319.
- Young, E.A., Altemus, M., Parkinson, V., & Shastry, S. (2001). Effects of estrogen antagonists and agonists on the ACTH response to restraint stress in female rats. Neuropsychopharmacology: Official Publication of the American College of Neuropsychopharmacology, 25, 881–891. doi:10.1016/S0893-133X(01)00301-3
- Zhang, D., Guo, Q., Bian, C., Zhang, J., Cai, W., & Su, B. (2011). Expression of steroid receptor coactivator-1 was regulated by postnatal development but not ovariectomy in the hippocampus of rats. Developmental Neuroscience, 33, 57–63. doi:10.1159/000322978
- Zhang, Y., Leung, D.Y., Nordeen, S.K., & Goleva, E. (2009). Estrogen inhibits glucocorticoid action via protein phosphatase 5 (PP5)-mediated glucocorticoid receptor dephosphorylation. Journal of Biological Chemistry, 284, 24542–24552. doi:10.1074/jbc.M109.021469