Abstract
Cholestasis is a multifaceted clinical complication. Obstructive jaundice induced by bile duct ligation (BDL) is known as an animal model to investigate cholestasis and its associated complications. N-acetyl cysteine (NAC) is an antioxidant, radical scavenger, and thiol reductant widely investigated for its cytoprotective properties. The current investigation was designed to evaluate the role of NAC treatment on biomarkers of oxidative stress and organ histopathological alterations in a rat model of cholestasis/cirrhosis. BDL animals were supplemented with NAC (100 and 300 mg/kg, i.p, 42 consecutive days). Biomarkers of oxidative stress in the liver, brain, heart, skeletal muscle, lung, serum, and kidney tissue, as well as organ histopathological changes, were monitored. A significant increase in reactive oxygen species, lipid peroxidation, and protein carbonylation were detected in different tissues of BDL rats. Moreover, tissue antioxidant capacity was hampered, glutathione (GSH) reservoirs were depleted, and oxidized glutathione (GSSG) levels were significantly increased in the BDL group. Significant tissue histopathological alterations were evident in cirrhotic animals. It was found that NAC treatment (100 and 300 mg/kg, i.p) significantly mitigated biomarkers of oxidative stress and alleviated tissue histopathological changes in cirrhotic rats. These data represent NAC as a potential protective agent with therapeutic capability in cirrhosis and its associated complications.
Cholestasis is a multifaceted clinical complication that affects different organs
Oxidative stress plays a pivotal role in cholestasis-associated complications
Tissue antioxidant capacity is hampered in different tissues of cholestatic animals
Antioxidant therapy might play a role in the management of cholestasis-induced organ injury
NAC alleviated biomarkers of oxidative stress in cholestatic animals
NAC significantly improved tissues histopathological alterations in cholestatic rats
HIGHLIGHTS
1. Introduction
Cholestasis could result from a variety of etiologies. Pregnancy, genetic disorders, gall stones, autoimmune disease, malignancies, as well as a wide range of xenobiotics could result in a decrease in bile flow and cholestasis (Hirschfield et al., Citation2010; Jüngst & Lammert, Citation2013; Levy, Citation2013; Poli, Citation2000; Poupon, Citation2010). Different experimental models have been developed to investigate the effect of cholestasis and its associated complications (Vinken, Citation2019). Bile duct ligation, genetically modified animals, and different in vitro models of cell culture have been used as experimental tools to evaluate cholestasis and its associated complications (Vinken, Citation2019). Different markers, including apoptosis/necrosis mode of cell death, oxidative/nitrosative markers, mitochondrial impairment, as well as histopathological tissue changes, could be assessed in these models of experimental cholestasis (Vinken, Citation2019). Prolonged and untreated cholestasis could lead to severe tissue fibrosis and cirrhosis (Poli, Citation2000). On the other hand, there are limited numbers of pharmaceuticals for the treatment of cholestasis and its associated complications. Surgical intervention is the only effective strategy against approximately all types of cholestasis. Currently, ursodeoxycholic acid (UDCA) is the leading pharmaceutical used against cholestasis (Kumar & Tandon, Citation2001; Paumgartner & Beuers, Citation2002). However, the efficacy of UDCA might be limited in some patients. UDCA may also not affect cholestasis-induced adverse effects in tissues such as skeletal muscle, heart, lung, or brain (Kumar & Tandon, Citation2001; Paumgartner & Beuers, Citation2002). Therefore, finding protective agents against cholestasis-induced organ injury could have clinical value.
The liver is the primary organ affected in cholestasis (Copple et al., Citation2010; Heidari, Niknahad, et al., Citation2018; O’Brien et al., Citation2016; Orellana et al., Citation2000). On the other hand, other tissues rather than the liver could also be affected in cholestasis (Heidari, Niknahad, et al., Citation2018; O’Brien et al., Citation2016; Orellana et al., Citation2000). The adverse effects of cholestasis on the brain, skeletal muscle, heart, lung, and kidneys have been observed in several investigations (Bosoi et al., Citation2017; Heidari, Ghanbarinejad, Mohammadi, et al., Citation2018a; Martínez-Cecilia et al., Citation2016; Shikata et al., Citation2014). It has been well-documented that extrahepatic cholestasis induced by bile duct ligation (BDL) is an appropriate experimental model to investigate cholestasis (Fickert et al., Citation2013; Heidari, Niknahad, et al., Citation2018; Tag, Sauer-Lehnen, et al., Citation2015; Tag, Weiskirchen, et al., Citation2015). Severe liver histopathological changes, skeletal muscle atrophy and muscle mass loss (sarcopenia), cardiac dysfunction, brain damage, and encephalopathy, lung injury, and renal damage (cholemic nephropathy) are appropriately induced in the BDL animal model (Fickert et al., Citation2013; Heidari, Niknahad, et al., Citation2018; Shikata et al., Citation2014; Tag, Sauer-Lehnen, et al., Citation2015; Tag, Weiskirchen, et al., Citation2015). The detrimental effects of cholestasis/cirrhosis on the central nervous system also have been repeatedly documented (Butterworth, Citation2011; Görg et al., Citation2010; Sheen et al., Citation2010). Progressive cognitive impairment and brain injury have been demonstrated in the BDL model of cirrhosis (Heidari et al., Citation2016; Huang et al., Citation2009). Accumulation of ammonia as a neurotoxin is the primary cause of oxidative stress and cognitive deterioration in the experimental model of cirrhosis as well as cirrhotic patients (Romero-Gómez et al., Citation2015). The role of oxidative stress and its associated complications also has been revealed in the pathogenesis of cholestasis/cirrhosis-associated nephropathy (García-Estañ et al., Citation2002; Martínez-Cecilia et al., Citation2016). Some clues also indicate the role of oxidative stress in cholestasis-induced cardiomyocytes injury (Shafaroodi et al., Citation2010). On the other hand, some studies indicate the role of oxidative stress and its associated events in the pathogenesis of cirrhosis-associated skeletal muscle atrophy (Sarcopenia) (Ohara et al., Citation2018). Cholestasis-induced lung injury also is a severe complication associated with a severe inflammatory response in this organ (Shikata et al., Citation2014).
Hydrophobic bile acids are the most suspected molecules responsible for organ injury during bile duct obstruction (Attili et al., Citation1986; Heidari, Ghanbarinejad, Ommati, et al., Citation2018; Palmeira & Rolo, Citation2004; Rolo et al., Citation2003; Woolbright & Jaeschke, Citation2012). Bilirubin is another toxic substance accumulated in the body during cholestasis (Malik et al., Citation2010; Mustafa et al., Citation1969; Silva et al., Citation2001; Vaz et al., Citation2010). The adverse effects of these compounds have been documented in different experimental models (Malik et al., Citation2010; Rolo et al., Citation2003; Silva et al., Citation2001; Sokol et al., Citation2006). The accumulation of cytotoxic molecules such as bile acids and bilirubin is suspected of being responsible for oxidative stress and organ injury in BDL model of cholestasis (Arduini et al., Citation2012; Malik et al., Citation2010; Mormone et al., Citation2011; Vaz et al., Citation2010). Severe elevation in tissue ROS level increased the damage of intracellular targets (e.g. lipids and proteins) (Ommati et al., Citation2017), and defect in cellular antioxidant defense mechanisms are documented in different tissues of cirrhotic animals as well as in human subjects (Arduini et al., Citation2012; Chen et al., Citation2013; Copple et al., Citation2010; Görg et al., Citation2010; Heidari, Ghanbarinejad, Mohammadi, et al., Citation2018b; Heidari et al., Citation2017; Heidari, Niknahad, et al., Citation2018; Ljubuncic et al., Citation2000; Orellana et al., Citation2000). It has been reported that oxidative stress is a general phenomenon in cholestasis/cirrhosis (Görg et al., Citation2010; Huang et al., Citation2009; Ljubuncic et al., Citation2000). BDL-induced oxidative stress affects different organs, even those protected by barriers (e.g. brain) (Görg et al., Citation2010; Huang et al., Citation2009; Ljubuncic et al., Citation2000).
Oxidative stress and its associated consequences are well-characterized events in cholestasis models (Assimakopoulos et al., Citation2007; Chen et al., Citation2013; Copple et al., Citation2010; Heidari, Ghanbarinejad, Mohammadi, et al., Citation2018b; Heidari et al., Citation2017; Heidari, Niknahad, et al., 2018; Holt et al., Citation1999; Jamshidzadeh et al., Citation2017a; Ljubuncic et al., Citation2000; Orellana et al., Citation2000). It has been documented that enzymatic and non-enzymatic antioxidant systems are hampered in the liver tissue of cholestatic/cirrhotic animals or human cases (Ljubuncic et al., Citation2000). However, there is little data about the occurrence of oxidative stress in other tissues. Some studies reported the association between oxidative stress and renal injury in the BDL animal model (Heidari, Ghanbarinejad, Mohammadi, et al., Citation2018a). There is also reports of elevated plasma level of oxidative stress biomarkers in the cirrhotic patients (Šebeková et al., Citation2002). Some studies also reported the importance of oxidative stress in the pathogenesis of brain injury during cholestasis/cirrhosis (Huang et al., Citation2009; Sheen et al., Citation2010).
N-acetyl cysteine (NAC) is a source of sulfhydryl groups and a precursor for glutathione (GSH) (Cotgreave, Citation1996; Lasram et al., Citation2015; Samuni et al., Citation2013). It has also been found that NAC could react with reactive species and acts as a radical scavenger (Lasram et al., Citation2015; Samuni et al., Citation2013). On the other hand, NAC is a thiol-reducing agent and acts as a precursor for the synthesis of vital molecules including GSH (Samuni et al., Citation2013). Several investigations, including clinical trials, mentioned NAC as a safe and clinically applicable therapeutic agent against a wide range of human diseases (Cotgreave, Citation1996; Elbini Dhouib et al., Citation2016; Lasram et al., Citation2015). The protective effects of NAC in diabetes mellitus-associated complications, neurodegenerative diseases, liver injury with different etiologies, ischemia-reperfusion-induced organ injury, acute respiratory distress syndrome, bronchitis, as well as xenobiotics-induced toxicity has been repeatedly mentioned in previous studies (Arakawa & Ito, Citation2007; Cotgreave, Citation1996; Elbini Dhouib et al., Citation2016; Lasram et al., Citation2015; Samuni et al., Citation2013). Oxidative stress-mediated injury is a characteristic feature of approximately all of these complications (Arakawa & Ito, Citation2007; Samuni et al., Citation2013; Sun et al., Citation2014).
The current study was designed to evaluate the effects of NAC supplementation on oxidative stress biomarkers in different tissues of cirrhotic animals. The data could help the development of effective therapeutic strategies in cholestatic/cirrhotic patients.
2. Materials and methods
2.1. Chemicals
4,2-Hydroxyethyl,1-piperazine ethane sulfonic acid (HEPES), 6-hydroxy-2,5,7,8-tetramethyl chroman-2-carboxylic acid (Trolox), fatty acid-free bovine serum albumin (BSA) fraction V, thiobarbituric acid (TBA), glutathione (GSH), methanol (HPLC grade), 2′,7′-Dichlorofluorescein diacetate (DCFH-DA), malondialdehyde (MDA), iodoacetic acid, N-acetylcysteine (NAC), dinitrophenylhydrazine (DNPH), sodium phosphate dibasic (Na2HPO4), fluoro-2,4-dinitrobenzene (FDNB), coomassie brilliant blue, dithiothreitol, acetonitrile (HPLC grade), oxidized glutathione (GSSG), ethylene glycol-bis (2-aminoethyl ether)-N, N, N′, N′-tetraacetic acid (EGTA), and ethylenediaminetetraacetic acid (EDTA) were purchased from Sigma Chemical Co. (St. Louis, MO, USA). Trichloroacetic acids (TCA), potassium chloride (KCl), and hydroxymethyl aminomethane hydrochloride (Tris-HCl) were purchased from Merck (Merck KGaA, 64271, Darmstadt, Germany). All salts for preparing buffer solutions were prepared from Merck (Merck KGaA, 64271, Darmstadt, Germany).
2.2. Animals
Mature male Sprague-Dawley (SD) rats (n = 32, weighing 200–250 g) were purchased from the Comparative and Experimental Medicine Center of Shiraz University of Medical Sciences, Shiraz, Iran. The experimental rat, maintained under controlled light (12:12 h) and temperature (23–25ºC), and 40% relative humidity, had free access to tap water and a standard rodent chow diet (RoyanFeed®, Esfahan, Iran). Rats were handled according to the animal handling procedure approved by the institutional ethics committee (Shiraz University of Medical Sciences, Iran, 1396-01-36-14822).
2.3. Bile duct ligation surgery and experimental setup
Rats underwent bile duct ligation surgery. For this purpose, animals were anesthetized (10 mg/kg of xylazine and 70 mg/kg of ketamine, i.p), a midline incision was made through the linea alba, and the common bile duct was localized, doubly ligated, and cut between two ligatures (Heidari & Niknahad, Citation2019). The sham operation consisted of laparotomy and bile duct identification and manipulation without ligation. Animals were randomly allotted into: (1) Sham-operated; (2) BDL; (3) BDL + NAC 100 mg/kg, i.p; (4) BDL + NAC 300 mg/kg, i.p. Animals received NAC daily for 42 consecutive days.
2.4. Blood biochemistry, tissue histopathology, and organ weight index
Rats were anesthetized by thiopental (80 mg/kg, i.p). Blood samples were collected from the inferior vena cava. Tissue samples were also collected and washed in ice-cooled (4 °C) normal saline for further evaluations. Standard commercial kits and a MindrayBS-200® auto analyzer were used to measure serum aspartate aminotransferase (AST), alanine aminotransferase (ALT), total bilirubin, alkaline phosphatase (ALP), gamma-glutamyl transferase (GGT), creatine kinase (CK-MB), and lactate dehydrogenase (LDH). Blood samples were collected (from the abdominal aorta) and transferred to standard tubes. Serum and tissue bile acids were measured using the EnzyFluo™ Bile Acid Assay Kit (BioAssay Systems, Hayward, CA 94545, USA) based on a fluorometric method. For the assessment of tissue level of bilirubin and bile acids, 1 mL of tissue homogenate (10% w: v in KCl) was digested with 100 µL of trichloroacetic acid (TCA; 50% w: v), centrifuged (12,000 g, 4 °C, 15 min), and the supernatant was used. For histopathological assessments, tissue samples were fixed in a buffered formalin solution (0.64% sodium phosphate dibasic, Na2HPO4, 0.4% sodium phosphate monobasic, NaH2PO4, and 10% v: v of formaldehyde in double distilled water; pH = 7.4). Finally, paraffin-embedded sections (5 µm) of tissues were stained with hematoxylin and eosin (H&E). The Masson trichrome staining was applied to assess fibrotic tissue alterations. Tissue slides were blindly analyzed by a pathologist using a light microscope (Olympus CX21®, Japan). Animals were weighed at the end of the experiment, and the organ weight index was measured as weight index = [Organ weight (g)/Body weight (g)] × 100.
2.5. Reactive oxygen species formation
Reactive oxygen species (ROS) formation was estimated in different tissues of cirrhotic rats, as previously described (Gupta et al., Citation2007). Briefly, tissue samples were homogenized in 5 mL of ice-cooled (4 °C) Tris-HCl buffer (40 mM, pH = 7.4). Samples of the resulted tissue homogenate (100 µL) were mixed with 40 mM Tris-HCl buffer (1 mL; pH = 7.4, 4 °C) and 2′, 7′-dichlorofluorescein diacetate (Final concentration of 10 µM) . The mixture was incubated at 37 °C (15 min, in the dark). Finally, the fluorescence intensity (FI) of the samples was assessed using a FLUOstar Omega® multifunctional microplate reader (BMG, Labtech Inc., Germany) with λexcitation = 485 nm and λemission = 525 nm (Gupta et al., Citation2007; Socci et al., Citation1999).
2.6. Tissue glutathione content
The reduced (GSH) and oxidized (GSSG) glutathione content in different tissues of cirrhotic animals was assessed by the HPLC analysis of deproteinized samples (TCA 50% w: v) after derivatization with iodoacetic acid and fluoro-2,4-dinitrobenzene (Meeks & Harrison, Citation1991). The technique is based on the formation of S-carboxymethyl derivatives of free thiol groups with iodoacetic acid, followed by the change of free amino groups to 2,4-dinitrophenyl derivatives by reaction with fluoro-2,4-dinitrobenzene (FDNB) (Meeks & Harrison, Citation1991). The HPLC system consisted of a 25 cm NH2 column (Bischoff chromatography, Leonberg, Germany), the flow rate 1 mL/min, and a UV detector (λ = 252 nm) (Meeks & Harrison, Citation1991). The mobile phases involved buffer A (Water: Methanol; 1:4 v: v) and buffer B (Acetate buffer: Buffer A; 1:4 v: v). A gradient method with a steady increase of buffer B to 95% in 20 min was used (Meeks & Harrison, Citation1991). GSSG and GSH were used as external standards. Tissue samples (200 mg) were homogenized in Tris-HCl buffer (250 mM; pH = 7.4; 4 °C), and 500 µL of TCA (50% w: v) was added. Samples were incubated for 15 min on ice. Afterward, samples were mixed well and centrifuged (15,000 g, 15 min, 4 °C). Then, the NaOH: NaHCO3 (2 M: 2 M) solution was added (≈300 µL) to 1 mL of the supernatant until the gas production was subsided. Afterward, 100 µL of iodoacetic acid (1.5% w: v in water) was added, and samples were incubated in the dark (1 h, 4 °C). Then, 500 µL of 2, 4-dinitrofluorobenzene (DNFB; 1.5% w: v in absolute ethanol) was added and incubated in the dark (25 °C, at least for 24 h). Finally, samples were centrifuged (15,000 g, 15 min), and 25 µL of the supernatant was injected into the described HPLC system (Meeks & Harrison, Citation1991; Truong et al., Citation2006).
2.7. Lipid peroxidation
The thiobarbituric acid reactive substances (TBARS) were measured as an index of lipid peroxidation (Eftekhari et al., Citation2018; Niknahad et al., Citation2015). The reaction mixture consisted of 1 mL of tissue homogenate (10% w: v in 40 mM Tris-HCl buffer), 1 mL of thiobarbituric acid (0.375%, w: v), and 3 mL of meta-phosphoric acid (1% w: v, pH = 2). Samples were mixed well and heated in a water bath (100 °C; 45 min). Then, the mixture was cooled, and 2 mL of n-butanol was added. Samples were vigorously vortexed and centrifuged (10,000 g, 5 min). Finally, the absorbance of developed color in n-butanol phase (Upper phase) was measured (λ = 532 nm, EPOCH plate reader, BioTek® instrument, Highland Park, USA) (Eftekhari et al., Citation2018; Jamshidzadeh et al., Citation2015).
2.8. Tissue protein carbonylation
The oxidative damage of proteins was assessed by the determination of carbonyl groups based on the reaction with dinitrophenylhydrazine (DNPH) (Heidari et al., Citation2013; Levine et al., Citation1990). Briefly, a 10% tissue homogenate was prepared in phosphate buffer solution (pH = 7.5) containing 0.1% Triton X-100. Tissue homogenate was centrifuged at 700 g, and 500 µL aliquots of the resulting supernatant were administered with 300 µL of 2, DNPH (10 mM, dissolved in 2 M HCl). Samples were then incubated for 1 h in the dark (25 °C, Vortexing every 10 min). Then 100 µL of trichloroacetic acid (20% w: v) was added, and the tubes were centrifuged (12,000 g for 3 min). The supernatant was discarded, and the pellet was washed three times, with 1 mL of ethanol: ethyl acetate (1:1 v: v). The precipitate was re-dissolved in 600 µL of guanidine solution (6 M, with 20 mM potassium phosphate, adjusted to pH = 2.3 with trifluoroacetic acid) for 15 min at 37 °C. Finally, samples were centrifuged (12,000 g for 3 min) and the absorbance was measured at λ = 370 nm (EPOCH plate reader, BioTek® instrument, Highland Park, USA) (Zhang et al., Citation2004).
2.9. Ferric reducing antioxidant power (FRAP) of rat tissue
FRAP assay is a method to measure the formation of Fe2+-tripyridyl-triazine complex (blue colored) from the colorless oxidized Fe3+, which is produced by the action of electron-donating antioxidants (Katalinic et al., Citation2005; Ommati et al., Citation2018). Briefly, the working FRAP reagent was freshly prepared by mixing ten volumes of acetate buffer (300 mmol/L, pH = 3.6), one volume of TPTZ (10 mmol/L in 40 mmol/L HCl) and one volume of ferric chloride (20 mmol/L). Tissue samples were homogenized in an ice-cooled Tris-HCl buffer (40 mM Tris-HCl, pH = 7.4, 4 °C). Then, 100 µL of tissue homogenate was added to 1 mL of the FRAP reagent . The reaction mixture was incubated at 37 °C for 5 min (in the dark). Finally, samples were centrifuged (15,000 g, 4 °C, 1 min) and the absorbance of developed color was measured (λ = 595 nm, EPOCH plate reader, BioTek® instrument, Highland Park, USA) (Alía et al., Citation2003).
2.10. Statistical analysis
Data are given as mean ± SD. The Kolmogorov-Smirnov test was used to assess data normality. The data were normally distributed. Afterward, the comparison of data sets was performed by the one-way analysis of variance (ANOVA) with Tukey’s multiple comparisons as the post hoc test. Histopathological changes scores are given as median and quartiles for eight random pictures per group. The statistical analysis of histopathological scores was performed by the Kruskal–Wallis tests, followed by the Mann Whitney U test. Values of p < 0.05 were considered as statistically significant.
3. Results
Serum biochemical measurements revealed signs of severe liver injury in the BDL rats (). Moreover, a significant increase in the markers of bile ducts damage (ALP and γ-GT), as well as accumulation of bilirubin and bile acids, were evident in the serum of BDL animals (p < 0.001) (). It was found that NAC supplementation (100 and 300 mg/kg) significantly decreased (p < 0.001) serum markers of tissue injury in BDL rats (). Tissue levels of bilirubin (p < 0.001) and bile acids (p < 0.001) were also significantly elevated in the brain, heart, lung, liver, kidney, and skeletal muscle of BDL animals (Supplementary file, Figure 1). NAC administration caused no significant change in tissue or serum level of bilirubin and bile acids (Supplementary file, Figure 1).
Figure 1. Serum biochemical measurements in bile duct ligated (BDL) rats. NAC: N-acetyl cysteine. Data are given as mean ± SD (n = 8). ***Significantly different as compared with the control group (p < 0.001). aSignificantly different as compared with the BDL group (p < 0.001). ns: not significant as compared with the control group.
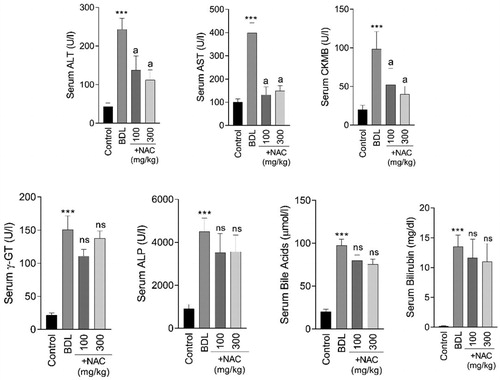
The organ weight index was significantly different for the liver, kidney, spleen, and skeletal muscle (Gastrocnemius) of BDL rats as compared with sham-operated animals (). No significant changes in heart and lung organ weight index was detected between BDL and the control group (). It was found that NAC supplementation significantly affected the alterations in organ weight index of BDL rats ().
Figure 2. Organ weight index in cirrhotic rats. Columns of weight index for each organ indicate sham-operated, BDL, BDL + NAC 100 mg/kg, and BDL + NAC 300 mg/kg respectively (left to right). Data are represented as mean ± SD (n = 8). Asterisks indicate significantly different as compared with the control group (*p < 0.05, **p < 0.01). aIndicates significantly different as compared with the BDL group (p < 0.01). ns: not significant as compared with the BDL group.
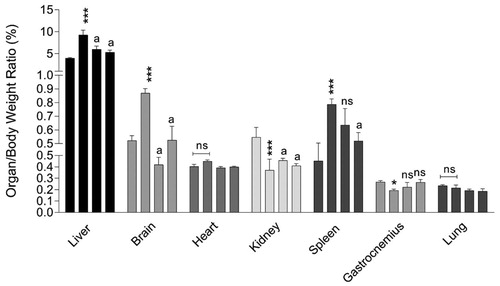
Biomarkers of oxidative stress were significantly increased (p < 0.001) in the brain tissue of BDL animals (). A significant increase in brain ROS level (F = 52.8; df = 3; p < 0.001), lipid peroxidation (F = 201.1; df = 3; p < 0.001), protein carbonylation (F = 8.186, df = 3; p < 0.001), and impaired antioxidant capacity (F = 99.42; df = 3; p < 0.001) was evident in the BDL group (). Moreover, brain tissue GSH content (F = 35.38; df = 3; p < 0.001) was depleted, where the GSSG level (F = 50.49; df = 3; p < 0.001) was significantly increased in BDL rats (). Brain tissue histopathological alterations were also evident as brain edema and inflammation in the BDL group (Supplementary file, Table 1, and ). It was found that NAC supplementation (100 and 300 mg/kg) decreased biomarkers of oxidative stress in the brain of BDL rats (). Brain histopathological alterations were also mitigated upon NAC supplementation (Supplementary file, Table 1, and ).
Figure 3. Markers of oxidative stress in the brain tissue of cirrhotic animals. BDL: Bile duct ligated; NAC: N-acetyl cysteine. Data are represented as mean ± SD (n = 8). ***Significantly different as compared with the control group (p < 0.001). aSignificantly different as compared with the BDL group (p < 0.001). ns: not significant as compared with the BDL group.
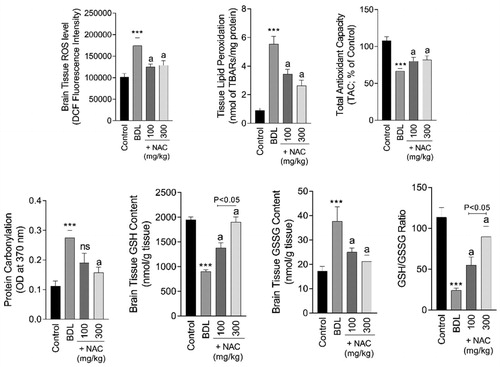
The most significant histopathological alteration in the heart tissue of BDL animals was evident as inflammation (Supplementary file, Table 1, and ). No sign of heart muscle remodeling and myopathy was detected in the current study. On the other hand, biomarkers of oxidative stress, including ROS formation (F = 64.93; df = 3; p < 0.001), lipid peroxidation (F = 51.68; df = 3; p < 0.001), protein carbonylation (F = 39.75; df = 3; p < 0.001), defected tissue antioxidant capacity (F = 193; df = 3; p < 0.001), depleted GSH reservoirs (F = 35.25, df = 3; p < 0.001), and increased GSSG content (F = 42.34; df = 3; p < 0.001) was evident in the heart tissue of BDL animals (). It was found that NAC treatment (100 and 300 mg/kg) significantly decreased oxidative stress biomarkers (), as well as tissue histopathological changes (Supplementary file Table 1, and ) in the heart of BDL rats.
Figure 4. Effect of N-acetyl cysteine (NAC) supplementation on the cirrhosis-associated heart tissue injury and oxidative stress. BDL: Bile duct ligated. Data are represented as mean ± SD (n = 8). ***Indicates significantly different as compared with the control group (p < 0.001). aIndicates significantly different as compared with the BDL group (p < 0.001).
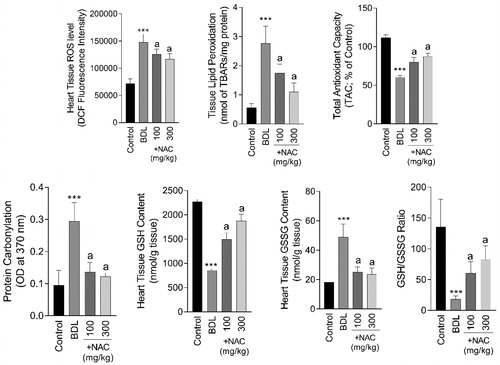
Liver tissue biomarkers of oxidative stress including ROS formation (F = 75.53; df = 3; p < 0.001), lipid peroxidation (F = 82.3; df = 3; p < 0.001), protein carbonylation (F = 13.54; df = 3; p < 0.001), defected tissue antioxidant capacity (F = 31.62; df = 3; p < 0.001), depleted GSH reservoirs (F = 148.1, df = 3; p < 0.001), and increased GSSG content (F = 217.2; df = 3; p < 0.001) were significantly increased in the liver of BDL animals (). On the other hand, NAC treatment (100 and 300 mg/kg) significantly decreased markers of oxidative stress in BDL animals (). Severe tissue histopathological changes, including bile duct proliferation, inflammatory cell infiltration, and tissue necrosis, were evident in the liver of BDL rats (Supplementary file, Table 1 and ). It was found that NAC supplementation (100 and 300 mg/kg) mitigated liver tissue histopathological alterations in BDL animals (Supplementary file, Table 1 and ).
Figure 5. N-acetyl cysteine (NAC) supplementation mitigates biomarkers of oxidative stress in the liver of bile duct ligated (BDL) rats. Data are represented as mean ± SD (n = 8). ***Significantly different as compared with the control group (p < 0.001). aSignificantly different as compared with the BDL group (p < 0.001).
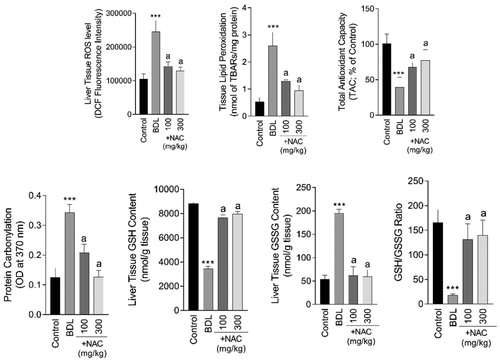
A significant increase in biomarkers of oxidative stress involved ROS formation (F = 53.41; df = 3; p < 0.001), lipid peroxidation (F = 992.1; df = 3; p < 0.001), protein carbonylation (F = 114.9; df = 3; p < 0.001), defected tissue antioxidant capacity (F = 74.69; df = 3; p < 0.001), depleted GSH reservoirs (F = 239.3, df = 3; p < 0.001), and increased GSSG content (F = 43.57; df = 3; p < 0.001) were evident in the renal tissue of BDL rats (). NAC treatment significantly decreased BDL-induced oxidative stress in the kidney tissue (). Severe histopathological alterations were also detected in the kidney of BDL animals (Supplementary file, Table 1, and ). Bile cast formation, kidney tissue necrosis, and interstitial inflammation were found in BDL rats (Supplementary file, Table 1 and ). It was found that NAC administration (100 and 300 mg/kg) ameliorated the detrimental effects of bile duct obstruction in the rat kidney (Supplementary file, Table 1 and ).
Figure 6. Effect of N-acetyl cysteine (NAC) on kidney tissue markers of oxidative stress in cirrhotic animals. BDL: Bile duct ligated. Data are represented as mean ± SD (n = 8). ***Indicates significantly different as compared with the control group (p < 0.001). aIndicates significantly different as compared with the BDL group (p < 0.001).
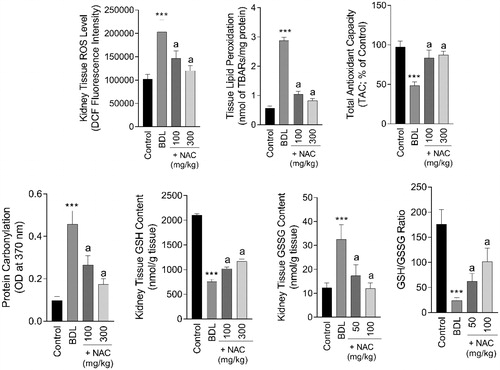
Biomarkers of oxidative stress were significantly increased in the skeletal muscle (Gastrocnemius) of BDL animals (). Significant ROS level (F = 65.19; df = 3; p < 0.001), protein carbonylation (F = 26.94; df = 3; p < 0.001), lipid peroxidation (F = 91.87; df = 3; p < 0.001), a decrease in tissue antioxidant capacity (F = 38.08; df = 3; p < 0.001), and GSH content (F = 95.42; df = 3; p < 0.001), besides increased GSSG level (F = 33.18; df = 3; p < 0.001), was detected in the skeletal muscle of BDL rats (). On the other hand, it was found that the higher dose of NAC (300 mg/kg, i.p) significantly alleviated biomarkers of oxidative stress in the muscle tissue of BDL animals (). Significant muscle atrophy was also detected in the BDL group (Supplementary file, Table 1, and ). No sign of muscle atrophy was evident in NAC-treated BDL animals (Supplementary file, Table 1, and ).
Figure 7. Biomarkers of oxidative stress in cirrhotic rats’ skeletal muscle (Gastrocnemius). NAC: N-acetyl cysteine; BDL: Bile duct ligation. Data are represented as mean ± SD (n = 8). ***Significantly different as compared with the control group (p < 0.001). a,bSignificantly different as compared with the BDL group (p < 0.001 and p < 0.05 respectively). ns: not significant as compared with the BDL group.
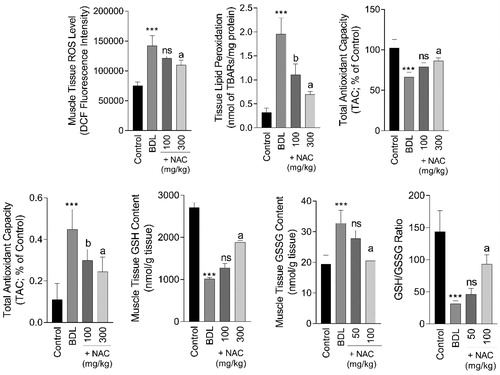
Inflammation, hemorrhage, and mild tissue fibrosis were the signs of histopathological alterations in the lung tissue of BDL rats (Supplementary file, Table 1, and ). On the other hand, lung tissue biomarkers of oxidative stress were significantly increased in BDL animals in comparison with the sham-operated group (). Significant lipid peroxidation (F = 75.53; df = 3; p < 0.001), ROS formation (F = 75.53; df = 3; p < 0.001), protein carbonylation (F = 75.53; df = 3; p < 0.001), and defect in antioxidant defense mechanisms (F = 75.53; df = 3; p < 0.001) were detected in the lung tissue (). It was found that NAC treatment (100 and 300 mg/kg, i.p) alleviated lung tissue histopathological changes (Supplementary file, Table 1 and ) and biomarkers of oxidative stress () in BDL animals.
Figure 8. N-acetyl cysteine (NAC) mitigates lung tissue injury in cirrhotic animals. BDL: Bile duct ligated. Data are represented as mean ± SD (n = 8). ***Significantly different as compared with the control group (p < 0.001). aIndicates significantly different as compared with the BDL group (p < 0.001).
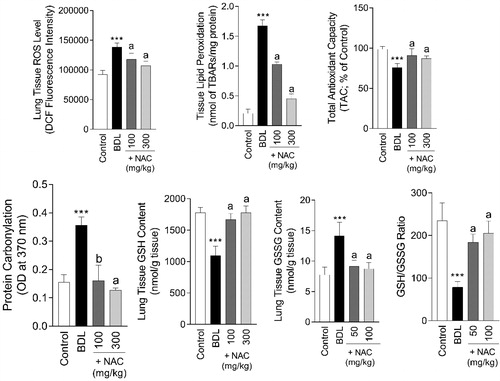
Markers of oxidative stress were significantly changed in the serum of BDL animals (). Significant elevation in protein carbonyls (F = 31.79; df = 3; p < 0.001), lipid peroxidation (F = 143.7; df = 3; p < 0.001), and ROS formation (F = 30.68; df = 3; p < 0.001) was detected in the serum of the BDL group (). Serum antioxidant capacity was also hampered (F = 85.92; df = 3; p < 0.001), and a significant decrease in serum GSH level (F = 21.72; df = 3; p < 0.001) was detected in the cirrhotic rats (). On the other hand, serum GSSG level was significantly increased (F = 22.79; df = 3; p < 0.001) in BDL animals (). It was found that NAC supplementation mitigated oxidative stress in the serum of the BDL group ().
Figure 9. Biomarkers of oxidative stress in the serum of cirrhotic animals. BDL: Bile duct ligated. Data are represented as mean ± SD (n = 8). ***Indicates significantly different as compared with the control group (p < 0.001). aSignificantly different as compared with the BDL group (p < 0.001).
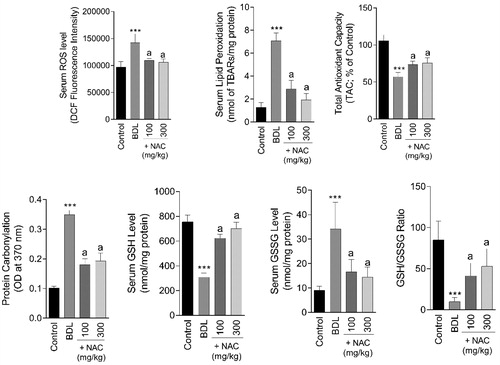
Figure 10. Effect of N-acetylcysteine (NAC) treatment on tissue histopathological changes in bile duct ligated (BDL) animal model of cirrhosis. Significant histopathological alterations were evident in different tissues of BDL animals (Supplementary file, Table 1). It was found that NAC supplementation (100 and 300 mg/kg) provided an ameliorative effect on BDL-associated tissue histopathological changes (Supplementary file, Table 1).
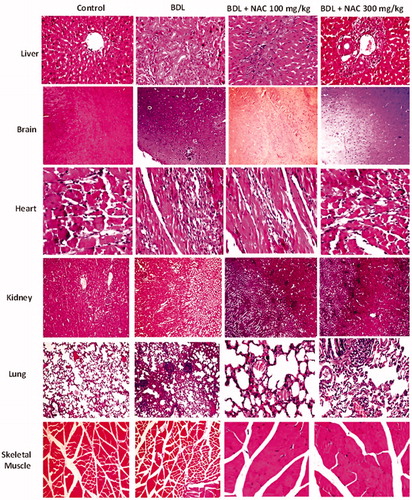
It is noteworthy to mention that the protective properties of NAC were not dose-dependent in the current study. Both 100 and 300 mg/kg doses of NAC provided significant protection in BDL animals.
4. Discussion
Cholestasis is a multifaceted clinical complication. The liver is the primary organ influenced by cholestasis (Copple et al., Citation2010; Li & Chiang, Citation2017; Woolbright & Jaeschke, Citation2012). Other organs, rather than the liver, could also be affected by cholestasis (Bosoi et al., Citation2017; Holt et al., Citation1999; Krones et al., Citation2015).
It has been well-documented that oxidative stress plays a fundamental role in the pathophysiology of cholestasis-associated hepatic injury (Assimakopoulos et al., Citation2007; Heidari, Ghanbarinejad, Mohammadi, et al., Citation2018b; Heidari et al., Citation2017; Orellana et al., Citation2000). Bile acids and bilirubin might be the significant hepatotoxic chemicals accumulated in the liver tissue during cholestasis (Assimakopoulos et al., Citation2007; Heidari, Ghanbarinejad, Mohammadi, et al., Citation2018b; Heidari et al., Citation2017; Ljubuncic et al., Citation2000). A battery of mechanisms has been proposed for cholestasis/cirrhosis-induced organ injury. However, oxidative stress and its related events are the most relevant mechanisms associated with cholestasis/cirrhosis-associated complications (Arduini et al., Citation2012; Chen et al., Citation2013; Copple et al., Citation2010; Heidari, Ghanbarinejad, Mohammadi, et al., Citation2018b; Heidari et al., Citation2017; Heidari, Niknahad, et al., Citation2018; Shafaroodi et al., Citation2010). In line with previous studies, we found that BDL-induced chronic cholestasis was associated with oxidative stress in different organs, as evidenced by ROS formation, increased lipid peroxidation, and low antioxidant enzyme activity.
It has been repeatedly mentioned that the balance of oxidant and antioxidants is interrupted in different tissues of cirrhotic animals (Copple et al., Citation2010; Görg et al., Citation2010). Singh et al. reported significant lipid peroxidation and defected in enzymatic and non-enzymatic antioxidant systems in the liver and serum of BDL rats (Singh et al., Citation1992). Decreased tissue glutathione levels and the impairment in the activity of glutathione-related enzymes are also reported in the animal model of obstructive jaundice (Singh et al., Citation1992; Yang et al., Citation2009). On the other hand, it has been reported that the administration of antioxidant and radical scavenging agents such as boldine (1 and 10 mg/kg, oral, for 28 consecutive days in BDL rats), α-tocopherol (50 mg/kg, i.p, for 10 consecutive days in BDL rats), allopurinol (100 mg/kg, i.p, for 10 consecutive days in BDL rats), dithiothreitol (0.25% and 1% w: v in drinking water, for 14 days in BDL mice), carnosine (250, 500, and 1000 mg/kg, daily, i.p, for 28 consecutive days in BDL rats), proline (0.25% and 0.5% w: v in drinking water for 42 consecutive days in BDL rats), and betaine (10 and 50 mg/kg, i.p, for 28 consecutive days in BDL rats) could serve as a protective option against cholestasis-induced organ injury (Assimakopoulos et al., Citation2007; Heidari, Ghanbarinejad, Mohammadi, et al., Citation2018b; Heidari et al., Citation2017; Heidari et al., Citation2018; Heidari, Niknahad, et al., Citation2018; Jamshidzadeh et al., Citation2017b). The radical scavenging properties and enhancing liver tissue antioxidant mechanisms seem to play a pivotal role in the hepatoprotective effects of these agents in cholestasis (Heidari, Ghanbarinejad, Mohammadi, et al., Citation2018b; Heidari, Niknahad, et al., Citation2018). However, the effect of antioxidants and protective agents has little been investigated on other organs rather than the liver.
In the current study, we found that oxidative stress is the hallmark of organ injury in the liver and other organs damaged during cholestasis/cirrhosis. On the other hand, NAC administration (100 and 300 mg/kg, i.p, for 28 consecutive days) significantly blunted organ injury in BDL animals. The role of NAC in preserving cellular redox environment and counteraction of oxidative stress seems to be the fundamental mechanism for its protective effects in cirrhotic animals. The response of different tissues to therapeutic agents such as NAC could be different due to the amount of ROS formed in each tissue during cholestasis, the nature of tissue antioxidants capacity, as well as the bioavailability of NAC for each tissue (Figure 2, Supplementary file). There is also evidence for the hepatoprotective effects of NAC in cholestatic liver disease (Pastor et al., Citation1997; Tahan et al., Citation2007; Yang et al., Citation2008). It has been reported that NAC supplementation decreased biomarkers of oxidative stress in the liver tissue of cholestatic animals (Ommati et al., Citation2017; Pastor et al., Citation1997; Tahan et al., Citation2007). The data provided form the current study confirms the antioxidative stress properties of NAC not only in the liver but also in other tissues during bile duct obstruction. Due to the dysregulation of glutathione synthesis in cholestasis (especially in the liver) (Yang et al., Citation2009), the administration of NAC could serve as a promising therapeutic option in the management of cholestasis/cirrhosis-associated organ injury.
There are several hypotheses about the source of ROS in the BDL model of cirrhosis. The elevated level of cytotoxic molecules in cirrhotic patients could be the primary cause of oxidative stress. On the other hand, inflammatory cells and cellular mitochondria could be a source of a considerable amount of ROS in different tissues during cholestasis/cirrhosis (Arduini et al., Citation2012; Copple et al., Citation2010; Khansari et al., Citation2009).
Several studies also indicate the fundamental role of the inflammatory response in ROS formation and oxidative stress (especially in the liver) of cholestatic animals (Babior, Citation2000; Khansari et al., Citation2009). Elevation serum and tissue level of lipopolysaccharide (LPS) could serve as a significant pathogenic factor for the inflammation in the BDL animal model of cholestasis (Fouts et al., Citation2012; Soares et al., Citation2010). Inflammatory cells, such as neutrophils, could produce a considerable amount of ROS (Babior, Citation2000; Khansari et al., Citation2009). Inflammatory cells are also a significant source of cytokines (Babior, Citation2000; Khansari et al., Citation2009). Cytokines could initially mediate inflammatory response and later promote tissue fibrosis (Copple et al., Citation2010). Hydrogen peroxide and superoxide anion generated during inflammatory cell activity could be involved in the oxidant response in cholestatic animals (Copple et al., Citation2010). The cholestasis-induced inflammation might also play a role in the pathogenesis of organ injury in other tissues rather than the liver. Severe interstitial nephritis, as a marker of the inflammatory response, has been documented in cholestasis-induced nephropathy (Lino et al., Citation2005; van Slambrouck et al., Citation2013). In the current study, we also found that inflammation was one of the significant histopathological changes in tissues such as the heart, liver, lung, and kidney of bile duct obstructed rats (Supplementary file, Table 1). Recent studies also revealed a severe inflammatory response in the lung tissue of cholestatic animals (Shikata et al., Citation2014). These data could indicate a significant role for the inflammatory response in the pathogenesis of organ injury not only in the liver tissue but also in other organs affected during cholestasis/cirrhosis. The precise mechanism(s) of the inflammatory response in cholestasis-induced organ injury and the effects of protective agents such as NAC on this process warranted further studies.
Cellular mitochondria could also serve as a crucial source of ROS in different tissues affected by cirrhosis (Arduini et al., Citation2012; Copple et al., Citation2010). The adverse effects of cytotoxic molecules such as ammonia, manganese (in the brain of cirrhotic patients), bile acids, and bilirubin on mitochondrial function, cellular energy metabolism, and oxidative stress have repeatedly been investigated (Arduini et al., Citation2012; Copple et al., Citation2010; Heidari, Niknahad, et al., Citation2018; Sokol et al., Citation2006). Oxidative stress and its subsequent events are well-described mechanisms for bile acids cytotoxicity (Arduini et al., Citation2012; Copple et al., Citation2010; Heidari, Ghanbarinejad, Mohammadi, et al., Citation2018a; Holt et al., Citation1999). On the other hand, cytotoxic bile acids are well-known for their adverse effects on cellular mitochondria. Hydrophobic bile acids are robust agents that induce mitochondrial permeability transition (mPT) (Attili et al., Citation1986; Palmeira & Rolo, Citation2004; Rolo et al., Citation2000). Mitochondrial permeabilization and release of cell death mediators have occurred in bile acid-exposed cells (Perez & Briz, Citation2009; Rodrigues et al., Citation1998). Other molecules, such as ammonia and manganese also have detrimental effects on cellular mitochondria (Ahmadi et al., Citation2018; Heidari, Jamshidzadeh, et al., Citation2018; Jamshidzadeh et al., Citation2017a; Niknahad et al., Citation2017; Ommati et al., Citation2019). The positive effects of NAC on cellular mitochondria have been documented in different experimental models (Fries & Kapczinski, Citation2011; Wright et al., Citation2015; Xiao et al., Citation2016). It has been found that NAC could protect cellular mitochondria against toxic insults (Fries & Kapczinski, Citation2011; Marullo et al., Citation2013; Wright et al., Citation2015; Xiao et al., Citation2016). Moreover, NAC administration significantly mitigated mitochondria-mediated cell death (Fries & Kapczinski, Citation2011; Marullo et al., Citation2013; Wright et al., Citation2015; Xiao et al., Citation2016). The effects of NAC on cellular mitochondria could be mediated through inhibition of mitochondria-facilitated ROS formation, prevention of mitochondrial permeabilization, and enhancement of mitochondrial membrane potential (Fries & Kapczinski, Citation2011; Wright et al., Citation2015; Xiao et al., Citation2016). Based on these data, we might be able to hypothesize that cellular mitochondria could also serve as a target for the cytoprotective and antioxidative effects of NAC in cholestatic animals. Obviously, evaluating the effects of NAC on mitochondrial function in different models of cholestasis warranted further investigations to be cleared.
The occurrence of oxidative stress seems to be a multifactorial and complex phenomenon in different tissue of cholestatic/cirrhotic animals. Despite the source of ROS generated in different tissues during cholestasis/cirrhosis, the data obtained from the current study indicate a significant therapeutic potential for NAC not only in the liver but also in other tissues. Generally, the cytoprotective properties of NAC are believed to be attributed to its ability to function as a GSH precursor (Samuni et al., Citation2013). However, the mechanisms underlying the cytoprotective properties of NAC are complex (Samuni et al., Citation2013). The GSH independent NAC biological activities could also be involved in its protective effects (Samuni et al., Citation2013). Regulation of cell cycle and apoptosis, gene expression and signal transduction, immune system modulation, and the effects of NAC on mitochondrial function all could be involved in its mechanisms of cytoprotection (Samuni et al., Citation2013). NAC is approved as the gold-standard treatment of acetaminophen poisoning (Heard, Citation2008). On the other hand, NAC is now emerging as a therapeutic agent against a wide range of human diseases such as neurodegenerative diseases, liver injury with different etiologies, ischemia-reperfusion-induced organ injury, acute respiratory distress syndrome, bronchitis, as well as xenobiotics-induced toxicity (Cotgreave, Citation1996; Lasram et al., Citation2015; Mokhtari et al., Citation2017; Samuni et al., Citation2013). These data could indicate the importance of NAC as a therapeutic option in clinical settings. Based on the data obtained from the current study, NAC could be considered as a potential protective agent in cirrhosis and its associated adverse effects.
The response of each tissue to oxidative stress is different during BDL (Supplementary file, Figure 2). Higher levels of ROS were formed in the liver, kidney, and brain tissue in our BDL model (Supplementary file, Figure 2). Hence, the protective effects of NAC could be different in each organ. Collectively, our data suggest that NAC supplementation positively affects different tissues in cirrhotic animals. These effects are possibly linked to the preservation of cellular redox environment and mitigation of oxidative stress. The direct (radical scavenging) and indirect (thiol reducing agent and GSH precursor) effects of NAC could be involved in its cytoprotective properties. Future studies are warranted to confirm the clinical significance of these data and the application of NAC as a therapeutic option in cholestatic/cirrhotic patients.
Supplementary_Data.docx
Download MS Word (64.2 KB)Disclosure statement
No potential conflict of interest was reported by the author(s).
Additional information
Funding
References
- Ahmadi, N., Ghanbarinejad, V., Ommati, M. M., Jamshidzadeh, A., & Heidari, R. (2018). Taurine prevents mitochondrial membrane permeabilization and swelling upon interaction with manganese: Implication in the treatment of cirrhosis-associated central nervous system complications. Journal of Biochemical and Molecular Toxicology, 32(11), e22216. https://doi.org/10.1002/jbt.22216
- Alía, M., Horcajo, C., Bravo, L., & Goya, L. (2003). Effect of grape antioxidant dietary fiber on the total antioxidant capacity and the activity of liver antioxidant enzymes in rats. Nutrition Research, 23(9), 1251–1267. https://doi.org/10.1016/S0271-5317(03)00131-3
- Arakawa, M., & Ito, Y. (2007). N-acetylcysteine and neurodegenerative diseases: Basic and clinical pharmacology. Cerebellum (London, England), 6(4), 308–314. https://doi.org/10.1080/14734220601142878
- Arduini, A., Serviddio, G., Tormos, A. M., Monsalve, M., & Sastre, J. (2012). Mitochondrial dysfunction in cholestatic liver diseases. Frontiers in bioscience (Elite Edition), 4, 2233–2252. https://doi.org/10.2741/539
- Assimakopoulos, S. F., Maroulis, I., Patsoukis, N., Vagenas, K., Scopa, C. D., Georgiou, C. D., & Vagianos, C. E. (2007). Effect of antioxidant treatments on the gut-liver axis oxidative status and function in bile duct-ligated rats. World Journal of Surgery, 31(10), 2023–2032. https://doi.org/10.1007/s00268-007-9191-3
- Attili, A. F., Angelico, M., Cantafora, A., Alvaro, D., & Capocaccia, L. (1986). Bile acid-induced liver toxicity: Relation to the hydrophobic-hydrophilic balance of bile acids. Medical Hypotheses, 19(1), 57–69. https://doi.org/10.1016/0306-9877(86)90137-4
- Babior, B. M. (2000). Phagocytes and oxidative stress. The American Journal of Medicine, 109(1), 33–44. https://doi.org/10.1016/S0002-9343(00)00481-2
- Bosoi, C. R., Oliveira, M. M., Ochoa-Sanchez, R., Tremblay, M., Ten Have, G. A., Deutz, N. E., Rose, C. F., & Bemeur, C. (2017). The bile duct ligated rat: A relevant model to study muscle mass loss in cirrhosis. Metabolic Brain Disease, 32(2), 513–518. https://doi.org/10.1007/s11011-016-9937-4
- Butterworth, R. F. (2011). Hepatic encephalopathy: a central neuroinflammatory disorder? Hepatology (Baltimore, Md.), 53(4), 1372–1376. https://doi.org/10.1002/hep.24228
- Chen, C.-C., Ho, C.-Y., Chaung, H.-C., Tain, Y.-L., Hsieh, C.-S., Kuo, F.-Y., Yang, C.-Y., & Huang, L.-T. (2013). Fish omega-3 fatty acids induce liver fibrosis in the treatment of bile duct-ligated rats. Digestive Diseases and Sciences, 58(2), 440–447. https://doi.org/10.1007/s10620-012-2489-5
- Copple, B. L., Jaeschke, H., & Klaassen, C. D. (2010). Oxidative stress and the pathogenesis of cholestasis. Seminars in Liver Disease, 30(2), 195–204. https://doi.org/10.1055/s-0030-1253228
- Cotgreave, I. A. (1996). N-acetylcystei ne: Pharmacological considerations and experimental and clinical applications. In H. Sies (Ed.), Advances in pharmacology (Vol. 38, pp. 205–227). Academic Press.
- Eftekhari, A., Ahmadian, E., Panahi-Azar, V., Hosseini, H., Tabibiazar, M., & Dizaj, S. M. (2018). Hepatoprotective and free radical scavenging actions of quercetin nanoparticles on aflatoxin B1-induced liver damage: In vitro/in vivo studies. Artificial Cells, Nanomedicine, and Biotechnology, 46(2), 411–420. https://doi.org/10.1080/21691401.2017.1315427
- Elbini Dhouib, I., Jallouli, M., Annabi, A., Gharbi, N., Elfazaa, S., & Lasram, M. M. (2016). A minireview on N-acetylcysteine: An old drug with new approaches. Life Sciences, 151, 359–363. https://doi.org/10.1016/j.lfs.2016.03.003
- Fickert, P., Krones, E., Pollheimer, M. J., Thueringer, A., Moustafa, T., Silbert, D., Halilbasic, E., Yang, M., Jaeschke, H., Stokman, G., Wells, R. G., Eller, K., Rosenkranz, A. R., Eggertsen, G., Wagner, C. A., Langner, C., Denk, H., & Trauner, M. (2013). Bile acids trigger cholemic nephropathy in common bile-duct-ligated mice. Hepatology (Baltimore, Md.), 58(6), 2056–2069. https://doi.org/10.1002/hep.26599
- Fouts, D. E., Torralba, M., Nelson, K. E., Brenner, D. A., & Schnabl, B. (2012). Bacterial translocation and changes in the intestinal microbiome in mouse models of liver disease. Journal of Hepatology, 56(6), 1283–1292. https://doi.org/10.1016/j.jhep.2012.01.019
- Fries, G. R., & Kapczinski, F. (2011). N-acetylcysteine as a mitochondrial enhancer: a new class of psychoactive drugs? Revista brasileira de psiquiatria (Sao Paulo, Brazil : 1999), 33(4), 321–322. https://doi.org/10.1590/S1516-44462011000400003
- García-Estañ, J., Ortiz, M. C., & Lee, S. S. (2002). Nitric oxide and renal and cardiac dysfunction in cirrhosis. Clinical Science (London, England : 1979), 102(2), 213–222. https://doi.org/10.1042/cs1020213
- Görg, B., Qvartskhava, N., Bidmon, H.-J., Palomero-Gallagher, N., Kircheis, G., Zilles, K., & Häussinger, D. (2010). Oxidative stress markers in the brain of patients with cirrhosis and hepatic encephalopathy. Hepatology (Baltimore, Md.), 52(1), 256–265. https://doi.org/10.1002/hep.23656
- Gupta, R., Dubey, D. K., Kannan, G. M., & Flora, S. J. S. (2007). Concomitant administration of Moringa oleifera seed powder in the remediation of arsenic-induced oxidative stress in mouse. Cell Biology International, 31(1), 44–56. https://doi.org/10.1016/j.cellbi.2006.09.007
- Heard, K. J. (2008). Acetylcysteine for acetaminophen poisoning. The New England Journal of Medicine, 359(3), 285–292. https://doi.org/10.1056/NEJMct0708278
- Heidari, R., & Niknahad, H. (2019). The role and study of mitochondrial impairment and oxidative stress in cholestasis. In M. Vinken (Ed.), Experimental cholestasis research (pp. 117–132). Springer New York.
- Heidari, R., Babaei, H., & Eghbal, M. A. (2013). Cytoprotective effects of organosulfur compounds against methimazole induced toxicity in isolated rat hepatocytes. Advanced Pharmaceutical Bulletin, 3(1), 135–142. https://doi.org/10.5681/apb.2013.023
- Heidari, R., Ghanbarinejad, V., Mohammadi, H., Ahmadi, A., Esfandiari, A., Azarpira, N., & Niknahad, H. (2018a). Dithiothreitol supplementation mitigates hepatic and renal injury in bile duct ligated mice: Potential application in the treatment of cholestasis-associated complications. Biomedicine & Pharmacotherapy = Biomedecine & Pharmacotherapie, 99, 1022–1032. https://doi.org/10.1016/j.biopha.2018.01.018
- Heidari, R., Ghanbarinejad, V., Mohammadi, H., Ahmadi, A., Esfandiari, A., Azarpira, N., & Niknahad, H. (2018b). Dithiothreitol supplementation mitigates hepatic and renal injury in bile duct ligated mice: Potential application in the treatment of cholestasis-associated complications. Biomedicine and Pharmacotherapy, 99, 1022–1032. https://doi.org/10.1016/j.biopha.2018.01.018
- Heidari, R., Ghanbarinejad, V., Ommati, M. M., Jamshidzadeh, A., & Niknahad, H. (2018). Mitochondria protecting amino acids: Application against a wide range of mitochondria-linked complications. PharmaNutrition, 6(4), 180–190. https://doi.org/10.1016/j.phanu.2018.09.001
- Heidari, R., Jamshidzadeh, A., Ghanbarinejad, V., Ommati, M. M., & Niknahad, H. (2018). Taurine supplementation abates cirrhosis-associated locomotor dysfunction. Clinical and Experimental Hepatology, 4(2), 72–82. https://doi.org/10.5114/ceh.2018.75956
- Heidari, R., Jamshidzadeh, A., Niknahad, H., Mardani, E., Ommati, M. M., Azarpira, N., Khodaei, F., Zarei, A., Ayarzadeh, M., Mousavi, S., Abdoli, N., Yeganeh, B. S., Saeedi, A., & Najibi, A. (2016). Effect of taurine on chronic and acute liver injury: Focus on blood and brain ammonia. Toxicology Reports, 3, 870–879. https://doi.org/10.1016/j.toxrep.2016.04.002
- Heidari, R., Moezi, L., Asadi, B., Ommati, M. M., & Azarpira, N. (2017). Hepatoprotective effect of boldine in a bile duct ligated rat model of cholestasis/cirrhosis. PharmaNutrition, 5(3), 109–117. https://doi.org/10.1016/j.phanu.2017.07.001
- Heidari, R., Mohammadi, H., Ghanbarinejad, V., Ahmadi, A., Ommati, M. M., Niknahad, H., Jamshidzadeh, A., Azarpira, N., & Abdoli, N. (2018). Proline supplementation mitigates the early stage of liver injury in bile duct ligated rats. Journal of Basic and Clinical Physiology and Pharmacology, 30(1), 91–101. https://doi.org/10.1515/jbcpp-2017-0221
- Heidari, R., Niknahad, H., Sadeghi, A., Mohammadi, H., Ghanbarinejad, V., Ommati, M. M., Hosseini, A., Azarpira, N., Khodaei, F., Farshad, O., Rashidi, E., Siavashpour, A., Najibi, A., Ahmadi, A., & Jamshidzadeh, A. (2018). Betaine treatment protects liver through regulating mitochondrial function and counteracting oxidative stress in acute and chronic animal models of hepatic injury. Biomedicine & Pharmacotherapy = Biomedecine & Pharmacotherapie, 103, 75–86. https://doi.org/10.1016/j.biopha.2018.04.010
- Hirschfield, G. M., Heathcote, E. J., & Gershwin, M. E. (2010). Pathogenesis of cholestatic liver disease and therapeutic approaches. Gastroenterology, 139(5), 1481–1496. https://doi.org/10.1053/j.gastro.2010.09.004
- Holt, S., Marley, R., Fernando, B., Harry, D., Anand, R., Goodier, D., & Moore, K. (1999). Acute cholestasis-induced renal failure: Effects of antioxidants and ligands for the thromboxane A2 receptor. Kidney International, 55(1), 271–277. https://doi.org/10.1046/j.1523-1755.1999.00252.x
- Huang, L.-T., Tiao, M.-M., Tain, Y.-L., Chen, C.-C., & Hsieh, C.-S. (2009). Melatonin ameliorates bile duct ligation-induced systemic oxidative stress and spatial memory deficits in developing Rats. Pediatric Research, 65(2), 176–180. https://doi.org/10.1203/PDR.0b013e31818d5bc7
- Jamshidzadeh, A., Heidari, R., Latifpour, Z., Ommati, M. M., Abdoli, N., Mousavi, S., Azarpira, N., Zarei, A., Zarei, M., Asadi, B., Abasvali, M., Yeganeh, Y., Jafari, F., Saeedi, A., Najibi, A., & Mardani, E. (2017a). Carnosine ameliorates liver fibrosis and hyperammonemia in cirrhotic rats. Clinics and Research in Hepatology and Gastroenterology, 41(4), 424–434. https://doi.org/10.1016/j.clinre.2016.12.010
- Jamshidzadeh, A., Heidari, R., Latifpour, Z., Ommati, M. M., Abdoli, N., Mousavi, S., Azarpira, N., Zarei, A., Zarei, M., Asadi, B., Abasvali, M., Yeganeh, Y., Jafari, F., Saeedi, A., Najibi, A., & Mardani, E. (2017b). Carnosine ameliorates liver fibrosis and hyperammonemia in cirrhotic rats. Clinics and Research in Hepatology and Gastroenterology, 41(4), 424–434. https://doi.org/10.1016/j.clinre.2016.12.010
- Jamshidzadeh, A., Heidari, R., Mohammadi-Samani, S., Azarpira, N., Najbi, A., Jahani, P., & Abdoli, N. (2015). A comparison between the nephrotoxic profile of gentamicin and gentamicin nanoparticles in mice. Journal of Biochemical and Molecular Toxicology, 29(2), 57–62. https://doi.org/10.1002/jbt.21667
- Jüngst, C., & Lammert, F. (2013). Cholestatic liver disease. Digestive Diseases (Basel, Switzerland)), 31(1), 152–154. https://doi.org/10.1159/000347210
- Katalinic, V., Modun, D., Music, I., & Boban, M. (2005). Gender differences in antioxidant capacity of rat tissues determined by 2,2'-azinobis (3-ethylbenzothiazoline 6-sulfonate; ABTS) and ferric reducing antioxidant power (FRAP) assays . Comparative Biochemistry and Physiology. Toxicology & Pharmacology : CBP, 140(1), 47–52. https://doi.org/10.1016/j.cca.2005.01.005
- Khansari, N., Shakiba, Y., & Mahmoudi, M. (2009). Chronic inflammation and oxidative stress as a major cause of age-related diseases and cancer. Recent Patents on Inflammation & Allergy Drug Discovery, 3(1), 73–80. https://doi.org/10.2174/187221309787158371
- Krones, E., Wagner, M., Eller, K., Rosenkranz, A. R., Trauner, M., & Fickert, P. (2015). Bile acid-induced cholemic nephropathy. Digestive Diseases (Basel, Switzerland), 33(3), 367–375. https://doi.org/10.1159/000371689
- Kumar, D., & Tandon, R. K. (2001). Use of ursodeoxycholic acid in liver diseases. Journal of Gastroenterology and Hepatology, 16(1), 3–14. https://doi.org/10.1046/j.1440-1746.2001.02376.x
- Lasram, M. M., Dhouib, I. B., Annabi, A., El Fazaa, S., & Gharbi, N. (2015). A review on the possible molecular mechanism of action of N-acetylcysteine against insulin resistance and type-2 diabetes development. Clinical Biochemistry, 48(16–17), 1200–1208. https://doi.org/10.1016/j.clinbiochem.2015.04.017
- Levine, R. L., Garland, D., Oliver, C. N., Amici, A., Climent, I., Lenz, A.-G., Ahn, B.-W., Shaltiel, S., & Stadtman, E. R. (1990). Determination of carbonyl content in oxidatively modified proteins. Methods in Enzymology, 186, 464–478. https://doi.org/10.1016/0076-6879(90)86141-H
- Levy, C. (2013). Cholestatic liver diseases, an issue of clinics in liver disease. Elsevier Health Sciences. https://doi.org/10.1016/j.cld.2013.01.001
- Li, T., & Chiang, J. Y. L. (2017). Bile acid-induced liver injury in cholestasis. In W.-X. Ding & X.-M. Yin (Eds.), Cellular injury in liver diseases (pp. 143–172).Springer International Publishing.
- Lino, M., Binaut, R., Noël, L.-H., Patey, N., Rustin, P., Daniel, L., Serpaggi, J., Varaut, A., Vanhille, P., Knebelmann, B., Grünfeld, J.-P., & Fakhouri, F. (2005). Tubulointerstitial nephritis and fanconi syndrome in primary biliary cirrhosis. American Journal of Kidney Diseases : The Official Journal of the National Kidney Foundation, 46(3), e41–e46. https://doi.org/10.1053/j.ajkd.2005.05.021
- Ljubuncic, P., Tanne, Z., & Bomzon, A. (2000). Evidence of a systemic phenomenon for oxidative stress in cholestatic liver disease. Gut, 47(5), 710–716. https://doi.org/10.1136/gut.47.5.710
- Malik, S. G., Irwanto, K. A., Ostrow, J. D., & Tiribelli, C. (2010). Effect of bilirubin on cytochrome c oxidase activity of mitochondria from mouse brain and liver. BMC Research Notes, 3, 162. https://doi.org/10.1186/1756-0500-3-162
- Martínez-Cecilia, D., Reyes-Díaz, M., Ruiz-Rabelo, J., Gomez-Alvarez, M., Villanueva, C. M., Álamo, J., Muntané, J., & Padillo, F. J. (2016). Oxidative stress influence on renal dysfunction in patients with obstructive jaundice: A case and control prospective study. Redox Biology, 8, 160–164. https://doi.org/10.1016/j.redox.2015.12.009
- Marullo, R., Werner, E., Degtyareva, N., Moore, B., Altavilla, G., Ramalingam, S. S., & Doetsch, P. W. (2013). Cisplatin induces a mitochondrial-ROS response that contributes to cytotoxicity depending on mitochondrial redox status and bioenergetic functions. PLoS One, 8(11), e81162. https://doi.org/10.1371/journal.pone.0081162
- Meeks, R. G., & Harrison, S. (1991). Hepatotoxicology. CRC Press.
- Mokhtari, V., Afsharian, P., Shahhoseini, M., Kalantar, S. M., & Moini, A. (2017). A review on various uses of N-acetyl cysteine. Cell Journal, 19(1), 11–17. https://doi.org/10.22074/cellj.2016.4872
- Mormone, E., George, J., & Nieto, N. (2011). Molecular pathogenesis of hepatic fibrosis and current therapeutic approaches. Chemico-Biological Interactions, 193(3), 225–231. https://doi.org/10.1016/j.cbi.2011.07.001
- Mustafa, M. G., Cowger, M. L., & King, T. E. (1969). Effects of bilirubin on mitochondrial reactions. The Journal of Biological Chemistry, 244(23), 6403–6414. doi:
- Niknahad, H., Heidari, R., Alzuhairi, A. M., & Najibi, A. (2015). Mitochondrial dysfunction as a mechanism for pioglitazone-induced injury toward HepG2 cell line. Pharmaceutical Sciences, 20, 169–174. https://doi.org/10.5681/PS.2015.007
- Niknahad, H., Jamshidzadeh, A., Heidari, R., Zarei, M., & Ommati, M. M. (2017). Ammonia-induced mitochondrial dysfunction and energy metabolism disturbances in isolated brain and liver mitochondria, and the effect of taurine administration: Relevance to hepatic encephalopathy treatment. Clinical and Experimental Hepatology, 3, 141–151. https://doi.org/10.5114/ceh.2017.68833
- O’Brien, A., China, L., Massey, K. A., Nicolaou, A., Winstanley, A., Newson, J., Hobbs, A., Audzevich, T., & Gilroy, D. W. (2016). Bile duct-ligated mice exhibit multiple phenotypic similarities to acute decompensation patients despite histological differences . Liver International: Official Journal of the International Association for the Study of the Liver, 36(6), 837–846. https://doi.org/10.1111/liv.12876
- Ohara, M., Ogawa, K., Suda, G., Kimura, M., Maehara, O., Shimazaki, T., Suzuki, K., Nakamura, A., Umemura, M., Izumi, T., Kawagishi, N., Nakai, M., Sho, T., Natsuizaka, M., Morikawa, K., Ohnishi, S., & Sakamoto, N. (2018). L-carnitine suppresses loss of skeletal muscle mass in patients with liver cirrhosis. Hepatology Communications, 2(8), 910–922. https://doi.org/10.1002/hep4.1207
- Ommati, M. M., Heidari, R., Ghanbarinejad, V., Abdoli, N., & Niknahad, H. (2019). Taurine treatment provides neuroprotection in a mouse model of manganism. Biological Trace Element Research, 190(2), 384–395. https://doi.org/10.1007/s12011-018-1552-2
- Ommati, M. M., Heidari, R., Jamshidzadeh, A., Zamiri, M. J., Sun, Z., Sabouri, S., Wang, J., Ahmadi, F., Javanmard, N., Seifi, K., Mousapour, S., & Yeganeh, B. S. (2018). Dual effects of sulfasalazine on rat sperm characteristics, spermatogenesis, and steroidogenesis in two experimental models. Toxicology Letters, 284, 46–55. https://doi.org/10.1016/j.toxlet.2017.11.034
- Ommati, M. M., Jamshidzadeh, A., Niknahad, H., Mohammadi, H., Sabouri, S., Heidari, R., & Abdoli, N. (2017). N-acetylcysteine treatment blunts liver failure-associated impairment of locomotor activity. PharmaNutrition, 5(4), 141–147. https://doi.org/10.1016/j.phanu.2017.10.003
- Orellana, M., Rodrigo, R., Thielemann, L., & Guajardo, V. (2000). Bile duct ligation and oxidative stress in the rat: effects in liver and kidney. Comparative Biochemistry and Physiology Part C: Pharmacology, Toxicology and Endocrinology, 126(2), 105–111. https://doi.org/10.1016/S0742-8413(00)00102-X
- Palmeira, C. M., & Rolo, A. P. (2004). Mitochondrially-mediated toxicity of bile acids. Toxicology, 203(1–3), 1–15. https://doi.org/10.1016/j.tox.2004.06.001
- Pastor, A., Collado, P. S., Almar, M., & González-Gallego, J. (1997). Antioxidant enzyme status in biliary obstructed rats: effects of N-acetylcysteine. Journal of Hepatology, 27(2), 363–370. https://doi.org/10.1016/S0168-8278(97)80183-3
- Paumgartner, G., & Beuers, U. (2002). Ursodeoxycholic acid in cholestatic liver disease: Mechanisms of action and therapeutic use revisited. Hepatology (Baltimore, Md.), 36(3), 525–531. https://doi.org/10.1053/jhep.2002.36088
- Perez, M. J., & Briz, O. (2009). Bile-acid-induced cell injury and protection. World Journal of Gastroenterology, 15(14), 1677–1689. https://doi.org/10.3748/wjg.15.1677
- Poli, G. (2000). Pathogenesis of liver fibrosis: Role of oxidative stress. Molecular Aspects of Medicine, 21(3), 49–98. https://doi.org/10.1016/S0098-2997(00)00004-2
- Poupon, R. (2010). Primary biliary cirrhosis: A 2010 update. Journal of Hepatology, 52(5), 745–758. https://doi.org/10.1016/j.jhep.2009.11.027
- Rodrigues, C. M., Fan, G., Ma, X., Kren, B. T., & Steer, C. J. (1998). A novel role for ursodeoxycholic acid in inhibiting apoptosis by modulating mitochondrial membrane perturbation. Journal of Clinical Investigation, 101(12), 2790–2799. https://doi.org/10.1172/JCI1325
- Rolo, A. P., Oliveira, P. J., Moreno, A. J. M., & Palmeira, C. M. (2000). Bile acids affect liver mitochondrial bioenergetics: Possible relevance for cholestasis therapy. Toxicological Sciences : An Official Journal of the Society of Toxicology, 57(1), 177–185. https://doi.org/10.1093/toxsci/57.1.177
- Rolo, A. P., Palmeira, C. M., & Wallace, K. B. (2003). Mitochondrially mediated synergistic cell killing by bile acids. Biochimica et Biophysica Acta (BBA) - Molecular Basis of Disease, 1637(1), 127–132. https://doi.org/10.1016/S0925-4439(02)00224-7
- Romero-Gómez, M., Montagnese, S., & Jalan, R. (2015). Hepatic encephalopathy in patients with acute decompensation of cirrhosis and acute-on-chronic liver failure. Journal of Hepatology, 62(2), 437–447. https://doi.org/10.1016/j.jhep.2014.09.005
- Samuni, Y., Goldstein, S., Dean, O. M., & Berk, M. (2013). The chemistry and biological activities of N-acetylcysteine. Biochimica et Biophysica Acta, 1830(8), 4117–4129. https://doi.org/10.1016/j.bbagen.2013.04.016
- Šebeková, K. n., Kupčová, V., Schinzel, R., & Heidland, A. (2002). Markedly elevated levels of plasma advanced glycation end products in patients with liver cirrhosis – amelioration by liver transplantation. Journal of Hepatology, 36(1), 66–71. https://doi.org/10.1016/S0168-8278(01)00232-X
- Shafaroodi, H., Ebrahimi, F., Moezi, L., Hashemi, M., Doostar, Y., Ghasemi, M., & Dehpour, A. R. (2010). Cholestasis induces apoptosis in mice cardiac cells: The possible role of nitric oxide and oxidative stress. Liver International : official Journal of the International Association for the Study of the Liver, 30(6), 898–905. https://doi.org/10.1111/j.1478-3231.2010.02249.x
- Sheen, J.-M., Huang, L.-T., Hsieh, C.-S., Chen, C.-C., Wang, J.-Y., & Tain, Y.-L. (2010). Bile duct ligation in developing rats: Temporal progression of liver, kidney, and brain damage. Journal of Pediatric Surgery, 45(8), 1650–1658. https://doi.org/10.1016/j.jpedsurg.2009.12.019
- Shikata, F., Sakaue, T., Nakashiro, K-i., Okazaki, M., Kurata, M., Okamura, T., Okura, M., Ryugo, M., Nakamura, Y., Yasugi, T., Higashiyama, S., & Izutani, H. (2014). Pathophysiology of lung injury induced by common bile duct ligation in mice. PLoS One, 9(4), e94550. https://doi.org/10.1371/journal.pone.0094550
- Silva, R. F. M., Rodrigues, C. l M. P., & Brites, D. (2001). Bilirubin-induced apoptosis in cultured rat neural cells is aggravated by chenodeoxycholic acid but prevented by ursodeoxycholic acid. Journal of Hepatology, 34(3), 402–408. https://doi.org/10.1016/S0168-8278(01)00015-0
- Singh, S., Shackleton, G., Ah-Sing, E., Chakraborty, J., & Bailey, M. E. (1992). Antioxidant defenses in the bile duct-ligated rat. Gastroenterology, 103(5), 1625–1629. https://doi.org/10.1016/0016-5085(92)91187-9
- Soares, J.-B., Pimentel-Nunes, P., Roncon-Albuquerque, R., & Leite-Moreira, A. (2010). The role of lipopolysaccharide/toll-like receptor 4 signaling in chronic liver diseases. Hepatology International, 4(4), 659–672. https://doi.org/10.1007/s12072-010-9219-x
- Socci, D. J., Bjugstad, K. B., Jones, H. C., Pattisapu, J. V., & Arendash, G. W. (1999). Evidence that oxidative stress is associated with the pathophysiology of inherited hydrocephalus in the H-Tx rat model. Experimental Neurology, 155(1), 109–117. https://doi.org/10.1006/exnr.1998.6969
- Sokol, R. J., Devereaux, M., Dahl, R., & Gumpricht, E. (2006). “Let there be bile”-understanding hepatic injury in cholestasis”– . Journal of pediatric gastroenterology and nutrition, 43 Suppl 1(Suppl 1), S4–S9. https://doi.org/10.1097/01.mpg.0000226384.71859.16
- Sun, Y., Pu, L.-Y., Lu, L., Wang, X.-H., Zhang, F., & Rao, J.-H. (2014). N-acetylcysteine attenuates reactive-oxygen-species-mediated endoplasmic reticulum stress during liver ischemia-reperfusion injury. World Journal of Gastroenterology, 20(41), 15289–15298. https://doi.org/10.3748/wjg.v20.i41.15289
- Tag, C. G., Sauer-Lehnen, S., Weiskirchen, S., Borkham-Kamphorst, E., Tolba, R. H., Tacke, F., & Weiskirchen, R. (2015). Bile duct ligation in mice: Induction of inflammatory liver injury and fibrosis by obstructive cholestasis. Journal of Visualized Experiments, (96), 52438. https://doi.org/10.3791/52438
- Tag, C. G., Weiskirchen, S., Hittatiya, K., Tacke, F., Tolba, R. H., & Weiskirchen, R. (2015). Induction of experimental obstructive cholestasis in mice. Laboratory Animals, 49(1 Suppl), 70–80. https://doi.org/10.1177/0023677214567748
- Tahan, G., Tarcin, O., Tahan, V., Eren, F., Gedik, N., Sahan, E., Biberoglu, N., Guzel, S., Bozbas, A., Tozun, N., & Yucel, O. (2007). The effects of N-acetylcysteine on bile duct ligation-induced liver fibrosis in rats. Digestive Diseases and Sciences, 52(12), 3348–3354. https://doi.org/10.1007/s10620-006-9717-9
- Truong, D. H., Eghbal, M. A., Hindmarsh, W., Roth, S. H., & O’Brien, P. J. (2006). Molecular mechanisms of hydrogen sulfide toxicity. Drug Metabolism Reviews, 38(4), 733–744. https://doi.org/10.1080/03602530600959607
- van Slambrouck, C. M., Salem, F., Meehan, S. M., & Chang, A. (2013). Bile cast nephropathy is a common pathologic finding for kidney injury associated with severe liver dysfunction. Kidney International, 84(1), 192–197. https://doi.org/10.1038/ki.2013.78
- Vaz, A. R., Delgado‐Esteban, M., Brito, M. A., Bolaños, J. P., Brites, D., & Almeida, A. (2010). Bilirubin selectively inhibits cytochrome c oxidase activity and induces apoptosis in immature cortical neurons: Assessment of the protective effects of glycoursodeoxycholic acid. Journal of Neurochemistry, 112(1), 56–65. https://doi.org/10.1111/j.1471-4159.2009.06429.x
- Vinken, M. (2019). Experimental cholestasis research. Springer.
- Woolbright, B. L., & Jaeschke, H. (2012). Novel insight into mechanisms of cholestatic liver injury. World Journal of Gastroenterology, 18(36), 4985–4993. https://doi.org/10.3748/wjg.v18.i36.4985
- Wright, D. J., Renoir, T., Smith, Z. M., Frazier, A. E., Francis, P. S., Thorburn, D. R., McGee, S. L., Hannan, A. J., & Gray, L. J. (2015). N-Acetylcysteine improves mitochondrial function and ameliorates behavioral deficits in the R6/1 mouse model of Huntington’s disease. Translational Psychiatry, 5, e492. https://doi.org/10.1038/tp.2014.131
- Xiao, H., Wu, M., Shao, F., Guan, G., Huang, B., Tan, B., & Yin, Y. (2016). N-acetyl-L-cysteine protects the enterocyte against oxidative damage by modulation of mitochondrial function. Mediators of Inflammation, 2016, 1–9. https://doi.org/10.1155/2016/8364279
- Yang, H., Ramani, K., Xia, M., Ko, K. S., Li, T. W. H., Oh, P., Li, J., & Lu, S. C. (2009). Dysregulation of glutathione synthesis during cholestasis in mice: Molecular mechanisms and therapeutic implications. Hepatology (Baltimore, Md.), 49(6), 1982–1991. https://doi.org/10.1002/hep.22908
- Yang, Y.-Y., Lee, K.-C., Huang, Y.-T., Wang, Y.-W., Hou, M.-C., Lee, F.-Y., Lin, H.-C., & Lee, S.-D. (2008). Effects of N-acetylcysteine administration in hepatic microcirculation of rats with biliary cirrhosis. Journal of Hepatology, 49(1), 25–33. https://doi.org/10.1016/j.jhep.2008.02.012
- Zhang, Y.-T., Zheng, Q.-S., Pan, J., & Zheng, R.-L. (2004). Oxidative damage of biomolecules in mouse liver induced by morphine and protected by antioxidants. Pharmacology and Toxicology, 95(2), 53–58. https://doi.org/10.1111/j.1742-7843.2004.950202.x