Abstract
Previous literature links maternal pregnancy-specific anxiety (PSA) with later difficulties in child emotional and social cognition as well as memory, functions closely related to the amygdala and the hippocampus. Some evidence also suggests that PSA affects child amygdalar volumes in a sex-dependent way. However, no studies investigating the associations between PSA and newborn amygdalar and hippocampal volumes have been reported. We investigated the associations between PSA and newborn amygdalar and hippocampal volumes and whether associations are sex-specific in 122 healthy newborns (68 males/54 females) scanned at 2–5 weeks postpartum. PSA was measured at gestational week 24 with the Pregnancy-Related Anxiety Questionnaire Revised 2 (PRAQ-R2). The associations were analyzed with linear regression controlling for confounding variables. PSA was associated positively with left amygdalar volume in girls, but no significant main effect was found in the whole group or in boys. No significant main or sex-specific effect was found for hippocampal volumes. Although this was an exploratory study, the findings suggest a sexually dimorphic association of mid-pregnancy PSA with newborn amygdalar volumes.
1. Introduction
The amygdala and the hippocampus are part of the fear circuitry – a system relevant in stress responses and later psychiatric symptomatology, such as anxiety (Richter-Levin, Citation2004; Shin & Liberzon, Citation2010; Van den Bergh et al., Citation2018). Severe anxiety has been associated with amygdalar hyperactivation and enlargement as well as hippocampal volume reduction in adults (Kolesar et al., Citation2019), supporting the evidence found in prenatal stress studies in animals (Charil et al., Citation2010). Trying to unravel the predisposition to psychiatric disorders, researchers have peered into the role of prenatal maternal psychological distress (PPD) in offspring neurodevelopment. Along with other forms of maternal prenatal distress, pregnancy-specific anxiety (PSA) has been associated with many adverse child health outcomes, such as lower birth weight, problems in attention regulation and executive functions as well as negative emotional reactivity and lower mental and motor developmental scores (Blackmore et al., Citation2016; Buss et al., Citation2011; Huizink et al., Citation2004; Nolvi et al., Citation2016). However, unlike other PPD, high PSA is currently not considered a diagnosis, and thus, it is not screened for in clinical settings. Further, despite the shared associations with other types of PPD, previous studies suggest that PSA should be considered distinct from prenatal general anxiety and depressive symptoms (Huizink et al., Citation2004).
Unlike other distress types, PSA is characterized as excessive worry or distress specific to pregnancy, including health of the developing child, changes in own appearance, labor and birth, as well as future parenting concerns (Blackmore et al., Citation2016). The suggested distinction of PSA from other types of distress is based on several observations. First, studies have found that there is only modest overlap between measures of PSA and conventional general anxiety and depression questionnaires (Blackmore et al., Citation2016; Huizink et al., Citation2004). Second, PSA can also be discriminated from other distress symptoms by external factors, such as maternal age (Blackmore et al., Citation2016). Third, PSA predicts external outcomes, such as birth weight, gestational age at birth, and postnatal mood disturbance independently of conventional symptom measures (Blackmore et al., Citation2016; Kramer et al., Citation2009; Wadhwa et al., Citation1993). Finally, PSA appears to predict child outcomes more strongly than indices of general anxiety and depression (Bayrampour et al., Citation2016; Brunton et al., Citation2019; Huizink et al., Citation2004; Theut et al., Citation1988). PSA has been associated e.g. with child attention regulation problems, weaker cognitive functions, socioemotional and behavioral problems, and anxiety symptoms (Acosta et al., Citation2019; Blackmore et al., Citation2016; Buss et al., Citation2011; Davis & Sandman, Citation2012; Huizink et al., Citation2002; Nolvi et al., Citation2016). Unfortunately, the mechanisms through which the different distress types act on fetal brain development are yet unknown and thus we cannot make any hypotheses on whether PSA works through the same or distinct mechanisms compared to other types of maternal distress.
Despite the growing evidence of higher maternal PSA having a negative effect on child neurodevelopment, the relationship between PSA and offspring amygdalar and hippocampal outcomes is understudied. Another study conducted by our research group is the only study, thus far, reporting results in children and concerning PSA in specific as the exposure. This study observed larger left amygdalar volumes in 4-year-old females compared to males (GW 24) (Acosta et al., Citation2019). This finding is consistent with newborn/child studies on prenatal depression and/or state/trait anxiety, which have reported larger amygdalar volumes after prenatal distress exposure (Acosta et al., Citation2020; Qiu et al., Citation2017; Wen et al., Citation2017). However, no studies on the potential effects of PSA on the offspring brain have been conducted in newborns, and no study thus far has investigated the potential associations between PSA and hippocampal volumes. It is crucial to obtain more information on this relationship early-on, to understand how PSA may influence the key parts of the developing limbic system involved in cognition, emotional behavior, and stress regulation as well as the development of psychopathologies, especially mood disorders (Van den Bergh et al., Citation2018).
In this study, we investigated the associations between PSA and newborn amygdalar and hippocampal volumes. Additionally, as sex differences have earlier been observed after exposure to maternal PPD (Acosta et al., Citation2019; Lehtola et al., Citation2020; Wen et al., Citation2017), we also investigated, whether the associations are sex-specific. Given the results in human and animal studies (Charil et al., Citation2010; Kolesar et al., Citation2019; Van den Bergh et al., Citation2005), we expected PSA to be positively associated with amygdalar volumes in females and negatively with hippocampal volumes, regardless of child sex. We chose to concentrate on the second trimester as the time of PSA exposure as it has been repeatedly associated with findings related to PPD in previous studies (Acosta et al., Citation2019; Qiu et al., Citation2013, Citation2017; Rifkin-Graboi et al., Citation2013; Wen et al., Citation2017).
2. Methods
The study was conducted according to the Declaration of Helsinki and was reviewed and approved by the Ethics Committee of the Hospital District of Southwest Finland (ETMK:31/180/2011).
2.1. Participants
Mothers involved in this study were drawn from the broader FinnBrain Birth Cohort Study (Karlsson et al., Citation2018). They were recruited at three healthcare locations in Southwest Finland during their first trimester ultrasound visits at gestational week (GW) 12. From this broader participant pool, 189 newborn-mother dyads were recruited into this study. They were recruited based on willingness to participate and availability of the newborn to have an MRI after birth. After explaining the study’s purpose and protocol, written informed consent was obtained from the parent(s). Of these 189 newborn participants, 64 had motion artifacts in the MR images, leaving 125 images for further segmentation analyses. Additionally, three mothers had missing prenatal questionnaires. Therefore, 122 newborn-mother dyads were eligible for statistical analyses.
The demographics of the participants and the excluded cases are presented in . Ethnicity was not inquired but the study population was predominantly Caucasian. Newborns were generally scanned two to five weeks after birth, although eleven scans were performed after this age, and three before (). All newborns exceeded 2500 g of weight at birth and all but one was born full-term [between GW37 and GW42; the single preterm-born at GW 36.3]. Newborns’ background information was gathered from the Finnish Medical Birth Register (FMBR) kept by the Finnish Institute for Health and Welfare (www.thl.fi): 14 newborns had mild asphyxia, one needed cardiopulmonary resuscitation (CPR) and respirator treatment, and one individual scored four points in the five-minute Apgar. These birth complications were included in a sensitivity analysis. Maternal background information was gathered by questionnaires and included monthly income, educational level, diagnosed medical conditions, central nervous system (CNS) affecting medications, and substance use during pregnancy. For CNS-affecting medications, only serotonin and norepinephrine reuptake inhibitors or benzodiazepines were reported. No substance use other than that of alcohol or tobacco was reported. Obstetric data were retrieved from the FMBR.
Table 1. Descriptives of the study participants and excluded individuals.
2.2. Maternal psychological distress questionnaire data
Maternal pregnancy-specific anxiety was measured at GW24 with Pregnancy-Related Anxiety Questionnaire Revised 2 (PRAQ-R2). PRAQ-Revised (PRAQ-R), a shortened version of the original PRAQ (Bergh, Citation1990), is a commonly used questionnaire to measure anxiety during pregnancy in primiparous women (Huizink et al., Citation2004). A rephrased version, PRAQ-R2, was successfully created to expand the applicability also to multiparous women (Huizink et al., Citation2016), and it has good to excellent internal consistency overall and at subscale level as well as strong metric and scalar invariance, indicating that the PRAQ-R2 measures similar constructs on the same scale for all pregnant women (Huizink et al., Citation2016). PRAQ-R2 has ten items with a range of five answers from “definitely not true” to “definitely true”, forming a total score range of 0-50 points.
The items in PRAQ-R2 can be ordered into three subscales:
Fear of giving birth (three items)
Worries about bearing a physically or mentally handicapped child (four items)
Concern about own appearance (three items)
To control for other symptoms of anxiety/depression, two additional distress scores were measured at GW24: Edinburgh Postnatal Depression Scale (EPDS) (Cox et al., Citation1987) for depressive symptoms and the anxiety subscale of Symptom Checklist 90 (SCL-90) for general (as opposed to pregnancy-related) anxiety (Deogratis et al., Citation1973). Although originally developed for screening postnatal depression, EPDS is also valid during pregnancy (Bergink et al., Citation2011; Bunevicius et al., Citation2009; Rubertsson et al., Citation2011; Tendais et al., Citation2014). The EPDS consists of 10 items and its score ranges from 0 to 30. The anxiety subscale of SCL-90 is a standard tool for measuring general anxiety (Bech et al., Citation2014; Deogratis et al., Citation1973) and widely used during the prenatal period (Adib-Rad et al., Citation2019; Kamel et al., Citation1999; Lin et al., Citation2017; van den Heuvel et al., Citation2015;). It consists of 10 items and the total score range is 0-40. EPDS and SCL-90 scores were combined to create an overall distress score by first standardizing the scores (mean = 0, SD = 1) and then summing the standardized values to create a composite score, “SCL + EPDS”.
The number of missing data for PRAQ-R2, EPDS, and SCL-90 was three, i.e. three mothers provided none of the three distress scores at the GW 24 measurement point. These mother-newborn dyads were excluded from the study sample as described above. For partial missing data a maximum of one missing item was tolerated per PRAQ-R2 factor (f1-f3) and in this case, the missing value was imputed with the arithmetic mean of the available values in the same factor.
2.3. MRI acquisition
A detailed description of the scanning protocol is provided in our previous publication (Lehtola et al., Citation2019). All the scans were performed on the same site with the same scanner and scanning parameters. Participants were scanned without anesthesia with a Siemens Magnetom Verio 3 T scanner (Siemens Medical Solutions, Erlangen, Germany). The 40-minute imaging protocol included an axial PD-T2-TSE (Dual-Echo Turbo Spin Echo) and a sagittal 3 D-T1 MPRAGE (Magnetization Prepared Rapid Acquisition Gradient Echo) sequences with isotropic 1.0 mm3 voxels and whole brain coverage. Repetition time (TR) time of 12 070 ms and effective Echo time (TE) of 13 ms and 102 ms were used in PD-T2 TSE sequence to produce both PD-weighted and T2-weighted images from the same acquisition. Total number of 1 mm thick slices was 128. TR of 1900 ms, TE of 3.26 ms, and inversion time (TI) of 900 ms were used for 3 D-T1-MPRAGE sequence. The number of slices was 176.
Table 2. Mean raw and relative brain volumes.
All brain images were assessed for incidental findings by a pediatric neuroradiologist (author RP). If found, parents were given a follow-up opportunity with a pediatric neurologist (author TL). Developmental status was age appropriate by the age of two years for all participants. The incidental findings (intracranial hemorrhages, N = 12, 6.9%) have been found to be common and clinically insignificant in previous studies (Rooks et al., Citation2008; Whitby et al., Citation2004). Intracranial hemorrhages were deemed not to affect volumetric estimates of interest and thus, did not exclude the individuals from the current study (Kumpulainen et al., Citation2020). All the brain images were checked visually by multiple researchers for motion artifacts, initially by three independent raters and the final segmentations were viewed by authors JDL and JJT. No specific rating scale for artifacts was used but a binary classification.
2.4. Construction of an unbiased population-specific template
The measurements used in analysis were derived using fusion-based methods that rely on a labeled template. These methods depend on achieving good registrations between the subjects and the template. This is increasingly difficult to achieve the further the template is from the subjects in terms of similarity. Thus, a template was constructed based on the subjects in this study; then the structures of interest were manually labeled, and a mask was automatically extracted for use in estimating total brain volume (TBV).
All 125 MRIs were used to construct a population-specific dual-contrast template. The template construction process has been previously published (Fonov et al., Citation2011). It is an iterative process that, given a set of MRI volumes, constructs a template, which minimizes the mean squared intensity difference between the template and each subject’s MRI, and minimizes the magnitude of all deformations used to map the template to each subject’s MRI. This method was applied to the T1 scans. The T1 scans were linearly registered to the MNI 152 template. The average scaling from the native MRIs to the MNI 152 template was then computed, and the inverse used to scale the MNI 152 template to the average size of the study population, which served as an initial target for construction of the population-specific template. The iterative template construction procedure was then applied producing the T1 template as well as non-linear transformations between each T1 and the T1 template. The T2 native scans were then registered to the T1 native scans, and the resulting transform was concatenated with the linear and non-linear transforms taking that T1 to the T1 template. These composite transformations were then used to map the T2 scans to template space, where they were averaged to create the T2 template.
2.5. Labeling the template
The structures of interest, the amygdalae and hippocampi, were manually labeled on the dual-contrast template. To ensure these labels were accurate, multiple variants of the template were produced, and each variant was manually labeled without the raters knowing that they were not actual subject data. Altogether, 21 variants were produced, representing well the morphological variation in the data, each a non-linear transformation of the template to overlay one of the subjects in the population. The non-linear transformations derived from the template construction procedure were used to cluster the subjects into 21 groups where the anatomical within-group variability was smaller than the inter-group variability. As the basis for clustering, the Jacobian was computed for the non-linear transform mapping each subject to the template. The values in the Jacobian were then extracted as a vector for each voxel within the template brain mask. These Jacobian vectors were then clustered using an equal combination of cosine similarity and Euclidean distance with Ward’s clustering method (Ward, Citation1963), with the number of clusters chosen to be 21. Then, within each cluster, the sum-squared distance from each subject to each other subject was computed, and the subject with the minimum sum-squared distance was taken as the central-most subject of the cluster. The dual-contrast template constructed in the previous step was then warped to these 21 representative subjects, and provided for manual segmentation.
Segmentation was done following standard procedures (Hashempour et al., Citation2019). One template was first segmented by the primary rater NH and subsequently by senior rater JJT and externally reviewed by JDL. Once the first segmentation was deemed satisfactory, the other templates were then segmented. The 21 manual segmentations were then warped back to the standard template, and each voxel was assigned a label based on the majority vote across all 21 manual segmentations. This yielded the final labels for the amygdalae and hippocampi on the standard template. The generalized conformity index (GCI) was calculated to determine the inter-rater agreement in spatial overlap for the newborn template (left hippocampus: CGI= 0.77, right hippocampus: CGI= 0.76, left amygdala: CGI= 0.72, right amygdala: CGI= 0.69), and the agreement was deemed to be excellent; CGI scores of 0.7–1.0 are regarded as excellent (Kouwenhoven et al., Citation2009; Visser et al., Citation2019). The inter-rater reliabilities were used as the primary quality assessment. It was deemed good and visual representation of this is provided in our prior publication – please see Figure 3 in Acosta et al. (Citation2020).
2.6. Labeling the subjects
Segmentation into left and right amygdalae and hippocampi was done for each subject using a label-fusion-based labeling technique based on Coupé et al. (Citation2011) and further developed by Weier et al. (Citation2014) and Lewis et al. (Citation2019). The approach uses a population-specific template library. The library was constructed by clustering deformation fields from the non-linear transforms produced during construction of the template and using the central-most subject of each cluster to construct the entries in the template library; this is similar to the method described above, but in this case, the clustering used a dilated mask of the amygdalae and hippocampi to capture the anatomical context of the nonlinear registration in that region of the brain, and the number of clusters was the square of the natural log of the number of subjects. The representative subject for each cluster was chosen as described above. This was done per hemisphere to accommodate hemispheric asymmetries. Thus, the template library represented the range of deformations of the amygdalae and hippocampi found in the population.
To create the library entry for a cluster, the non-linear transform for the central-most subject was used to warp the template together with the segmentation defined on it, and this pair was added to the template library. The template library was thus a set of warped copies of the template together with their correspondingly warped segmentations. Once the template library had been created, each subject in the population was non-linearly registered to the n closest templates in the library (here, n = 7), and the resulting transforms were used to warp their corresponding segmentations to the subject; the final labeling was then established patch-based label fusion. This was also done separately for each hemisphere. An example of such a labeling is shown in . The volumes of each of the final labellings were then computed and scaled to native space based on the scaling factors in the subject’s linear transforms.
Figure 1. Example of the segmentation of amygdala and hippocampus in a study subject. The amygdala and hippocampus are shown in coronal, axial, and sagittal planes with orange and blue colors respectively.
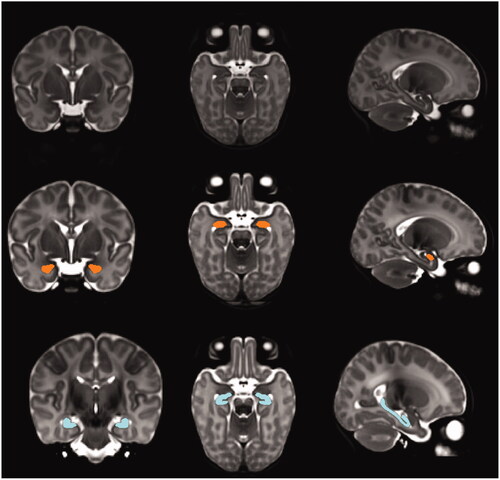
2.7. Code availability
Custom computer codes were used for data analysis. The core of the code to construct the library, and also to perform the fusion labeling can be found in https://github.com/NIST-MNI/nist_mni_pipelines/blob/master/iplScoopFusionSegmentation.py. Please contact the corresponding author for other code related questions.
2.8. Statistical analysis
IBM SPSS Statistics Version 26 for Microsoft was used for statistical analyses (Armonk, NY: IBM Corp.) and R Version 3.6.0 for visualization of the results as scatter plots (Vienna, Austria: R Core Team). Data normality for descriptive variables, brain volumetric measures, and distress scores were checked by visual inspection and by the Shapiro-Wilk test. Dependent variables used in the analyses were the relative (raw volume divided by TBV) volumes for the left and right amygdala and hippocampus (). The birthweights were adjusted to gestational age to investigate if there were large differences between individuals referring to small- or large-for-gestational-age conditions. All newborns, except one, were found to be appropriate for gestational age, thus the original birthweight value was used. The median value was used to replace the missing values of birthweight (n = 1) and maternal BMI (n = 2). The most common education level (3) was used to replace the missing values in maternal education (n = 2, male newborn n = 1).
Associations between the study variables (maternal and newborn health parameters, distress questionnaire scoring, volumetric measures) were investigated with zero-order Pearson correlations (). Independent samples T-test and chi-square tests were used to detect sex differences in newborn variables (newborn age, birthweight, birth complications), maternal variables (maternal age, pre-pregnancy BMI, prenatal alcohol and/or nicotine consumption, prenatal CNS affecting medication, maternal education, gestational diabetes, high blood pressure, or moderate pre-eclampsia) (), and mean brain volumes (). Sex differences in distress scores (maternal PRAQ-R2, SCL + EPDS composite scores) were analyzed with the Mann-Whitney U Test.
Table 3. Correlations between the study variables and their p values.
General linear modeling (GLM) was used to investigate the relationships between the PRAQ-R2 scores and volumetric measures of the amygdalae and hippocampi. The GLM method was chosen because of the intercorrelations of the predictor variables. The GLM analyses were performed in three parts: the first part investigated the main effect of PSA on the volumetric measures of amygdalae and hippocampi in the whole group; the second part explored the interaction between newborn sex and maternal distress score by adding an interaction variable (sex*distress score); in the third part, the main effect analysis was repeated but separately in male and female subgroups of the sample. Covariates were added stepwise into the analyses. In the first step, newborn total age (gestational age + days counted from birth), birthweight, maternal BMI and maternal education were included. In the second step, SCL + EPDS composite score was added. The analyses were also repeated with other newborn age variables (gestational age, postnatal age in days at scan) to test the consistency of the results. These covariates were selected based on theoretical relevance for newborn brain development in line with prior literature (Caputo et al., Citation2016; Contu & Hawkes, Citation2017; de Haan et al., Citation2006; Falster et al., Citation2018; Gingrich et al., Citation2017; Holland et al., Citation2014; Knickmeyer et al., Citation2016; Little et al., Citation2021; Pesonen et al., Citation2016; Rotem-Kohavi & Oberlander, Citation2017; Sandin, Citation2012; Van Lieshout et al., Citation2011; van Soelen et al., Citation2010; Wadhwa et al., Citation1993; Wortinger et al., Citation2020; Zhu et al., Citation2010). The p values from the sixteen main analyses (shown in ) were also corrected for multiple comparisons using Benjamini-Hochberg method (Benjamini & Hochberg, Citation1995) that controls the false discovery rate (FDR). The corrections were performed separately for the eight tests including all the children (the main effects and the sex interactions) and for the eight sex-wise effects.
Table 4. Associations between Pregnancy-related anxiety Questionnaire scores at GW24 and newborn amygdalar and hippocampal volumes.
As a confirmation, five sets of sensitivity analyses were run, where the following groups were individually removed from the analyzed sample: maternal prenatal CNS affecting medication (n = 8) and/or substance use (n = 35); birth complications (n = 16); gestational diabetes (n = 15) and/or hypertension/pre-eclampsia (n = 5) (). Both gestational diabetes and pre-eclampsia have been associated with impaired cognitive development in children (Devarshi et al., Citation2019).
3. Results
3.1. Sex differences in maternal distress questionnaire data and brain volumetric measures
The mid-pregnancy PRAQ-R2 scores were higher in mothers of the male newborns than those of female newborns. However, no offspring sex difference was detected in SCL + EPDS composite scores (). All the raw volumetric measures of the amygdalae and hippocampi were greater in males than females. However, in the volumetric measures corrected with TBV, only the right amygdalar volume was significantly greater in males than females ().
3.2. Correlations between variables
PRAQ-R2 and SCL + EPDS composite scores were highly positively intercorrelated. Additionally, a strong positive correlation was observed between the right amygdala/TBV and left amygdala/TBV as well as the right amygdala/TBV and right hippocampus/TBV. The relative volumes of the left amygdala and left hippocampus were only mildly intercorrelated. Birth complications were strongly positively correlated with left hippocampal volume. All associations between study variables are displayed in .
3.3. The relationships between maternal prenatal PRAQ-R2 and newborn volumetric measures
The analysis done with the sex-interaction model revealed a significant association between the left amygdala/TBV volume and the interaction term of sex by PRAQ-R2, when controlled for newborn age, birthweight, and sex, as well as for maternal BMI and education (). The result persisted after controlling for SCL + EPDS composite score but not after correction for multiple comparisons (). In contrast, no associations were observed between the hippocampus/TBV or the right amygdala/TBV volumes and the sex-by-PRAQ-R2-interaction term. In the separate analyses for the two sexes, no significant findings were observed in males, but in females, the left amygdala/TBV volume was positively associated with maternal prenatal PRAQ-R2 (). This result was controlled for newborn age, birthweight, as well as maternal BMI and education and persisted after controlling for SCL + EPDS composite score but not after correction for multiple comparisons (). The results were similar when calculated with other newborn age variables (gestational age: B = 1.05E-8, p = .033; postnatal age in days at scan: B = 9.68E-8, p = .036). The group analyses for the hippocampi yielded no significant results in males or females. In the whole group, no significant associations between PRAQ-R2 and newborn brain volumes were found.
Figure 2. Scatter plots of the associations between Pregnancy-Related Anxiety Questionnaire Revised 2 (PRAQ-R2) at GW24 and newborn amygdalar volumes in males and females. The significant association between PRAQ-R2 and female left amygdalar volume (p = .039) is on the left.
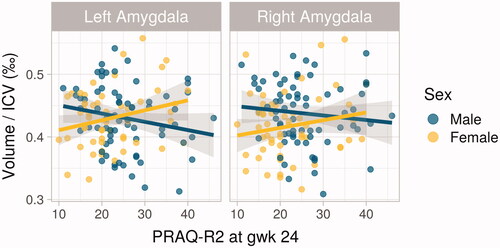
In the sensitivity analyses, the results were similar, and the association between the sex-interaction term and the left amygdala/TBV volume persisted after confounding factors were individually removed from the sample (maternal prenatal CNS affecting medication [B = 3.5E-6, p = .008] and/or substance use [B = 4.1E-6, p = .003], birth complications [B = 3.2E-6, p = .029], gestational diabetes [B = 3.7E-6, p = .012] and/or hypertension/pre-eclampsia [B = 2.7E-6, p = .036]). In the sensitivity analyses of the male and female subgoups, the association between PRAQ-R2 and female left amygdala/TBV volume persisted after removing individuals exposed to maternal prenatal CNS affecting medication (B = 3.06E-6, p = .019) and substance use (B = 3.17E-6, p = .008). However, the finding did not persist after controlling for birth complications (including mild asphyxia, CPR, or Apgar under 5 points, B = 2.12E-6, p = .055), gestational diabetes (B = 1.90E-6, p = .096), and/or hypertension/pre-eclampsia (B=-2.84E-6, p = .148).
4. Discussion
We investigated PSA in mid-pregnancy and its associations with newborn amygdalar and hippocampal volumes, and whether these associations are sex-specific. We observed that higher PSA associated with larger left amygdalar volumes in newborn females, but not in males, even after controlling for symptoms of prenatal depression and general anxiety. We observed no associations between PSA and newborn hippocampal volumes. Our result of larger left amygdalar volumes in females exposed to PSA, in line with the similar observation in 4-year-old female children from the same cohort study (Acosta et al., Citation2019), further supports the notion that PSA has a sex-specific association with the offspring amygdala.
The result of the sex-interaction model survived all sensitivity analyses, however the result of the female subgroup did not persist after controlling for gestational illnesses or birth complications, which may owe to the proportionately high number of covariates per the size of the subgroup. A similar observation to ours (i.e. positive association with right amygdalar volumes) regarding the sex difference, has also been observed after exposure to prenatal maternal depression in female children (aged 4.5 years) (Wen et al., Citation2017). However, not all studies report evidence for sex differences in amygdalar volumes after exposure to maternal psychological or psychiatric symptoms prenatally. In a study of American newborns with low polygenic risk scores for major depressive disorder, exposure to PPD, defined as maternal depression, was associated with larger right amygdalar volumes in both sexes (Qiu et al., Citation2017). The same study design was repeated with individuals of European ancestry and yielded similar results to those of the American cohort (Acosta et al., Citation2020).
Further emphasizing the sexually dimorphic associations of maternal prenatal distress with offspring amygdalar volumes, our previous study showed a negative association between maternal depressive and anxiety symptoms and male amygdalar volumes (Lehtola et al., 2020). Summing up the results of this and our previous study, different maternal distress symptomatologies (prenatal depressive and anxiety symptoms and PSA) seem to be associated with amygdalar volumes in the offspring in opposite directions, and the associations have concerned only one of the two sexes, also depending on the distress type. We propose that the results differ between studies because the distress exposures are indeed different. It is yet unknown how and to what extent PSA differs from other forms of prenatal distress and through which pathways it may affect fetal brain development. However, as stated before, PSA predicts external outcomes (birth weight, gestational age at birth, and postnatal mood disturbance) independently and child outcomes more strongly than conventional distress symptom measures (Bayrampour et al., Citation2016; Blackmore et al., Citation2016; Brunton et al., Citation2019; Huizink et al., Citation2004; Kramer et al., Citation2009; Szekely et al., Citation2021; Theut et al., Citation1988; Wadhwa et al., Citation1993), suggesting it to be a distinct exposure from general anxiety and depressive symptoms. However, this is only partially supported by extant studies as some studies on prenatal depression and anxiety replicate the findings of this study. In addition, even though we observed a correlation between PSA and SCL + EPDS scores in this study, this is likely explained by somewhat overlapping symptomatology with general anxiety (Blackmore et al., Citation2016; Huizink et al., Citation2004). In short, the mothers that are already anxious, are also more likely to suffer from pregnancy-specific anxiety (Huizink et al., Citation2014; Mudra et al., Citation2020).
Exposure to elevated PSA/prenatal anxiety increases the risk for developing anxiety problems and/or depressive symptoms during the preadolescent/adolescent period (Davis & Sandman, Citation2012; Van den Bergh et al., Citation2008), especially in females (Van den Bergh et al., Citation2008). Similar behavioral traits have been observed in the female offspring of prenatally stressed rats, when investigating the neurocircuitry of fear responses as correlates of anxiety (Ehrlich et al., Citation2015; Weinstock, Citation2011). In rats, enhanced fear conditioning has been related to smaller amygdalar size, particularly smaller basolateral amygdalar volume (Yang et al., Citation2008). In humans, the observations are mixed. Acosta et al. (Citation2019) observed that while higher PSA associated with more emotional symptoms, peer relationship problems, and overall childhood difficulties in 4-year-old males, in females, larger right amygdalar volume associated with less of these problems. Then again, increased anxiety in 7- to 9 -year-old children has been associated with increased amygdalar volumes, localized specifically to the basolateral amygdala (Qin et al., Citation2014). The observations on the interaction between the prenatal environment and offspring genetics suggest that smaller amygdalar volumes may result from when an individual possesses both genetic and environmental (maternal prenatal distress) risk factors, whereas larger volumes may follow when only one risk factor is present (Acosta et al., Citation2020). It has been hypothesized that larger amygdalar volumes in female children compared to males after exposure to PSA would be linked with greater postnatal stress reactivity in females, while the smaller amygdala in males would be related to hypercortisolaemia without emotional arousal (Acosta et al., Citation2019). Combined with the results previously published by our research team (Lehtola et al., 2020), our results show that this sex difference in amygdalar volume is already present in early postnatal life, suggesting that the sex difference may not solely be based on postnatal emotional arousal, but differences in overall early brain development of males and females in the intrauterine environment. For instance, sexual dimorphism in placental functions, such as placental steroid profile, responsiveness to cortisol (possibly via differences in glucocorticoid receptor expression and function), growth hormone concentrations, and gene expression related to immune function have been reported (Clifton, Citation2010). These differences may underlie the sex-specific alterations in amygdalar volumes, however, the evidence of these possible explanations is yet lacking and remain to be proven in future studies.
Related to distress, cortisol, being a “stress hormone”, has been investigated as a possible mediating factor. Larger right amygdalar volume and more affective problems in female children (age 7 years) have been associated with higher maternal prenatal cortisol concentrations (Buss et al., Citation2012). Although no association between PSA and high maternal cortisol concentrations has been observed (Mustonen et al., Citation2019; van den Heuvel et al., Citation2018; Vlenterie et al., Citation2021), it remains possible that the fetus is ultimately exposed to higher cortisol concentrations due to suppression of placental 11-beta-hydroxysteroid dehydrogenase 2 -enzyme caused by some forms of prenatal anxiety (Dahlerup et al., Citation2018; O’Donnell et al., Citation2012). This could in turn increase the amount of active cortisol transmission to the fetus (Dahlerup et al., Citation2018) and interfere with the function of the fetal HPA-axis. In fact, PSA has predicted higher neonatal hair cortisol levels, while higher maternal hair cortisol levels in the first trimester of pregnancy had no association with PSA and predicted lower neonatal hair cortisol (Romero-Gonzalez et al., Citation2018). Keeping in mind that the amygdala is rich in glucocorticoid receptors and hence subject to its effects (Herman et al., Citation2005), it is intriguing that one study found PSA to be the most important psychological factor in explaining variance in methylation state of the promoter region of the human newborn glucocorticoid receptor gene NR3C1 (Hompes et al., Citation2013). Glucocorticoid receptor methylation is one possible mechanism by which PSA could influence the morphologically different nuclei of the amygdala (Schumann et al., Citation2011). However, the influence of PSA is most likely mediated through several mechanisms and is thus difficult to pinpoint to a specific individual objective mediating measure. A common feature in these studies is that outcomes vary depending on the subtype of distress.
Similarly to some previous studies focusing on PPD (not including PSA) (Lehtola et al., 2020; Qiu et al., Citation2013), the results of our current study did not reveal any association between PSA and newborn hippocampal volumes. However, other studies have observed smaller hippocampal volumes in fetuses and slower hippocampal growth during the first six months of age after exposure to prenatal general anxiety (Qiu et al., Citation2013; Wen et al., Citation2017). Further, the interplay of genetic variance and environmental factors appears to be associated with the development of hippocampal volumes in the offspring, as was observed in an Asian cohort, where a polygenic risk score for major depressive disorder moderated the association between PPD and the volume of the newborn right hippocampus (Qiu et al., Citation2017). Interestingly, a similar study with a European sample detected a sex-specific connection between PPD and right hippocampal volumes: male infants with a higher genetic risk for major depressive disorder had smaller volumes compared to their female counterparts, while males in the low genetic risk group had larger hippocampal volumes compared to females (Acosta et al., Citation2020).
Overall, evidence suggests that PSA/prenatal anxiety has significant long-term influences on offspring neurodevelopment, which may predispose the offspring to psychiatric disorders. Based on the sex-dependent neuroanatomical findings in individuals exposed to PPD, the susceptibility to later psychopathology may differ between sexes and may in part explain, for instance, the higher prevalence of certain disorders in males or females, such as depression, anxiety disorders, autism spectrum disorders, and attention deficit/hyperactivity disorders. However, one cannot merely deduct what the potential effects of PSA on these limbic structures could be, as the mechanisms, through which maternal distress is mediated to the fetus, are complex. Further, although anxiety has been associated with enlarged amygdalae and reduced hippocampal values in the human brain (Kolesar et al., Citation2019), this may not be applicable within the context of in-utero exposure to maternal anxiety – the effect could actually be reverse through adaptation. Thus, empirical studies are essential. When investigating the predisposition to psychopathology after exposure to PPD, it would be ideal to include the functional brain networks that connect with the amygdala (and hippocampus), as well as genetic factors, and postnatal events, especially if studying older individuals.
5. Strengths and limitations
This study has several strengths. Importantly, to the best of our knowledge, this is the first study to report results on the relationship of PSA with both amygdalar and hippocampal volumes in newborns. Studies concerning PSA and neuroanatomy in children are few, and in newborns, non-existent. Moreover, the sample size was relatively large. The young age of the study participants minimized the confounding effects of postnatal events with possible influences on amygdalar growth and development. The fact that both the measurement of PSA, as well as the acquisition of the MRI were performed only at one time point, is a limitation of this study, precluding the investigation of associations between PSA in other points of pregnancy and newborn brain volumes, as well as the observation of whether the significant association between amygdalar volumes in females persists later in early postnatal life. The investigation of gene-environment interactions, as well as different factors related to HPA-axis activity would be of major interest to better understand the underlying mechanisms. In addition, as the results did not survive after correction for multiple comparisons, they should be considered preliminary.
Acknowledgements
We thank our radiographer Krisse Kuvaja for performing the imaging, the FinnBrain staff and the participating families.
Disclosure statement
No potential conflict of interest was reported by the author(s).
Additional information
Funding
References
- Acosta, H., Kantojärvi, K., Hashempour, N., Pelto, J., Scheinin, N. M., Lehtola, S. J., Lewis, J. D., Fonov, V. S., Collins, D. L., Evans, A., Parkkola, R., Lähdesmäki, T., Saunavaara, J., Karlsson, L., Merisaari, H., Paunio, T., Karlsson, H., & Tuulari, J. J. (2020). Partial support for an interaction between a polygenic risk score for major depressive disorder and prenatal maternal depressive symptoms on infant right amygdalar volumes. Cerebral Cortex (Cortex), 30(12), 6121–6134. https://doi.org/10.1093/cercor/bhaa158
- Acosta, H., Tuulari, J. J., Scheinin, N. M., Hashempour, N., Rajasilta, O., Lavonius, T. I., Pelto, J., Saunavaara, V., Parkkola, R., Lähdesmäki, T., Karlsson, L., & Karlsson, H. (2019). Maternal pregnancy-related anxiety is associated with sexually dimorphic alterations in amygdala volume in 4-year-old children. Frontiers in Behavioral Neuroscience, 13, 175. https://doi.org/10.3389/fnbeh.2019.00175
- Adib-Rad, H., Basirat, Z., Faramarzi, M., Mostafazadeh, A., & Bijani, A. (2019). Psychological distress in women with recurrent spontaneous abortion: A case-control study. Turkish Journal of Obstetrics and Gynaecology, 16(3), 151–157. https://doi.org/10.4274/tjod.galenos.2019.88899
- Bayrampour, H., Ali, E., McNeil, D. A., Benzies, K., MacQueen, G., & Tough, S. (2016). Pregnancy-related anxiety: A concept analysis. International Journal of Nursing Studies, 55, 115–130. https://doi.org/10.1016/j.ijnurstu.2015.10.023
- Bech, P., Bille, J., Møller, S. B., Hellström, L. C., & Østergaard, S. D. (2014). Psychometric validation of the Hopkins Symptom Checklist (SCL-90) subscales for depression, anxiety, and interpersonal sensitivity. Journal of Affective Disorders, 160, 98–103. https://doi.org/10.1016/j.jad.2013.12.005
- Benjamini, Y., & Hochberg, Y. (1995). Controlling the false discovery rate: A practical and powerful approach to multiple testing. Journal of the Royal Statistical Society: Series B (Methodological)), 57(1), 289–300. https://doi.org/10.1111/j.2517-6161.1995.tb02031.x
- Bergh, B. V. d. (1990). The influence of maternal emotions during pregnancy on fetal and neonatal behavior. Journal of Prenatal & Perinatal Psychology & Health, 5, 119–130.
- Bergink, V., Kooistra, L., Lambregtse-van den Berg, M. P., Wijnen, H., Bunevicius, R., van Baar, A., & Pop, V. (2011). Validation of the Edinburgh Depression Scale during pregnancy. Journal of Psychosomatic Research, 70(4), 385–389. https://doi.org/10.1016/j.jpsychores.2010.07.008
- Blackmore, E. R., Gustafsson, H., Gilchrist, M., Wyman, C., & G O'Connor, T. (2016). Pregnancy-related anxiety: Evidence of distinct clinical significance from a prospective longitudinal study. Journal of Affective Disorders, 197, 251–258. https://doi.org/10.1016/j.jad.2016.03.008
- Brunton, R., Dryer, R., Saliba, A., & Kohlhoff, J. (2019). Re-examining pregnancy-related anxiety: A replication study. Women and Birth: Journal of the Australian College of Midwives, 32(1), e131–e137. https://doi.org/10.1016/j.wombi.2018.04.013
- Bunevicius, A., Kusminskas, L., Pop, V. J., Pedersen, C. A., & Bunevicius, R. (2009). Screening for antenatal depression with the Edinburgh Depression Scale. Journal of Psychosomatic Obstetrics & Gynecology, 30(4), 238–243. https://doi.org/10.3109/01674820903230708
- Buss, C., Davis, E. P., Hobel, C. J., & Sandman, C. A. (2011). Maternal pregnancy-specific anxiety is associated with child executive function at 6-9 years age. Stress, 14(6), 665–676. https://doi.org/10.3109/10253890.2011.623250
- Buss, C., Davis, E. P., Shahbaba, B., Pruessner, J. C., Head, K., & Sandman, C. A. (2012). Maternal cortisol over the course of pregnancy and subsequent child amygdala and hippocampus volumes and affective problems. Proceedings of the National Academy of Sciences of the United States of America, 109(20), E1312–E1319. https://doi.org/10.1073/pnas.1201295109
- Caputo, C., Wood, E., & Jabbour, L. (2016). Impact of fetal alcohol exposure on body systems: A systematic review. Birth Defects Research. Part C, Embryo Today: Reviews, 108(2), 174–180. https://doi.org/10.1002/bdrc.21129
- Charil, A., Laplante, D. P., Vaillancourt, C., & King, S. (2010). Prenatal stress and brain development. Brain Research Reviews, 65(1), 56–79. https://doi.org/10.1016/j.brainresrev.2010.06.002
- Clifton, V. L. (2010). Review: Sex and the human placenta: Mediating differential strategies of fetal growth and survival. Placenta, 31(SUPPL), S33–S39. https://doi.org/10.1016/j.placenta.2009.11.010
- Contu, L., & Hawkes, C. A. (2017). A review of the impact of maternal obesity on the cognitive function and mental health of the offspring. International Journal of Molecular Sciences, 18(5), 1093. https://doi.org/10.3390/ijms18051093
- Coupé, P., Manjón, J. V., Fonov, V., Pruessner, J., Robles, M., & Collins, D. L. (2011). Patch-based segmentation using expert priors: Application to hippocampus and ventricle segmentation. NeuroImage, 54(2), 940–954. https://doi.org/10.1016/j.neuroimage.2010.09.018
- Cox, J. L., Holden, J. M., & Sagovsky, R. (1987). Detection of postnatal depression. Development of the 10-item Edinburgh Postnatal Depression Scale . The British Journal of Psychiatry: The Journal of Mental Science, 150(JUNE), 782–786. https://doi.org/10.1192/bjp.150.6.782
- Dahlerup, B. R., Egsmose, E. L., Siersma, V., Mortensen, E. L., Hedegaard, M., Knudsen, L. E., & Mathiesen, L. (2018). Maternal stress and placental function, a study using questionnaires and biomarkers at birth. PLoS One, 13(11), e0207184. https://doi.org/10.1371/journal.pone.0207184
- Davis, E., & Sandman, C. (2012). Prenatal psychobiological predictors of anxiety risk in preadolescent children. Psychoneuroendocrinology, 37(8), 1224–1233. https://doi.org/10.1016/j.psyneuen.2011.12.016
- de Haan, M., Wyatt, J. S., Roth, S., Vargha-Khadem, F., Gadian, D., & Mishkin, M. (2006). Brain and cognitive-behavioural development after asphyxia at term birth. Developmental Science, 9(4), 350–358. https://doi.org/10.1111/j.1467-7687.2006.00499.x
- Deogratis, L. R., Lipman, R. S., & Covi, L. (1973). SCL-90: An outpatient psychiatric rating scale-preliminary report. Psychopharmacology Bulletin, 9(1), 13–28.
- Devarshi, P. P., Grant, R. W., Ikonte, C. J., & Hazels Mitmesser, S. (2019). Maternal omega-3 nutrition, placental transfer and fetal brain development in gestational diabetes and preeclampsia. Nutrients, 11(5), 1107. https://doi.org/10.3390/nu11051107
- Ehrlich, D. E., Neigh, G. N., Bourke, C. H., Nemeth, C. L., Hazra, R., Ryan, S. J., Rowson, S., Jairam, N., Sholar, C. A., Rainnie, D. G., Stowe, Z. N., & Owens, M. J. (2015). Prenatal stress, regardless of concurrent escitalopram treatment, alters behavior and amygdala gene expression of adolescent female rats. Neuropharmacology, 97, 251–258. https://doi.org/10.1016/j.neuropharm.2015.05.012
- Falster, K., Hanly, M., Banks, E., Lynch, J., Chambers, G., Brownell, M., Eades, S., & Jorm, L. (2018). Maternal age and offspring developmental vulnerability at age five: A population-based cohort study of Australian children. PLOS Medicine, 15(4), e1002558. https://doi.org/10.1371/journal.pmed.1002558
- Fonov, V., Evans, A. C., Botteron, K., Almli, C. R., McKinstry, R. C., Collins, D. L., & Brain Development Cooperative Group. (2011). Unbiased average age-appropriate atlases for pediatric studies. NeuroImage, 54(1), 313–327. https://doi.org/10.1016/j.neuroimage.2010.07.033
- Gingrich, J. A., Malm, H., Ansorge, M. S., Brown, A., Sourander, A., Suri, D., Teixeira, C. M., Caffrey Cagliostro, M. K., Mahadevia, D., & Weissman, M. M. (2017). New insights into how serotonin selective reuptake inhibitors shape the developing brain. Birth Defects Research, 109(12), 924–932. https://doi.org/10.1002/bdr2.1085
- Hashempour, N., Tuulari, J. J., Merisaari, H., Lidauer, K., Luukkonen, I., Saunavaara, J., Parkkola, R., Lähdesmäki, T., Lehtola, S. J., Keskinen, M., Lewis, J. D., Scheinin, N. M., Karlsson, L., & Karlsson, H. (2019). A novel approach for manual segmentation of the amygdala and hippocampus in neonate MRI. Frontiers in Neuroscience, 13, 1025. https://doi.org/10.3389/fnins.2019.01025
- Herman, J. P., Ostrander, M. M., Mueller, N. K., & Figueiredo, H. (2005). Limbic system mechanisms of stress regulation: Hypothalamo-pituitary-adrenocortical axis. Progress in Neuro-Psychopharmacology & Biological Psychiatry, 29(8), 1201–1213. https://doi.org/10.1016/j.pnpbp.2005.08.006
- Holland, D., Chang, L., Ernst, T. M., Curran, M., Buchthal, S. D., Alicata, D., Skranes, J., Johansen, H., Hernandez, A., Yamakawa, R., Kuperman, J. M., & Dale, A. M. (2014). Structural growth trajectories and rates of change in the first 3 months of infant brain development. JAMA Neurology, 71(10), 1266–1274. https://doi.org/10.1001/jamaneurol.2014.1638
- Hompes, T., Izzi, B., Gellens, E., Morreels, M., Fieuws, S., Pexsters, A., Schops, G., Dom, M., Van Bree, R., Freson, K., Verhaeghe, J., Spitz, B., Demyttenaere, K., Glover, V., Van den Bergh, B., Allegaert, K., & Claes, S. (2013). Investigating the influence of maternal cortisol and emotional state during pregnancy on the DNA methylation status of the glucocorticoid receptor gene (NR3C1) promoter region in cord blood. Journal of Psychiatric Research, 47(7), 880–891. https://doi.org/10.1016/j.jpsychires.2013.03.009
- Huizink, A., Mulder, E., & Buitelaar, J. (2004). Prenatal stress and risk for psychopathology: specific effects or induction of general susceptibility? Psychological Bulletin, 130(1), 115–142. https://doi.org/10.1037/0033-2909.130.1.115
- Huizink, A. C., Delforterie, M. J., Scheinin, N. M., Tolvanen, M., Karlsson, L., & Karlsson, H. (2016). Adaption of pregnancy anxiety questionnaire-revised for all pregnant women regardless of parity: PRAQ-R2. Archives of Women's Mental Health, 19(1), 125–132. https://doi.org/10.1007/s00737-015-0531-2
- Huizink, A. C., de Medina, P. G. R., Mulder, E. J. H., Visser, G. H. A., & Buitelaar, J. K. (2002). Psychological measures of prenatal stress as predictors of infant temperament. Journal of the American Academy of Child and Adolescent Psychiatry, 41(9), 1078–1085. https://doi.org/10.1097/00004583-200209000-00008
- Huizink, A. C., Menting, B., Oosterman, M., Verhage, M. L., Kunseler, F. C., & Schuengel, C. (2014). The interrelationship between pregnancy-specific anxiety and general anxiety across pregnancy: A longitudinal study. Journal of Psychosomatic Obstetrics and Gynaecology, 35(3), 92–100. https://doi.org/10.3109/0167482X.2014.944498
- Huizink, A. C., Mulder, E. J. H., Robles de Medina, P. G., Visser, G. H. A., & Buitelaar, J. K. (2004). Is pregnancy anxiety a distinctive syndrome? Early Human Development, 79(2), 81–91. https://doi.org/10.1016/j.earlhumdev.2004.04.014
- Kamel, H. S., Ahmed, H. N., Eissa, M. A., & Abol-Oyoun Al-S, M. (1999). Psychological and obstetrical responses of mothers following antenatal fetal sex identification. The Journal of Obstetrics and Gynaecology Research, 25(1), 43–50. https://doi.org/10.1111/j.1447-0756.1999.tb01121.x
- Karlsson, L., Tolvanen, M., Scheinin, N. M., Uusitupa, H.-M., Korja, R., Ekholm, E., Tuulari, J. J., Pajulo, M., Huotilainen, M., Paunio, T., & Karlsson, H., & FinnBrain Birth Cohort Study Group. (2018). Cohort profile: The FinnBrain Birth Cohort Study (FinnBrain). International Journal of Epidemiology, 47(1), 15–16j. https://doi.org/10.1093/ije/dyx173
- Knickmeyer, R. C., Xia, K., Lu, Z., Ahn, M., Jha, S. C., Zou, F., Zhu, H., Styner, M., & Gilmore, J. H. (2016). Impact of demographic and obstetric factors on infant brain volumes: A population neuroscience study. Cerebral Cortex, 5(Suppl 1), 187. https://doi.org/10.1093/cercor/bhw331
- Kolesar, T. A., Bilevicius, E., Wilson, A. D., & Kornelsen, J. (2019). Systematic review and meta-analyses of neural structural and functional differences in generalized anxiety disorder and healthy controls using magnetic resonance imaging. NeuroImage: Clinical, 24, 102016. https://doi.org/10.1016/j.nicl.2019.102016
- Kouwenhoven, E., Giezen, M., & Struikmans, H. (2009). Measuring the similarity of target volume delineations independent of the number of observers - IOPscience. Physics in Medicine and Biology, 54(9), 2863–2873. https://doi.org/10.1088/0031-9155/54/9/018
- Kramer, M. S., Lydon, J., Séguin, L., Goulet, L., Kahn, S. R., McNamara, H., Genest, J., Dassa, C., Chen, M. F., Sharma, S., Meaney, M. J., Thomson, S., Van Uum, S., Koren, G., Dahhou, M., Lamoureux, J., & Platt, R. W. (2009). Stress pathways to spontaneous preterm birth: The role of stressors, psychological distress, and stress hormones. American Journal of Epidemiology, 169(11), 1319–1326. https://doi.org/10.1093/aje/kwp061
- Kumpulainen, V., Lehtola, S. J., Tuulari, J. J., Silver, E., Copeland, A., Korja, R., Karlsson, H., Karlsson, L., Merisaari, H., Parkkola, R., Saunavaara, J., Lähdesmäki, T., & Scheinin, N. M. (2020). Prevalence and risk factors of incidental findings in Brain MRIs of healthy neonates—The FinnBrain Birth Cohort Study. Frontiers in Neurology, 10, 1347. https://doi.org/10.3389/fneur.2019.01347
- Lehtola, S. J., Tuulari, J. J., Karlsson, L., Parkkola, R., Merisaari, H., Saunavaara, J., Lähdesmäki, T., Scheinin, N. M., & Karlsson, H. (2019). Associations of age and sex with brain volumes and asymmetry in 2-5-week-old infants. Brain Structure & Function, 224(1), 501–513. https://doi.org/10.1007/s00429-018-1787-x
- Lehtola, S. J., Tuulari, J. J., Scheinin, N. M., Karlsson, L., Parkkola, R., Merisaari, H., Lewis, J. D., Fonov, V. S., Louis Collins, D., Evans, A., Saunavaara, J., Hashempour, N., Lähdesmäki, T., Acosta, H., & Karlsson, H. (2020). Newborn amygdalar volumes are associated with maternal prenatal psychological distress in a sex-dependent way. NeuroImage: Clinical, 28, 102380. https://doi.org/10.1016/j.nicl.2020.102380
- Lewis, J. D., Fonov, V. S., Collins, D. L., Evans, A. C., Tohka, J., & Brain Development Cooperative Group and the Pediatric Imaging, Neurocognition, and Genetics Study. (2019). Cortical and subcortical T1 white/gray contrast, chronological age, and cognitive performance. NeuroImage, 196, 276–288. https://doi.org/10.1016/j.neuroimage.2019.04.022
- Lin, Y., Hu, W., Xu, J., Luo, Z., Ye, X., Yan, C., Liu, Z., & Tong, S. (2017). Association between temperature and maternal stress during pregnancy. Environmental Research, 158, 421–430. https://doi.org/10.1016/j.envres.2017.06.034
- Little, B., Sud, N., Nobile, Z., & Bhattacharya, D. (2021). Teratogenic effects of maternal drug abuse on developing brain and underlying neurotransmitter mechanisms. Neurotoxicology, 86, 172–179. https://doi.org/10.1016/j.neuro.2021.08.007
- Mudra, S., Göbel, A., Barkmann, C., Goletzke, J., Hecher, K., Schulte-Markwort, M., Diemert, A., & Arck, P. (2020). The longitudinal course of pregnancy-related anxiety in parous and nulliparous women and its association with symptoms of social and generalized anxiety. Journal of Affective Disorder, 260, 111–118. https://doi.org/10.1016/j.jad.2019.08.033
- Mustonen, P., Karlsson, L., Kataja, E.-L., Scheinin, N. M., Kortesluoma, S., Coimbra, B., Rodrigues, A. J., Sousa, N., & Karlsson, H. (2019). Maternal prenatal hair cortisol is associated with prenatal depressive symptom trajectories. Psychoneuroendocrinology, 109, 104383. https://doi.org/10.1016/j.psyneuen.2019.104383
- Nolvi, S., Karlsson, L., Bridgett, D. J., Korja, R., Huizink, A. C., Kataja, E.-L., & Karlsson, H. (2016). Maternal prenatal stress and infant emotional reactivity six months postpartum. Journal of Affective Disorders, 199, 163–170. https://doi.org/10.1016/j.jad.2016.04.020
- O’Donnell, K. J., Bugge Jensen, A., Freeman, L., Khalife, N., O’Connor, T. G., & Glover, V. (2012). Maternal prenatal anxiety and downregulation of placental 11ß-HSD2. Psychoneuroendocrinology, 37(6), 818–826. https://doi.org/10.1016/j.psyneuen.2011.09.014
- Pesonen, A.-K., Lahti, M., Kuusinen, T., Tuovinen, S., Villa, P., Hämäläinen, E., Laivuori, H., Kajantie, E., & Räikkönen, K. (2016). Maternal prenatal positive affect, depressive and anxiety symptoms and birth outcomes: The PREDO study. PLoS One, 11(2), e0150058. https://doi.org/10.1371/journal.pone.0150058
- Qin, S., Young, C. B., Duan, X., Chen, T., Supekar, K., & Menon, V. (2014). Amygdala subregional structure and intrinsic functional connectivity predicts individual differences in anxiety during early childhood. Biological Psychiatry, 75(11), 892–900. https://doi.org/10.1016/j.biopsych.2013.10.006
- Qiu, A., Rifkin-Graboi, A., Chen, H., Chong, Y.-S., Kwek, K., Gluckman, P. D., Fortier, M. V., & Meaney, M. J. (2013). Maternal anxiety and infants' hippocampal development: Timing matters. Translational Psychiatry, 3(9), e306. https://doi.org/10.1038/tp.2013.79
- Qiu, A., Shen, M., Buss, C., Chong, Y.-S., Kwek, K., Saw, S.-M., Gluckman, P. D., Wadhwa, P. D., Entringer, S., Styner, M., Karnani, N., Heim, C. M., O'Donnell, K. J., Holbrook, J. D., Fortier, M. V., Meaney, M. J., & the GUSTO Study Group. (2017). Effects of antenatal maternal depressive symptoms and socio-economic status on neonatal brain development are modulated by genetic risk. Cerebral Cortex, 27(5), 3080–3092. https://doi.org/10.1093/cercor/bhx065
- Richter-Levin, G. (2004). The amygdala, the hippocampus, and emotional modulation of memory. The Neuroscientist : A Review Journal Bringing Neurobiology, Neurology and Psychiatry, 10(1), 31–39. https://doi.org/10.1177/1073858403259955
- Rifkin-Graboi, A., Bai, J., Chen, H., Hameed, W. B., Sim, L. W., Tint, M. T., Leutscher-Broekman, B., Chong, Y.-S., Gluckman, P. D., Fortier, M. V., Meaney, M. J., & Qiu, A. (2013). Prenatal maternal depression associates with microstructure of right amygdala in neonates at birth. Biological Psychiatry, 74(11), 837–844. https://doi.org/10.1016/j.biopsych.2013.06.019
- Romero-Gonzalez, B., Caparros-Gonzalez, R. A., Gonzalez-Perez, R., Delgado-Puertas, P., & Peralta-Ramirez, M. I. (2018). Newborn infants’ hair cortisol levels reflect chronic maternal stress during pregnancy. PLoS One, 13(7), e0200279. https://doi.org/10.1371/journal.pone.0200279
- Rooks, V. J., Eaton, J. P., Ruess, L., Petermann, G. W., Keck-Wherley, J., & Pedersen, R. C. (2008). Prevalence and evolution of intracranial hemorrhage in asymptomatic term Infants. American Journal of Neuroradiology, 29(6), 1082–1089. https://doi.org/10.3174/ajnr.A1004
- Rotem-Kohavi, N., & Oberlander, T. F. (2017). Variations in neurodevelopmental outcomes in children with prenatal SSRI antidepressant exposure. Birth Defects Research, 109(12), 909–923. https://doi.org/10.1002/bdr2.1076
- Rubertsson, C., Börjesson, K., Berglund, A., Josefsson, A., & Sydsjö, G. (2011). The Swedish validation of Edinburgh Postnatal Depression Scale (EPDS) during pregnancy. Nordic Journal of Psychiatry, 65(6), 414–418. https://doi.org/10.3109/08039488.2011.590606
- Sandin, S. (2012). Advancing maternal age is associated with increasing risk for autism: A review and meta-analysis. Journal of the American Academy of Child and Adolescent Psychiatry, 51(5), 477–486.E1. https://doi.org/10.1016/j.jaac.2012.02.018
- Schumann, C. M., Bauman, M. D., & Amaral, D. G. (2011). Abnormal structure or function of the amygdala is a common component of neurodevelopmental disorders. Neuropsychologia, 49(4), 745–759. https://doi.org/10.1016/j.neuropsychologia.2010.09.028
- Shin, L. M., & Liberzon, I. (2010). The neurocircuitry of fear, stress, and anxiety disorders. Neuropsychopharmacology: Neuropsychopharmacology, 35(1), 169–191. https://doi.org/10.1038/npp.2009.83
- Szekely, E., Neumann, A., Sallis, H., Jolicoeur-Martineau, A., Verhulst, F. C., Meaney, M. J., Pearson, R. M., Levitan, R. D., Kennedy, J. L., Lydon, J. E., Steiner, M., Greenwood, C. M. T., Tiemeier, H., Evans, J., & Wazana, A. (2021). Maternal prenatal mood, pregnancy-specific worries, and early child psychopathology: Findings from the DREAM BIG Consortium. Journal of the American Academy of Child and Adolescent Psychiatry, 60(1), 186–197. https://doi.org/10.1016/j.jaac.2020.02.017
- Tendais, I., Costa, R., Conde, A., & Figueiredo, B. (2014). Screening for depression and anxiety disorders from pregnancy to postpartum with the EPDS and STAI. The Spanish Journal of Psychology, 17, E7. https://doi.org/10.1017/sjp.2014.7
- Theut, S. K., Pedersen, F. A., Zaslow, M. J., & Rabinovich, B. A. (1988). Pregnancy subsequent to perinatal loss: Parental anxiety and depression. Journal of the American Academy of Child and Adolescent Psychiatry, 27(3), 289–292. https://doi.org/10.1097/00004583-198805000-00004
- Van den Bergh, B. R. H., Dahnke, R., & Mennes, M. (2018). Prenatal stress and the developing brain: Risks for neurodevelopmental disorders. Development and Psychopathology, 30(3), 743–762. https://doi.org/10.1017/S0954579418000342
- Van den Bergh, B. R. H., Mulder, E. J. H., Mennes, M., & Glover, V. (2005). Antenatal maternal anxiety and stress and the neurobehavioural development of the fetus and child: Links and possible mechanisms. A review. Neuroscience and Biobehavioral Reviews, 29(2), 237–258. https://doi.org/10.1016/j.neubiorev.2004.10.007
- Van den Bergh, B. R. H., Van Calster, B., Smits, T., Van Huffel, S., & Lagae, L. (2008). Antenatal maternal anxiety is related to HPA-axis dysregulation and self-reported depressive symptoms in adolescence: A prospective study on the fetal origins of depressed mood. Neuropsychopharmacology: Official Publication of the American College of Neuropsychopharmacology, 33(3), 536–545. https://doi.org/10.1038/sj.npp.1301450
- van den Heuvel, M. I., Donkers, F. C. L., Winkler, I., Otte, R. A., & Van den Bergh, B. R. H. (2015). Maternal mindfulness and anxiety during pregnancy affect infants’ neural responses to sounds. Social Cognitive and Affective Neuroscience, 10(3), 453–460. https://doi.org/10.1093/scan/nsu075
- van den Heuvel, M. I., van Assen, M. A. L. M., Glover, V., Claes, S., & Van den Bergh, B. R. H. (2018). Associations between maternal psychological distress and salivary cortisol during pregnancy: A mixed-models approach. Psychoneuroendocrinology, 96, 52–60. https://doi.org/10.1016/j.psyneuen.2018.06.005
- Van Lieshout, R. J., Taylor, V. H., & Boyle, M. H. (2011). Pre-pregnancy and pregnancy obesity and neurodevelopmental outcomes in offspring: A systematic review. Obesity Reviews: Reviews, 12(5), e548–e559. https://doi.org/10.1111/j.1467-789X.2010.00850.x
- van Soelen, I. L. C., Brouwer, R. M., Peper, J. S., van Beijsterveldt, T. C. E. M., van Leeuwen, M., de Vries, L. S., Kahn, R. S., Hulshoff Pol, H. E., & Boomsma, D. I. (2010). Effects of gestational age and birth weight on brain volumes in healthy 9 year-old children. The Journal of Pediatrics, 156(6), 896–901. https://doi.org/10.1016/j.jpeds.2009.12.052
- Visser, M., Müller, D. M. J., van Duijn, R. J. M., Smits, M., Verburg, N., Hendriks, E. J., Nabuurs, R. J. A., Bot, J. C. J., Eijgelaar, R. S., Witte, M., van Herk, M. B., Barkhof, F., de Witt Hamer, P. C., & de Munck, J. C. (2019). Inter-rater agreement in glioma segmentations on longitudinal MRI. NeuroImage: Clinical, 22, 101727. https://doi.org/10.1016/j.nicl.2019.101727
- Vlenterie, R., Geuijen, P. M., van Gelder, M. M. H. J., & Roeleveld, N. (2021). Questionnaires and salivary cortisol to measure stress and depression in mid-pregnancy. PLoS One, 16(4), e0250459. https://doi.org/10.1371/journal.pone.0250459
- Wadhwa, P. D., Sandman, C. A., Porto, M., Dunkel-Schetter, C., & Garite, T. J. (1993). The association between prenatal stress and infant birth weight and gestational age at birth: A prospective investigation. American Journal of Obstetrics and Gynecology, 169(4), 858–865. https://doi.org/10.1016/0002-9378(93)90016-C
- Ward, J. H., Jr. (1963). Hierarchical grouping to optimize an objective function. Journal of the American Statistical Association, 58(301), 236–244. https://doi.org/10.1080/01621459.1963.10500845
- Weier, K., Fonov, V., Lavoie, K., Doyon, J., & Collins, D. L. (2014). Rapid automatic segmentation of the human cerebellum and its lobules (RASCAL)-Implementation and application of the patch-based label-fusion technique with a template library to segment the human cerebellum. Human Brain Mapping, 35(10), 5026–5039. https://doi.org/10.1002/hbm.22529
- Weinstock, M. (2011). Sex-dependent changes induced by prenatal stress in cortical and hippocampal morphology and behaviour in rats: An update. Stress, 14(6), 604–613. https://doi.org/10.3109/10253890.2011.588294
- Wen, D. J., Poh, J. S., Ni, S. N., Chong, Y.-S., Chen, H., Kwek, K., Shek, L. P., Gluckman, P. D., Fortier, M. V., Meaney, M. J., & Qiu, A. (2017). Influences of prenatal and postnatal maternal depression on amygdala volume and microstructure in young children. Translational Psychiatry, 7(4), e1103. https://doi.org/10.1038/tp.2017.74
- Whitby, E. H., Griffiths, P. D., Rutter, S., Smith, M. F., Sprigg, A., Ohadike, P., Davies, N. P., Rigby, A. S., & Paley, M. N. (2004). Frequency and natural history of subdural haemorrhages in babies and relation to obstetric factors. The Lancet, 363(9412), 846–851. https://doi.org/10.1016/S0140-6736(04)15730-9
- Wortinger, L. A., Engen, K., Barth, C., Andreassen, O. A., Nordbø Jørgensen, K., & Agartz, I. (2020). Asphyxia at birth affects brain structure in patients on the schizophrenia-bipolar disorder spectrum and healthy participants. Psychological Medicine, 1–10. https://doi.org/10.1017/S0033291720002779
- Yang, R. J., Mozhui, K., Karlsson, R.-M., Cameron, H. A., Williams, R. W., & Holmes, A. (2008). Variation in mouse basolateral amygdala volume is associated with differences in stress reactivity and fear learning. Neuropsychopharmacology: Official Publication of the American College of Neuropsychopharmacology, 33(11), 2595–2604. https://doi.org/10.1038/sj.npp.1301665
- Zhu, P., Tao, F., Hao, J., Sun, Y., & Jiang, X. (2010). Prenatal life events stress: implications for preterm birth and infant birthweight. American Journal of Obstetrics and Gynecology, 203(1), 34.e1–34.e8. https://doi.org/10.1016/j.ajog.2010.02.023