Abstract
The heart is the main organ of the circulatory system and requires fatty acids to maintain its activity. Stress is a contributor to aggravating cardiovascular diseases and even death, and exacerbates the abnormal lipid metabolism. The cardiac metabolism may be disturbed by stress. Cholecystokinin (CCK), which is a classical peptide hormone, and its receptor (CCKR) are expressed in myocardial cells and affect cardiovascular function. Nevertheless, under stress, the exact role of CCKR on cardiac function and cardiac metabolism is unknown and the mechanism is worth exploring. After unpredictable stress, a common stress-inducing model that induces the development of mood disorders such as anxiety and reduces motivated behavior, we found that the abnormal contraction and diastole of the heart, myocardial injury, oxidative stress and inflammation of mice were aggravated. Cholecystokinin A receptor and cholecystokinin B receptor knockout (CCK1R2R-/-) significantly reversed these changes. Mechanistically, fatty acid metabolism was found to be altered in CCK1R2R-/- mice. Differential metabolites, especially L-tryptophan, L-aspartic acid, cholesterol, taurocholic acid, ADP, oxoglutaric acid, arachidonic acid and 17-Hydroxyprogesterone, influenced cardiac function after CCK1R2R knockout and unpredictable stress. We conclude that CCK1R2R-/- ameliorated myocardial damage caused by unpredictable stress via altering fatty acid metabolism.
1. Introduction
Stress broadly refers to the body’s response to environmental challenges and is caused by a stimulus (stressor) that produces the stress perception in the brain and, in turn, activates the physiologic stress response in the body (Hinterdobler et al., Citation2021). The two systems in the brain and body, including the hypothalamus pituitary adrenal axis and sympathetic adrenomedullary system, are triggered by stress. Commonly, after stress exposure, neural stress reactions occur immediately. The hypothalamus stimulates sympathetic nuclei in the medulla, and then the epinephrine (EPI) and noradrenaline (NA) are released from the adrenal medulla. Pulse, heart rate, blood pressure, electrodermal activity and other reactions within the sympathetic nervous system will be increased due to the release of EPI and NA (Starcke & Brand, Citation2012). Stress is a factor that aggravates cardiovascular diseases, including myocardial infarction, atherosclerosis and stroke (Kuebler et al., Citation2015). More seriously, sudden deaths due to cardiac causes can result in more common manifestations of stress such as anger or emotional upset (Sara et al., Citation2021). Despite the enormous impact of stress on human health, exactly how it influences cardiac function remains unclear, and it is meaningful to investigate the harmful mechanism of stress.
Cholecystokinin (CCK) is a typical peptide hormone and has been found in small intestinal mucosa cells, immune cells and neurons (Saia et al., Citation2013). Functionally, it plays a regulatory role in satiety, nociception and anxiety through cholecystokinin A receptor (CCK1R) and cholecystokinin B receptor (CCK2R) (Kurosawa et al., Citation2001). Recently, CCK and its receptors have been reported to be expressed in mammalian cardiomyocytes (Han et al., Citation2022). CCK regulates heart rate, increases cardiac contractility (Zhao et al., Citation2005), and has an effect on sympathetic vasomotor function and cardiovascular responses, which is mediated by CCKR (Sartor & Verberne, Citation2002). However, under stress, the role of CCKR remains to be revealed.
The heart has a huge adenosine triphosphate (ATP) demand to sustain ionic homeostasis, contractile function and basal metabolic processes. In normal conditions, 40–60% of ATP acquisition is dependent on fatty acids (Karwi et al., Citation2018), and in cardiomyocytes, 95% of ATP is produced by mitochondrial oxidative phosphorylation (Yamamoto & Sano, Citation2022). Changes in fatty acid metabolism impact the structure and function of the heart, and even cause adverse consequences, including induction of cardiac function damage, heart failure and other cardiovascular diseases (Hu et al., Citation2022). CCK has the ability to alter fatty acid metabolism and fatty acid sensing in the hypothalamus and hindbrain (Velasco et al., Citation2019). CCKR agonists amended adipose tissue function or fatty acid metabolism via mediating hunger and satiety signals (Jensen, Citation2006). However, the study about effects on cardiac function and mechanistic fatty acid metabolism changes after CCKR knockout and unpredictable stress have not been reported. Our study was committed to exploring the changes in cardiac function and differential metabolites to reveal the influence of CCKR knockout and unpredictable stress on the heart of mice.
2. Methods
Please check the Supplemental online material for details.
3. Results
3.1. Unpredictable stress induced heart dysfunction
As shown in , the movement distance in the central zone of wild-type mice stimulated by unpredictable stress (WT + ST) mice was decreased in comparison with wild-type (WT) mice (P < 0.01). It demonstrated that the stress model was successfully built and the stress caused anxiety-like behavior in mice. By observing the electrocardiogram of mice, significant changes in heart rate were seen (). The heart rate of WT + ST mice was increased, compared with the WT mice. It indicated that stress caused the presence of tachycardia. The results of displayed that the left ventricular systolic pressure (LVSP) value fell significantly in the WT + ST mice in contrast to the WT mice, and the left ventricular end-diastolic pressure (LVEDP) was increased in mice with stress in comparison with WT mice. The WT + ST mice showed lower maximal left ventricular pressure rising/drop rate (±dp/dtmax) than WT mice (0.01). These changes indicated that the contractile function and diastolic function of the heart were abnormal after stress. Stress increased EPI (P < 0.05) though barely changed NA in mouse serum (). The content of cardiac troponin-I (cTnI), creatine kinase-MB (CK-MB) and lactate dehydrogenase (LDH) (P < 0.01) was enhanced in WT + ST mice, compared with WT mice (). It suggested that myocardial injury occurred in mice after stress.
Figure 1. Unpredictable stress-induced heart dysfunction.
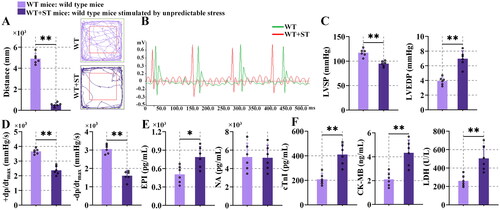
3.2. Unpredictable stress caused inflammation and apoptosis
The results of the enzyme-linked immune sorbent assay demonstrated that stress increased the tumor necrosis factor-alpha (TNF-α) and interleukin-6 (IL-6) levels (P < 0.01), compared with WT mice. No difference in the level of IL-10 was observed (). It seemed that stress increased the inflammation of mice. As shown in , the apoptosis was higher in WT + ST mice compared with WT mice. All these data revealed that stress-induced inflammation and apoptosis in the heart.
Figure 2. Unpredictable stress causes inflammation and apoptosis.
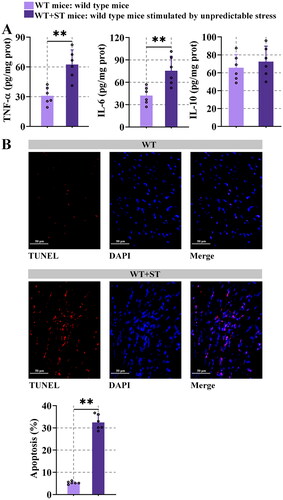
3.3. CCK and CCKR were upregulated after unpredictable stress
The expression of CCK1R, CCK2R and CCK content were prominently increased in WT + ST mice (, P < 0.01) than WT mice, suggesting the incremental production of CCK and CCKR after stress. However, their function during stress was unknown and was worth studying.
Figure 3. CCK and CCKR were upregulated after unpredictable stress.
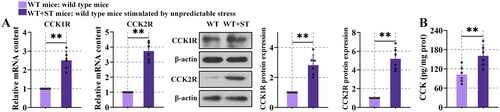
3.4. Cardiac function and myocardial injury were ameliorated in cholecystokinin a receptor and cholecystokinin B receptor knockout (CCK1R2R-/-) mice
Stress significantly reduced the movement distance in comparison with the WT mice or CCK1R2R-/- mice (, P < 0.01). Under stress, CCK1R2R-/- mice travelled more distance than WT mice (, P < 0.01). It seemed that CCK1R2R-/- might reduce anxiety levels. The heart rate was increased and R amplitude was decreased in WT + ST mice, in contrast to WT mice. Compared with CCK1R2R-/- mice, heart rate and R amplitude were enhanced in CCK1R2R-/- mice stimulated by unpredictable stress (CCK1R2R-/-+ST mice). The heart rate of CCK1R2R-/- mice was decreased, in comparison with WT mice. Increased R amplitude was shown in CCK1R2R-/-+ST mice rather than WT + ST mice (). The LVSP was decreased and LVEDP was increased in WT mice after stress. Higher LVEDP was observed in CCK1R2R-/-+ST mice than CCK1R2R-/- mice. Under stress, reduced LVEDP was displayed in the CCK1R2R-/- mice rather than WT mice (, P < 0.01). The ± dp/dtmax was decreased in WT + ST mice or CCK1R2R-/-+ST mice, compared with stress-unstimulated mice (, P < 0.01). These results suggested that CCK1R2R-/- might contribute to the amelioration of systolic and diastolic dysfunction of the heart. As shown in , the levels of cTnI, CK-MB and LDH in WT + ST mice were significantly higher than those in WT mice (P < 0.01). Similarly, CCK1R2R-/-+ST mice showed higher cTnI, CK-MB and LDH values than CCK1R2R-/- mice. Compared with WT + ST mice, CCK1R2R-/-+ST mice appeared to downregulate cTnI, CK-MB and LDH levels (, P < 0.05). In addition, terminal deoxynucleotidyl transferase-mediated dUTP-biotin nick end labelling staining was used for cell apoptosis detection. The apoptosis was further enlarged after stress in comparison with WT mice. CCK1R2R-/-+ST mice possessed remarkably upregulated apoptosis in contrast to CCK1R2R-/- mice. Under stress, the apoptosis was significantly downregulated in CCK1R2R-/- mice rather than WT mice (, P < 0.01). Our results implied that the existence of stress and CCK1R and CCK2R were conducive to myocardial injury and apoptosis.
Figure 4. Cardiac function and myocardial injury were ameliorated in CCK1R2R-/- mice.
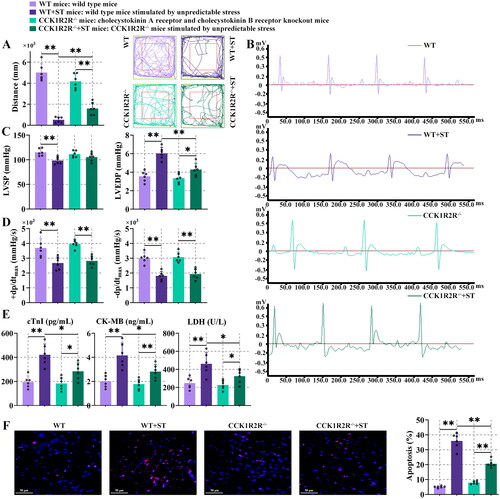
3.5. Oxidative stress and inflammation were decreased in CCK1R2R-/- mice
As illustrated in , fluorescence of reactive oxygen species (ROS) was higher after stress, compared with WT mice or CCK1R2R-/- mice. In contrast to WT + ST mice, the reduced fluorescence intensity of ROS was shown in CCK1R2R-/-+ST mice. The protein expression of heme oxygenase-1 (HO-1) and nuclear factor erythroid-2 related factor 2 (Nrf2) in the nucleus was decreased in WT + ST mice in comparison with WT or CCK1R2R-/-+ST mice. CCK1R2R-/- mice exhibited enhanced HO-1 and nucleus Nrf2 expression compared with CCK1R2R-/-+ST mice (, P < 0.05). These results demonstrated that CCK1R2R-/- inhibited the stress-induced oxidative stress.
Figure 5. Oxidative stress and inflammation were decreased in CCK1R2R-/- mice.
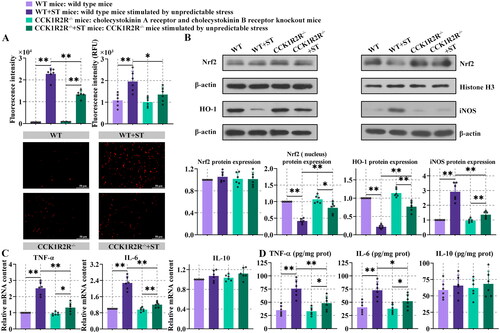
The mRNA expression of TNF-α and IL-6 was higher in WT + ST mice than WT or CCK1R2R-/-+ST mice (, P < 0.01). Higher content of TNF-α and IL-6 was observed in myocardial tissue of stress-stimulated WT mice than WT mice (, P < 0.01). In the presence of stress, compared with WT mice, the reduced TNF-α and IL-6 content was found in CCK1R2R-/- mice (, P < 0.05). No differences in IL-10 expression and content were found between different kinds of mice. After CCK1R2R knockout, stress caused increased mRNA expression and content of TNF-α and IL-6 (, P < 0.05). The expression of inducible nitric oxide synthase (iNOS) was increased in WT + ST mice compared with WT or CCK1R2R-/-+ST mice. In comparison with CCK1R2R-/-+ST, decreased iNOS expression was shown in CCK1R2R-/- mice (, P < 0.01), although there was no significant difference. These results indicated that inflammation was decreased in mice with CCK1R2R knockout.
3.6. Effects of CCK1R2R-/- on the metabolome
As shown in , a total of 497 metabolites were identified after matching with various databases. When compared with the WT, a similar pattern of metabolites were found in CCK1R2R-/- mice, but WT + ST exhibited a difference. Interestingly, a difference was shown between CCK1R2R-/- mice and CCK1R2R-/-+ST mice. The amount of specific differential metabolites between WT and WT + ST, between WT and CCK1R2R-/-, between CCK1R2R-/- and CCK1R2R-/-+ST, between WT + ST and CCK1R2R-/-+ST was 17, 9, 61 and 28, respectively (). Between WT and WT + ST, 48 differential metabolites were found, of which 34 metabolites were down-regulated and 14 metabolites were up-regulated in WT mice. Compared with CCK1R2R knockout mice, 20 metabolites were down-regulated and 18 metabolites were up-regulated in WT mice. Thirty-seven metabolites were down-regulated and sixty-eight metabolites were up-regulated in CCK1R2R-/- rather than CCK1R2R-/-+ST. WT + ST group showed 27 down-regulated metabolites and 33 up-regulated metabolites in comparison with CCK1R2R-/-+ST ( and ).
Figure 6. Overall identification of differential metabolites.
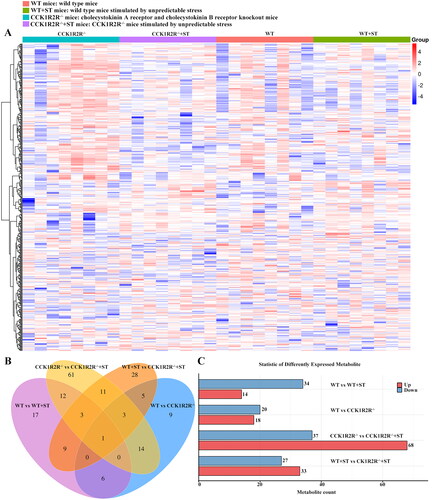
Figure 7. Analysis of differential metabolites between different groups.
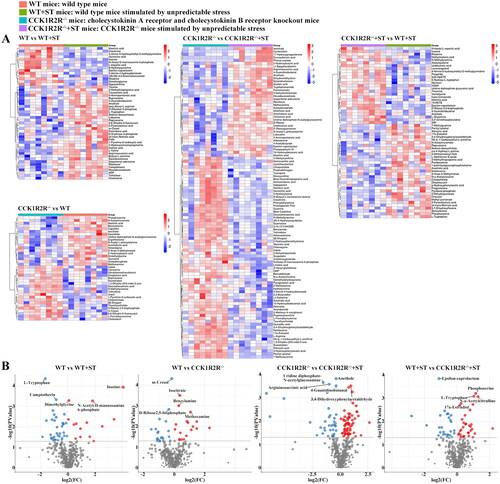
The significantly differential expression of L-Tryptophan, camptothecin, dimethylglycine, N-acetyl-D-mannosamine6-phosghate and inosine was found between WT and WT + ST. Isocitrate, benzylamine and methoxamine were markedly up-regulated, and m-cresol and D-ribose2,5-bisphosphate were signally down-regulated in WT rather than CCK1R2R-/-. CCK1R2R-/- group showed remarkable downregulation of uridine diphosphate-N-acetylglucosamine, anethole, argininosuccinic acid and 4-guanidinobutanal, and upregulation of 3,4-dihydroxyphenylacetaldehyde, compared with CCK1R2R-/-+ST. Phosphoserine, L-Tryptophan, N-a-acetylcitrulline and 17a-estradiol expression was upregulated, and epsilon-caprolactam expression was downregulated in WT + ST in comparison with CCK1R2R-/-+ST ( & Supplemental Table 1–4).
As shown in , the content of 8-Hydroxyquinoline, inosine, L-aspartic acid, (3S,5S)-3,5-diaminohexanoate, maslinic acid, and epsilon-caprolactam was higher in WT, compared with WT + ST. More ascorbate and maslinic acid were found in WT rather than CCK1R2R-/-. N-Acetyl-D-mannosamine 6-phosphate, ciliatocholate, N-methyltyramine, deoxyuridine, 17a-estradiol and ketoleucine level was more in CCK1R2R-/-, in comparison with CCK1R2R-/-+ST (). WT + ST showed higher content of pyridoxamine, ciliatocholate, chavicol, phosphoserine, 2-pyrocatechuic acid and 2-dehydropantoate than CCK1R2R-/-+ST.
Figure 8. The relative amounts of metabolites (WT vs WT + ST; WT vs CCK1R2R-/-) is shown in Z-score plot.
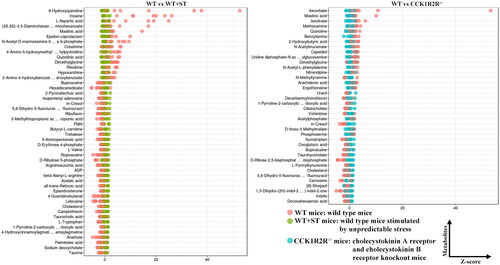
Figure 9. The relative amounts of metabolites (CCK1R2R-/- vs CCK1R2R-/-+ST; WT + ST vs CCK1R2R-/-+ST) is shown in Z-score plot.
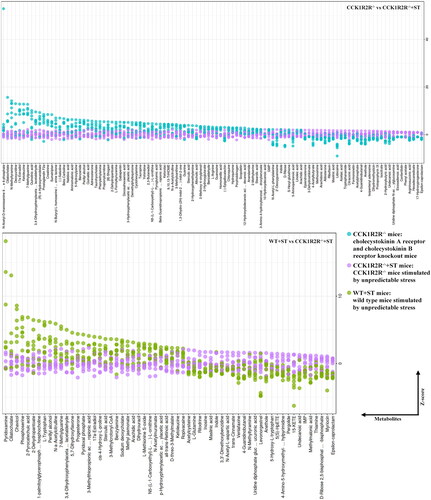
The differential metabolites between WT and WT + ST enriched in riboflavin metabolism, ABC transporters, arginine and proline metabolism, cholesterol metabolism, beta-alanine metabolism, basal cell carcinoma, central carbon metabolism in cancer, vitamin digestion and absorption, oxidative phosphorylation, the intestinal immune network for IgA production, protein digestion and absorption, primary bile acid biosynthesis, glycine, serine and threonine metabolism, aminoacyl-tRNA biosynthesis, neuroactive ligand-receptor interaction, taurine and hypotaurine metabolism, arginine biosynthesis, and small cell lung cancer pathways. Among them, the basal cell carcinoma pathway was affected enormously by cholesterol (Impact = 1.0). Most differential metabolites were associated with ABC transporters pathway (). Basal cell carcinoma, HIF-1 signaling pathway, histidine metabolism, citrate cycle (TCA cycle), aldosterone synthesis and secretion, taurine and hypotaurine metabolism, ovarian steroidogenesis, and glucagon signaling pathway were pathways that enriched by differential metabolites between WT and CCK1R2R-/-. Interestingly, oxoglutaric acid was associated with six pathways (). As shown in , the differential metabolites of CCK1R2R-/- and CCK1R2R-/-+ST markedly impacted the arginine biosynthesis and ovarian steroidogenesis pathways. Arachidonic acid participated in seven pathways (). Vitamin B6 metabolism, thiamin metabolism, vitamin digestion and absorption, intestinal immune network for IgA production, valine, leucine and isoleucine degradation, and serotonergic synapse pathways were observably enriched by differential metabolites of WT + ST and CCK1R2R-/-+ST. All-trans-retinoic acid played a significant role in the intestinal immune network for IgA production pathway (Impact = 0.5) (). All data indicated that the metabolites and their affected pathways were changed by stress or CCK1R2R knockout.
Figure 10. Differential metabolite pathway analysis (WT vs WT + ST; WT vs CCK1R2R-/-).
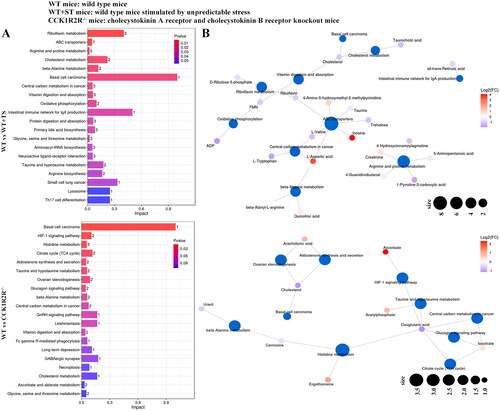
Figure 11. Differential metabolite pathway analysis (CCK1R2R-/- vs CCK1R2R-/-+ST; WT + ST vs CCK1R2R-/-+ST).
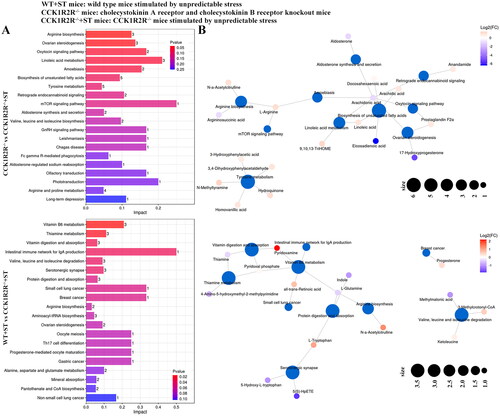
4. Discussion
The cardiovascular system is a target of stress along with the brain, metabolic system and immune system. Unpredictable stress ultimately affects heart function by activating certain regions in the brain and following responses from the sympathetic nervous system and hypothalamic-pituitary-adrenal axis (Hasan et al., Citation2011). Increased heart rate and EPI after stress stimulation in our study suggested that the unpredictable stress activated the sympathetic nervous system and hypothalamic–pituitary–adrenal axis. The reduced distance also indicated stress-induced motor dysfunction. The abnormal levels of left ventricular pressure and rate, increased cardiac enzymes related to myocardial infarction, enhanced inflammatory factor, and anabatic apoptosis revealed stress-induced myocardial injury. The risk of adverse cardiovascular events and psychiatric disorders was increased by stress (Das et al., Citation2022). Following stress, our study demonstrated that WT mice exhibited decreased cardiac function, and increased levels of CCK and CCKR, interestingly. The role of CCK and CCKR in cardiac function was worth studying.
In the open-field test, animals with increased CCK showed less locomotion in the centre zone (Wu et al., Citation2022). In addition to previous studies, we observed that compared with WT + ST mice, the CCK1R2R-/-+ST mice showed increased independent exploration ability, and it implied that CCK1R2R knockout weakened the stress-induced negative influence on the nerve. In addition, CCK affected cell apoptosis (Lavine et al., Citation2015), and CCK1R2R activated various kinases that are involved in cell proliferation, differentiation and apoptosis (Zeng et al., Citation2020). In this work, we showed that the absence of CCK1R2R ameliorated the cardiac dysfunction and cardiac cell apoptosis caused by stress.
Oxidative stress is characterized by excess production of ROS, and participates in the progression of cardiac dysfunction. Excess ROS leads to DNA damage, protein and lipid peroxidation, and cellular dysfunction, eventually causing cell damage and death (van der Pol et al., Citation2019). Under stress, oxidative stress was reduced in CCK1R2R-/- mice. The cellular dysfunction, caused by excess ROS, was improved by CCK1R2R knockout. On the other hand, nrf2 is involved in the antioxidative defense system by upregulating the antioxidant enzyme expression. After activation, Nrf2 is translocated to the nucleus and then facilitates the expression of antioxidant genes and enzymes (such as HO-1) (Chen et al., Citation2021). Upregulation of Nrf2/HO-1 pathway inhibited oxidative stress, thereby ameliorating cardiac dysfunction (Wu et al., Citation2021). CCK1R2R knockout reduced myocardial oxidative damage, which was induced by stress, through Nrf2/HO-1 pathway. Stress (Westfall et al., Citation2021), and oxidative stress cause inflammation, accordingly aggravating myocardial tissue damage and contributing to heart failure progression (Aimo et al., Citation2020). Reduced proinflammatory cytokine expression contributed to the decreased cardiovascular reactivity to stress (Wang et al., Citation2016). As a crucial mediator of immune activation and inflammation, inhibition of iNOS synthase halved the inflammatory response (Cevik-Aras and Ekstrom, Citation2010). CCKR antagonist improve chronic pancreatitis by reversing inflammation (Nadella et al., Citation2020). Our results proposed that the stress-caused inflammation was decreased by CCK1R2R knockout.
Metabonomics, as an important part of system biology, is widely used in the study of cardiovascular diseases in order to more comprehensively understand the pathophysiological processes. In our study, various differential metabolites between WT, WT + ST, CCK1R2R-/- and CCK1R2R-/-+ST mice were found, and these differential metabolites may reveal the reasons for different performance after unpredictable stress and CCK1R2R knockout. In the fetal serum, a raised level of taurocholic acid caused fetal dysrhythmia development (Williamson et al., Citation2001). Increased cholesterol content was observed in cardiomyopathic hamsters, and palmitoleic acid was positively associated with heart failure risk (Djoussé et al., Citation2012). These findings were in conformity with our study that the taurocholic acid, cholesterol and palmitoleic acid were enhanced in WT + ST mice, compared with WT mice. Patients with myocardial infarction showed higher dimethylglycine levels (Wei & Wang, Citation2021). Increased uracil during heart failure protected against myocardial damage, and carnosine played a beneficial role in diseased myocardial models (Creighton et al., Citation2022). Cardiac hypertrophy and dysfunction occur with elevated levels of arachidonic acid (Toko et al., Citation2020). CCK1R2R knockout decreased dimethylglycine and arachidonic acid content, and enhanced uracil and carnosine, compared with WT mice. Aldosterone, which exacerbated inflammation and myocardial hypertrophy at the cardiac level (Buffolo et al., Citation2022), was increased in CCK1R2R-/- mice after stress, and on the contrary, the antidepressant phenylethylamine decreased after stress. In comparison with CCK1R2R-/-+ST mice, increased L-arginine, which contributed to improving the cardiac function of rats with myocardial infarction (MacCarter et al., Citation2009), appeared in CCK1R2R-/- mice. Similarly, higher linoleic acid was detected in mice with CCK1R2R knockout, and linoleic acid provided protection during myocardial infarction (Qipshidze-Kelm et al., Citation2013). D-ribose improved ischemia-induced cardiac dysfunction (MacCarter et al., Citation2009) and the increased level of d-ribose may be a self-regulation of CCK1R2R-/- mice under stress. Anethole diet ameliorated dyslipidemia and decreased chronic heart disease risk of rats fed with high-fat diet (Noreen et al., Citation2023). Loss of thiamin caused neurological abnormalities and heart failure (DiNicolantonio et al., Citation2018). L-glutamine was beneficial in patients with heart failure by demonstrating anti-inflammatory and antioxidant capacities (Durante, Citation2019). Under stress, the increased anethole, thiamin and L-glutamine were found in mice with CCK1R2R knockout than WT mice, and they could contribute to the improvement of cardiac dysfunction. Creatinine level was reduced in patients with cardiac dysfunction (Lam et al., Citation2011), and elevated hexadecanedioate was associated with an increased risk of heart failure (Yu et al., Citation2016). Azelaic acid aggravated cardiomyocyte apoptosis and heart failure (Satoh et al., Citation2007), and an analogously higher level of it was observed in WT and CCK1R2R-/- mice after stress in our paper. In our study, lower creatinine, and higher hexadecanedioate and azelaic acid were found in WT and CCK1R2R-/- mice after unpredictable stress. The cardioprotective docosahexaenoic acid was increased in CCK1R2R-/- mice, in comparison with WT or CCK1R2R-/-+ST mice. Addition of taurine restrained cell apoptosis and oxidative stress, thereby promoting cardiac function recovery (Oriyanhan et al., Citation2005). Infusion of L-valine exhibited antiarrhythmic properties (Mitręga et al., Citation2011). Higher aspartate level was considered to be associated with cardiomegaly (Ritterhoff et al., Citation2020). Enhanced plasma levels of quinolinic acid (Lund et al., Citation2020), and low intake of riboflavin were found in patients with heart failure. Degradation of 5-aminopentanoic acid was involved in the development of early cardiac hypertrophy (Liu et al., Citation2021). Increased taurine and L-valine, and decreased L-aspartic acid were observed in WT + ST mice, and higher riboflavin and 5-aminopentanoic acid and reduced quinolinic acid were found in WT and CCK1R2R-/- mice after stress, and we speculated that this might be a spontaneous regulatory increase or decrease of the body to resist stress. L-tryptophan induced the secretion of CCK (Lee et al., Citation2019), and the L-tryptophan was downregulated in WT mice, which showed less content of CCK. Increased L-tryptophan alleviated unpredictable stress-induced intestinal barrier dysfunction (Yue et al., Citation2017). In compared with CCK1R2R-/-+ST mice, the L-tryptophan expression was increased in WT + ST mice, suggesting that in the presence of CCK1R2R, L-tryptophan would be produced by the body in feedback to protect the body from the damage caused by stress. Maslinic acid inhibited inflammation, prevented heart hypertrophy and protected the heart (Fang et al., Citation2022), and the higher level was found in WT mice than WT + ST mice, in CCK1R2R-/-+ST mice rather than WT + ST mice. Notably, the expression of metabolites related to fatty acids, such as palmitoleic acid, arachidonic acid, hexadecanedioate, azelaic acid and docosahexaenoic acid, were changed after CCKR knockout and unpredictable stress. Considering the influence of their changes on the heart, we speculated that CCK1R2R-/- and stress could regulate the heart function by targeting these fatty acid metabolites. Cholesterol, taurocholic acid and ADP that upregulated in WT + ST mice, were enriched in cholesterol metabolism and oxidative phosphorylation pathways. Downregulated oxoglutaric acid and cholesterol in WT mice rather than CCK1R2R-/- mice, were enriched in the citrate cycle pathway and ovarian steroidogenesis pathway. Arachidonic acid and 17-Hydroxyprogesterone that were downregulated in CCK1R2R-/- mice compared with CCK1R2R-/-+ST mice, were enriched in ovarian steroidogenesis pathway. Above differential metabolites and pathways might influence fatty acid metabolism, thereby changing myocardial damage in CCKR knockout and unpredictable stress situations. In addition, ADP and L-aspartic acid as differential metabolites between WT and WT + ST mice, were enriched in neuroactive ligand-receptor interaction pathway, and they may be associated with the changes in motion of different mice. In future studies, further investigation of these differential metabolites will be performed, which can provide a potential target for diagnosis and treatment.
5. Conclusion
CCKR knockout ameliorated cardiac function, myocardial injury, oxidative stress and inflammation that were induced by unpredictable stress. CCKR knockout seemed to affect unpredictable stress caused damage to heart through changing fatty acid metabolism.
Author contributions
Zhi-Hua Zhang, Chen-Teng Yang, Xiao-Rui Su, Ya-Ping Li and Xiao-Jing Zhang performed and analyzed the experiments; Zhi-Hua Zhang designed the study and wrote the manuscript; Song-Jun Wang and Bin Cong modified the manuscript.
Declaration of statement
No potential conflict of interest was reported by the author(s).
Supplemental Material
Download Zip (75.5 KB)Additional information
Funding
References
- Aimo, A., Castiglione, V., Borrelli, C., Saccaro, L. F., Franzini, M., Masi, S., Emdin, M., & Giannoni, A. (2020). Oxidative stress and inflammation in the evolution of heart failure: From pathophysiology to therapeutic strategies. European Journal of Preventive Cardiology, 27(5), 1–14. https://doi.org/10.1177/2047487319870344
- Buffolo, F., Tetti, M., Mulatero, P., & Monticone, S. (2022). Aldosterone as a mediator of cardiovascular damage. Hypertension, 79(9), 1899–1911. https://doi.org/10.1161/hypertensionaha.122.17964
- Cevik-Aras, H., & Ekstrom, J. (2010). Anti-inflammatory action of cholecystokinin and melatonin in the rat parotid gland. Oral Diseases, 16(7), 661–667. https://doi.org/10.1111/j.1601-0825.2010.01672.x
- Chen, X., Wan, W., Guo, Y., Ye, T., Fo, Y., Sun, Y., Qu, C., Yang, B., & Zhang, C. (2021). Pinocembrin ameliorates post-infarct heart failure through activation of Nrf2/HO-1 signaling pathway. Molecular Medicine, 27(1), 100. https://doi.org/10.1186/s10020-021-00363-7
- Creighton, J. V., de Souza Goncalves, L., Artioli, G. G., Tan, D., Elliott-Sale, K. J., Turner, M. D., Doig, C. L., & Sale, C. (2022). Physiological roles of carnosine in myocardial function and health. Advances in Nutrition, 13(5), 1914–1929. https://doi.org/10.1093/advances/nmac059
- Das, S., Komnenov, D., Newhouse, L., Rishi, A. K., & Rossi, N. F. (2022). Paraventricular nucleus v(1a) receptor knockdown blunts neurocardiovascular responses to acute stress in male rats after chronic mild unpredictable stress. Physiology & Behavior, 253, 113867. https://doi.org/10.1016/j.physbeh.2022.113867
- DiNicolantonio, J. J., Liu, J., & O’Keefe, J. H. (2018). Thiamine and cardiovascular disease: a literature review. Progress in Cardiovascular Diseases, 61(1), 27–32. https://doi.org/10.1016/j.pcad.2018.01.009
- Djoussé, L., Weir, N. L., Hanson, N. Q., Tsai, M. Y., & Gaziano, J. M. (2012). Plasma phospholipid concentration of cis-palmitoleic acid and risk of heart failure. Circulation Heart Failure, 5(6), 703–709. https://doi.org/10.1161/circheartfailure.112.967802
- Durante, W. (2019). The emerging role of l-glutamine in cardiovascular health and disease. Nutrients, 11(9), 2092. https://doi.org/10.3390/nu11092092
- Fang, M., Deng, J., Zhou, Q., Hu, Z., & Yang, L. (2022). Maslinic acid protects against pressure-overload-induced cardiac hypertrophy by blocking METTL3-mediated m(6)A methylation. Aging, 14(6), 2548–2557. https://doi.org/10.18632/aging.203860
- Han, Z. N., Lin, X. X., Wang, Y. Y., Ding, R., Hong, L., & Cui, X. (2022). Cholecystokinin octapeptide promotes ANP secretion through activation of NOX4-PGC-1alpha-PPARalpha/PPARgamma signaling in isolated beating rat atria. Oxidative Medicine and Cellular Longevity, 2022, 5905374–5905319. https://doi.org/10.1155/2022/5905374
- Hasan, S., Bilal, N., Naqvi, S., Ashraf, G. M., Suhail, N., Sharma, S., & Banu, N. (2011). Multivitamin-mineral and vitamins (E + C) supplementation modulate chronic unpredictable stress-induced oxidative damage in brain and heart of mice. Biological Trace Element Research, 142(3), 589–597. https://doi.org/10.1007/s12011-010-8771-5
- Hinterdobler, J., Schott, S., Jin, H., Meesmann, A., Steinsiek, A. L., Zimmermann, A. S., Wobst, J., Muller, P., Mauersberger, C., Vilne, B., Baecklund, A., Chen, C. S., Moggio, A., Braster, Q., Molitor, M., Krane, M., Kempf, W. E., Ladwig, K. H., Hristov, M., … Sager, H. B. (2021). Acute mental stress drives vascular inflammation and promotes plaque destabilization in mouse atherosclerosis. European Heart Journal, 42(39), 4077–4088. https://doi.org/10.1093/eurheartj/ehab371
- Hu, T., Wu, Q., Yao, Q., Jiang, K., Yu, J., & Tang, Q. (2022). Short-chain fatty acid metabolism and multiple effects on cardiovascular diseases. Ageing Research Reviews, 81, 101706. https://doi.org/10.1016/j.arr.2022.101706
- Jensen, M. D. (2006). Potential role of new therapies in modifying cardiovascular risk in overweight patients with metabolic risk factors. Obesity, 14 Suppl 3, 143s–149s. https://doi.org/10.1038/oby.2006.294
- Karwi, Q. G., Uddin, G. M., Ho, K. L., & Lopaschuk, G. D. (2018). Loss of metabolic flexibility in the failing heart. Frontiers in Cardiovascular Medicine, 5, 68. https://doi.org/10.3389/fcvm.2018.00068
- Kuebler, U., Zuccarella-Hackl, C., Arpagaus, A., Wolf, J. M., Farahmand, F., von Känel, R., Ehlert, U., & Wirtz, P. H. (2015). Stress-induced modulation of NF-κB activation, inflammation-associated gene expression, and cytokine levels in blood of healthy men. Brain, Behavior, and Immunity, 46, 87–95. https://doi.org/10.1016/j.bbi.2014.12.024
- Kurosawa, M., Iijima, S., Funakoshi, A., Kawanami, T., Miyasaka, K., Bucinskaite, V., & Lundeberg, T. (2001). Cholecystokinin-8 (CCK-8) has no effect on heart rate in rats lacking CCK-A receptors. Peptides, 22(8), 1279–1284. https://doi.org/10.1016/s0196-9781(01)00452-1
- Lam, C. S., Lyass, A., Kraigher-Krainer, E., Massaro, J. M., Lee, D. S., Ho, J. E., Levy, D., Redfield, M. M., Pieske, B. M., Benjamin, E. J., & Vasan, R. S. (2011). Cardiac dysfunction and noncardiac dysfunction as precursors of heart failure with reduced and preserved ejection fraction in the community. Circulation, 124(1), 24–30. https://doi.org/10.1161/CIRCULATIONAHA.110.979203
- Lavine, J. A., Kibbe, C. R., Baan, M., Sirinvaravong, S., Umhoefer, H. M., Engler, K. A., Meske, L. M., Sacotte, K. A., Erhardt, D. P., & Davis, D. B. (2015). Cholecystokinin expression in the β-cell leads to increased β-cell area in aged mice and protects from streptozotocin-induced diabetes and apoptosis. American Journal of Physiology. Endocrinology and Metabolism, 309(10), E819–828. https://doi.org/10.1152/ajpendo.00159.2015
- Lee, S. B., Lee, K. W., Wang, T., Lee, J. S., Jung, U. S., Nejad, J. G., Oh, Y. K., Baek, Y. C., Kim, K. H., & Lee, H. G. (2019). Intravenous administration of L-tryptophan stimulates gastrointestinal hormones and melatonin secretions: study on beef cattle. Journal of Animal Science and Technology, 61(4), 239–244. https://doi.org/10.5187/jast.2019.61.4.239
- Liu, J., Hu, J., Tan, L., Zhou, Q., & Wu, X. (2021). Abnormalities in lysine degradation are involved in early cardiomyocyte hypertrophy development in pressure-overloaded rats. BMC Cardiovascular Disorders, 21(1), 403. https://doi.org/10.1186/s12872-021-02209-w
- Lund, A., Nordrehaug, J. E., Slettom, G., Solvang, S. H., Pedersen, E. K., Midttun, O., Ulvik, A., Ueland, P. M., Nygard, O., & Giil, L. M. (2020). Plasma kynurenines and prognosis in patients with heart failure. PLoS One, 15(1), e0227365. https://doi.org/10.1371/journal.pone.0227365
- MacCarter, D., Vijay, N., Washam, M., Shecterle, L., Sierminski, H., & St Cyr, J. A. (2009). D-ribose aids advanced ischemic heart failure patients. International Journal of Cardiology, 137(1), 79–80. https://doi.org/10.1016/j.ijcard.2008.05.025
- Mitręga, K., Zorniak, M., Varghese, B., Lange, D., Nożynski, J., Porc, M., Białka, S., & Krzemiński, T. F. (2011). Beneficial effects of l-leucine and l-valine on arrhythmias, hemodynamics and myocardial morphology in rats. Pharmacological Research, 64(3), 218–225. https://doi.org/10.1016/j.phrs.2011.04.011
- Nadella, S., Ciofoaia, V., Cao, H., Kallakury, B., Tucker, R. D., & Smith, J. P. (2020). Cholecystokinin receptor antagonist therapy decreases inflammation and fibrosis in chronic pancreatitis. Digestive Diseases and Sciences, 65(5), 1376–1384. https://doi.org/10.1007/s10620-019-05863-5
- Noreen, S., Rehman, H. U., Tufail, T., Badar Ul Ain, H., & Awuchi, C. G. (2023). Secoisolariciresinol diglucoside and anethole ameliorate lipid abnormalities, oxidative injury, hypercholesterolemia, heart, and liver conditions. Food Science & Nutrition, 11(6), 2620–2630. https://doi.org/10.1002/fsn3.3250
- Oriyanhan, W., Yamazaki, K., Miwa, S., Takaba, K., Ikeda, T., & Komeda, M. (2005). Taurine prevents myocardial ischemia/reperfusion-induced oxidative stress and apoptosis in prolonged hypothermic rat heart preservation. Heart and Vessels, 20(6), 278–285. https://doi.org/10.1007/s00380-005-0841-9
- Qipshidze-Kelm, N., Piell, K. M., Solinger, J. C., & Cole, M. P. (2013). Co-treatment with conjugated linoleic acid and nitrite protects against myocardial infarction. Redox Biology, 2, 1–7. https://doi.org/10.1016/j.redox.2013.10.009
- Ritterhoff, J., Young, S., Villet, O., Shao, D., Neto, F. C., Bettcher, L. F., Hsu, Y. A., Kolwicz, S. C., Jr., Raftery, D., & Tian, R. (2020). Metabolic remodeling promotes cardiac hypertrophy by directing glucose to aspartate biosynthesis. Circulation Research, 126(2), 182–196. https://doi.org/10.1161/CIRCRESAHA.119.315483
- Saia, R. S., Bertozi, G., Mestriner, F. L., Antunes-Rodrigues, J., Queiróz Cunha, F., & Cárnio, E. C. (2013). Cardiovascular and inflammatory response to cholecystokinin during endotoxemic shock. Shock, 39(1), 104–113. https://doi.org/10.1097/SHK.0b013e3182793e2e
- Sara, J. D. S., Lerman, L. O., & Lerman, A. (2021). The endothelium is a key player in the vascular response to acute mental stress. European Heart Journal, 42(39), 4089–4091. https://doi.org/10.1093/eurheartj/ehab510
- Sartor, D. M., & Verberne, A. J. (2002). Cholecystokinin selectively affects presympathetic vasomotor neurons and sympathetic vasomotor outflow. American Journal of Physiology. Regulatory, Integrative and Comparative Physiology, 282(4), R1174–1184. https://doi.org/10.1152/ajpregu.00500.2001
- Satoh, M., Matter, C. M., Ogita, H., Takeshita, K., Wang, C. Y., Dorn, G. W., 2nd., & Liao, J. K. (2007). Inhibition of apoptosis-regulated signaling kinase-1 and prevention of congestive heart failure by estrogen. Circulation, 115(25), 3197–3204. https://doi.org/10.1161/CIRCULATIONAHA.106.657981
- Starcke, K., & Brand, M. (2012). Decision making under stress: a selective review. Neuroscience and Biobehavioral Reviews, 36(4), 1228–1248. https://doi.org/10.1016/j.neubiorev.2012.02.003
- Toko, H., Morita, H., Katakura, M., Hashimoto, M., Ko, T., Bujo, S., Adachi, Y., Ueda, K., Murakami, H., Ishizuka, M., Guo, J., Zhao, C., Fujiwara, T., Hara, H., Takeda, N., Takimoto, E., Shido, O., Harada, M., & Komuro, I. (2020). Omega-3 fatty acid prevents the development of heart failure by changing fatty acid composition in the heart. Scientific Reports, 10(1), 15553. https://doi.org/10.1038/s41598-020-72686-0
- van der Pol, A., van Gilst, W. H., Voors, A. A., & van der Meer, P. (2019). Treating oxidative stress in heart failure: past, present and future. European Journal of Heart Failure, 21(4), 425–435. https://doi.org/10.1002/ejhf.1320
- Velasco, C., Comesana, S., Conde-Sieira, M., Miguez, J. M., & Soengas, J. L. (2019). Effects of CCK-8 and GLP-1 on fatty acid sensing and food intake regulation in trout. Journal of Molecular Endocrinology, 62(3), 101–116. https://doi.org/10.1530/JME-18-0212
- Wang, L., Hiller, H., Smith, J. A., de Kloet, A. D., & Krause, E. G. (2016). Angiotensin type 1a receptors in the paraventricular nucleus of the hypothalamus control cardiovascular reactivity and anxiety-like behavior in male mice. Physiological Genomics, 48(9), 667–676. https://doi.org/10.1152/physiolgenomics.00029.2016
- Wei, Y., & Wang, B. (2021). The expression levels of plasma dimethylglycine (DMG), human maternally expressed gene 3 (MEG3), and Apelin-12 in patients with acute myocardial infarction and their clinical significance. Annals of Palliative Medicine, 10(2), 2175–2183. https://doi.org/10.21037/apm-21-122
- Westfall, S., Caracci, F., Zhao, D., Wu, Q. L., Frolinger, T., Simon, J., & Pasinetti, G. M. (2021). Microbiota metabolites modulate the T helper 17 to regulatory T cell (Th17/Treg) imbalance promoting resilience to stress-induced anxiety- and depressive-like behaviors. Brain, Behavior, and Immunity, 91, 350–368. https://doi.org/10.1016/j.bbi.2020.10.013
- Williamson, C., Gorelik, J., Eaton, B. M., Lab, M., de Swiet, M., & Korchev, Y. (2001). The bile acid taurocholate impairs rat cardiomyocyte function: a proposed mechanism for intra-uterine fetal death in obstetric cholestasis. Clinical Science, 100(4), 363–369. https://doi.org/10.1042/cs1000363
- Wu, J., Cao, M., Hu, M., Gong, Y., Xue, J., Yang, Y., & Zhou, H. (2022). Intervention effects of okra extract on brain-gut peptides and intestinal microorganisms in sleep deprivation rats. Evidence-Based Complementary and Alternative Medicine, 2022, 9855411–9855413. https://doi.org/10.1155/2022/9855411
- Wu, T., Yao, H., Zhang, B., Zhou, S., Hou, P., & Chen, K. (2021). 2021). Kappa opioid receptor agonist inhibits myocardial injury in heart failure rats through activating Nrf2/HO-1 pathway and regulating Ca(2+)-SERCA2a. Oxidative Medicine and Cellular Longevity, 2021, 7328437. https://doi.org/10.1155/2021/7328437
- Yamamoto, T., & Sano, M. (2022). Deranged myocardial fatty acid metabolism in heart failure. International Journal of Molecular Sciences, 23(2), 996. https://doi.org/10.3390/ijms23020996
- Yu, B., Li, A. H., Metcalf, G. A., Muzny, D. M., Morrison, A. C., White, S., Mosley, T. H., Gibbs, R. A., & Boerwinkle, E. (2016). Loss-of-function variants influence the human serum metabolome. Science Advances, 2(8), e1600800. https://doi.org/10.1126/sciadv.1600800
- Yue, Y., Guo, Y., & Yang, Y. (2017). Effects of dietary L-tryptophan supplementation on intestinal response to chronic unpredictable stress in broilers. Amino Acids, 49(7), 1227–1236. https://doi.org/10.1007/s00726-017-2424-3
- Zeng, Q., Ou, L., Wang, W., & Guo, D. Y. (2020). Gastrin, cholecystokinin, signaling, and biological activities in cellular processes. Frontiers in Endocrinology, 11, 112. https://doi.org/10.3389/fendo.2020.00112
- Zhao, X. Y., Ling, Y. L., Li, Y. G., Meng, A. H., & Xing, H. Y. (2005). Cholecystokinin octapeptide improves cardiac function by activating cholecystokinin octapeptide receptor in endotoxic shock rats. World Journal of Gastroenterology, 11(22), 3405–3410. https://doi.org/10.3748/wjg.v11.i22.3405