Abstract
Stress is a series of physical and psychological responses to external and internal environmental stimuli. Growing studies have demonstrated the detrimental impacts of acute restraint stress (ARS) and chronic restraint stress (CRS) on animal behavior. However, the related pathogenesis and therapeutic mechanisms remain unclear. Hence, the present study aimed to examine whether unfolded protein response (UPR) and Kelch-like ECH-associated protein 1 (Keap1)-nuclear factor erythroid 2 related factor 2 (Nrf2) pathway are associated with ARS- and CRS- induced abnormal behaviors of pain sensitivity and cognitive function. We here used four behavioral tests to evaluate pain sensitivity and cognitive function in ARS and CRS mice. CRS markedly decreased Paw Withdrawal Mechanical Threshold (PWMT) and Tail-flick Latency (TFL) scores, whereas ARS altered TFL but had no effect on PWMT scores. Additionally, CRS, but not ARS, significantly changed behaviors in nest building behavior and MWMT. Intriguingly, the expression of Keap1 and Nrf2 protein were decreased in the spinal cord and hippocampus in CRS mice, but not in ARS mice. Moreover, neither the ARS nor the CRS groups significantly differed from the control group in terms of endoplasmic reticulum stress (ERS). Taken together, this study demonstrated that CRS could induce abnormal pain sensitivity and cognitive function probably via Keap1/Nrf2 pathway in spinal cord and hippocampus. It is therefore likely that effective intervention of Keap1/Nrf2 pathway may contribute to preventing and treating hyperalgesia and cognitive dysfunction in CRS.
1. Introduction
Stress is aroused by an aversive situation, which is a series of physical and psychological responses to external and internal environmental stimuli. Physiology and neuropsychiatric disorders like hyperalgesia, anxiety, autism, and Alzheimer’s disease (AD) are greatly influenced by stress (Daudelin-Peltier et al., Citation2017; De Gregorio et al., Citation2022; Weger & Sandi, Citation2018; Yuede et al., Citation2018). Stress and pain are two distinct yet overlapping processes that share several conceptual and physiological similarities (Abdallah & Geha, Citation2017). Additionally, it has been reported that chronic stress can trigger hyperalgesia, which is an increased sensitivity to painful stimuli (Bardin et al., Citation2009). Restraint stress is similar to the pathogenesis of human psychosomatic diseases, so the restraint stress model is one of the most commonly used stress models in mimicking human physical and mental diseases (Howland & Cazakoff, Citation2010). The restraint stress can be classified into acute restraint stress (ARS) and chronic restraint stress (CRS) according to the different protocols of modeling. It is well-recognized that short-term or long-term stress could affect activity and long-term abnormalities in the central nervous system (Imbe et al., Citation2006). Specifically, depression-like behaviors of mice could be developed followed by exposure to chronic social defeat stress (Yang et al., Citation2017), and chronic forced swimming stress could develop anxiety-like behaviors (Dhingra et al., Citation2014). Although we previously demonstrated that altered brain-derived neurotrophic factor (BDNF)-mammalian target of rapamycin (mTOR) pathway may participate in CRS-induced hyperalgesia (Huang et al., Citation2019), the differences in pain and cognitive behaviors between ARS and CRS are not fully understood and the underlying mechanisms remain unclear.
Endoplasmic reticulum stress (ERS), an evolutionarily conserved adaptive response, which occurs due to the perturbations of ER homeostasis, has been reported to play a vital role in many diseases such as neuropathic pain, AD, Parkinson’s disease (PD) and depression (Hetz et al., Citation2020; Marciniak et al., Citation2022; Muneer & Shamsher Khan, Citation2019). In attempt to retain the intracellular homeostasis, unfolded protein response (UPR) was activated. Binding immunoglobulin protein (BIP/GRP78) dissociate from the three sensors of ER: inositol-requiring enzyme1 (IRE1), protein kinase RNA-like ER kinase (PERK), and activating transcription factor 6 (ATF6), and then the three pathways were activated (Kopp et al., Citation2019). It has been reported that UPR might be involved in neuropathic pain (Zhang et al., Citation2015). Specifically, the major chaperone BIP was activated in the neurons of the spinal cord in the SNL model, followed by the IRE and ATF6 pathways but not the PERK pathway. Inhibition of ATF6 reduced SNL-induced pain behavior. Several studies demonstrated amyloid precursor protein (APP) and presenilin1 (PS1) double-transgenic (Tg) AD mouse models increased protein levels of UPR signaling pathways (Branca et al., Citation2017).
Nuclear factor erythroid-derived 2-related factor 2 (Nrf2), a transcription factor, is essential for the antioxidant defense system and mediates the cell survival response (Li et al., Citation2018). Under normal conditions, Nrf2 interacts with kelch-like erythroid cell-derived protein with CNC homology (ECH)-associated protein1 (Keap1), a repressive protein for Nrf2 that controls the degradation of Nrf2 in the cytoplasm (Wang et al., Citation2016; Yamamoto et al., Citation2018). In our previous study, we demonstrated that Keap1/Nrf2 signaling pathway exerts beneficial effects in improving anhedonia symptoms induced by chronic neuropathic pain (Li et al., Citation2018). Zhang et al reported that Keap1/Nrf2 signaling might support stress resilience in the learned helplessness paradigm (Zhang et al., Citation2018). Additionally, deficiency of Nrf2 increases Aβ production, by contrast, activation of Nrf2 ameliorates cognitive dysfunction in AD mice (Bahn et al., Citation2019; Ramsey et al., Citation2007). Collectively, it seems that Keap1/Nrf2 pathway was involved in stress response.
Comorbidity of pain and cognitive dysfunction has been well established. Numerous preclinical and clinical studies have suggested that postoperative pain and analgesics applied during surgery might affect cognitive function postoperatively (Fong et al., Citation2006; Gu et al., Citation2019; O’Gara et al., Citation2021). Additionally, previous studies demonstrated that post-traumatic stress disorder symptoms were related to an incidence of chronic back pain (Suri et al., Citation2019). Therefore, surgery as a stressful stimulus may affect the pain sensitivity and cognitive function of patients.
Taken together, the present study was conducted to compare the behaviors of pain sensitivity and cognitive function in ARS and CRS. Furthermore, we examined the alteration of protein levels of UPR and Keap1/Nrf2 signaling pathways in ARS and CRS, and attempted to offer a new strategy to prevent and treat stress-induced abnormal behaviors.
2. Materials and methods
2.1. Animals
A total of 34 male C57BL/6 mice (2-month-old) were purchased from the Laboratory Animal Center of Tongji hospital. Animals were housed in groups (3–5 per cage) at a controlled temperature of 22 ± 2 °C and a relative humidity of 60 ± 5% with food and water ad libitum. This study was approved by the Experimental Animal Committee of Tongji Hospital, Tongji Medical College, Huazhong University of Science and Technology (Wuhan, China). All experimental procedures were carried out in strict accordance with the National Institute of Health Guide for the Care and Use of Laboratory Animals.
2.2. ARS procedure
ARS procedure was performed by a method described previously (Mitsumoto & Mori, Citation2018; Zhang et al., Citation2019). Sixteen mice were randomly assigned into control group or ARS group. The control mice were housed in their home cages while the mice in the ARS group were restrained in a 50-ml centrifuge tube from 9:00 to 11:00 a.m. for 2 hours. Animals in the ARS group were able to move their limbs and head but not their trunks, and both control and ARS mice were unable to access water and food during the duration of ARS exposure.
2.3. CRS procedures
CRS procedure was performed by a method described previously with minor modifications (Rafa-Zabłocka et al., Citation2021; Woo et al., Citation2018). Eighteen mice were assigned to control group and CRS group. CRS mice were restrained for 6 h/day (from 9:00 a.m. to 15:00 p.m.) in a 50-ml centrifuge tube for 2 weeks, while the mice in control group were housed in their home cages. Animals in the CRS group were only able to move their limbs and head but not their trunks, and neither control mice nor CRS mice were able to access water and food during the duration of CRS exposure.
2.4. Paw withdrawal mechanical threshold (PWMT)
Mechanical allodynia was accessed by evaluating hind paw withdrawal mechanical threshold (PWMT) in response to the von Frey apparatus stimuli (Castany et al., Citation2018). Briefly, to avoid the stress caused by the test conditions, mice were kept in chambers with a wire net floor for 30 min. PWMT was evaluated by the “up and down” method after being stimulated at a force ranging 0.008–2 g. Sudden paw withdrawal, lickings and shaking were regarded as positive responses, 0.6 g von Frey filament was firstly applied to observe baseline responses. Once a positive response occurred, a weaker filament was selected, and a stronger filament was selected when no respond was observed. After the first response, four assessments were made at an interval of 10 s, and the cutoff force value was 2 g for the mice. The formula of [(10(Xf + κδ)/10,000)] was used to calculate the 50% PWMT. After testing left and right hind paws, the final PWMT was determined using mean values.
2.5. Tail-flick latency (TFL)
Thermal nociceptive thresholds in mice were accessed using a tail-flick latency test (Cui et al., Citation2008; Raghavendra et al., Citation2002). The tail was immersed into water (50 °C ± 0.5 °C), and a positive response was characterized as a tail rapidly flicking. The latency was recorded, and a 10-s cutoff time was set to prevent tail damage. The final TFL latency was calculated as the mean of three tests that were conducted at 10-minute intervals.
2.6. Nest-building assay
After restraint stress, thirty-two pieces of rectangle odorless tissue paper with the same size (5 × 7 cm) were added to each cage at 16:00, and scores were measured on the next day (9:00 am) (Woo et al., Citation2018). Pictures of nests were taken with a camera, and each nest of each mouse was graded on a scale of 1 to 5 (5 being outstanding nest building characterized by the size of the pieces of bitten paper and cohesive bowl shape) by a rater who was unaware of the experimental design.
2.7. Morris water maze test (MWMT)
MWMT was used to assess the function of spatial learning and memory (Zhan et al., Citation2019). The MWMT consists of a five-day training trials and a one-day probe testing. All training trials were conducted in a circular pool (120 cm diameter and 50 cm height), with a 10-cm-diameter hidden platform submerged 1 cm below the water surface in the target quadrant. Each mouse was given 1 min to reach the hidden platform, and the mice were gently lead to the platform and given permission to stay there for 15 s if they didn’t get there within 1 min. The time spent to find the platform (escape latency) was recorded and the number of times the mice traversed the platform area (platform crossing) was recorded on the 60-s probe trial by a digital video camera.
2.8. Western blot
Following 5% isoflurane anesthesia, the mice were swiftly sacrificed, and the L3-L5 spinal cord segments and hippocampus of mice were extracted. Samples were homogenized with RIPA lysis buffer combined with proteinase inhibitors. The supernatants were then collected after centrifuging at 12,000 rpm for 15 min at 4 °C. Subsequently, the protein concentration in the supernatant was measured using a BCA protein assay kit (Boster, Wuhan, China). The samples were separated by gel electrophoresis and were transferred to polyvinylidene fluoride membranes (Millipore, Bedford, MA, USA). Bands were blocked for 1 h at room temperature with 5% bovine serum albumin (BSA). Relative primary antibodies were incubated at 4 °C overnight: rabbit anti-Keap1 (1:1000; Proteintech, Rosemont, IL, USA, 10503-2-AP), rabbit anti-Nrf2 (1:1000; Proteintech, Rosemont, IL, USA, 16396-1-AP), rabbit anti-GRP78 (1:1000; Affinity, Cincinnati, OH, USA, AF5366), rabbit anti-p-PERK (1:500; Affinity, Cincinnati, OH, USA, DF7576), rabbit anti-PERK (1:500; Affinity, Cincinnati, OH, USA, AF5304), rabbit anti-p-IRE1 (1:500; Affinity, Cincinnati, OH, USA, DF8322), rabbit anti-IRE1 (1:500; Affinity, Cincinnati, OH, USA, DF7709), rabbit anti-ATF6 (1:500; Affinity, Cincinnati, OH, USA, DF6009), and rabbit anti-GAPDH (1:2000; Affinity, Cincinnati, OH, USA, AF7021). Next, membranes were washed and incubated for 1 h at room temperature with corresponding second antibody. Finally, these membranes were washed and detected by enhanced chemiluminescence reagents (Qidongzi, Wuhan, China) with the ChemiDocXRS chemiluminescence imaging system (BioRad, Hercules, CA, USA).
2.9. Statistical analysis
Values presented are expressed as mean ± standard error of the mean (S.E.M.). Statistical analyzes were performed using SPSS software version 17.0 (SPSS Inc., Armonk, New York, USA). Results of Nest-building score, platform crossing, and western blotting were analyzed using the unpaired t-test. Other data were analyzed using two-way ANOVA with repeated measures followed by post-hoc Tukey’s test. P < 0.05 was considered statistically significant.
3. Results
3.1. The scores of PWMT and TFL between control and ARS mice
PWMT was used to evaluate mechanical allodynia, and thermal pain thresholds in mice were accessed by a tail flick latency test (Fregni et al., Citation2018; Yang et al., Citation2018). As shown in , the results showed no changes in body weight () and PWMT () between the groups, while TFL scores () in mice received ARS were significantly lower than those in control mice on day 1.
Figure 1. Effects of ARS on PWMT and TFL in mice. (A) The schedule of ARS and behavioral tests. Behavioral tests, including the PWMT and TFL were performed on day 0 after acclimation (baseline), and 2 hours after ARS on day 1. Nest-building behavioral test was performed on day 1 (16:00 p.m.) and measured on day 2 (09:00 a.m.). Mice were scheduled for MWMT and probe trial on days 2-7. Tissue samples were collected for western blotting on day 8. (B) Body weight (two-way ANOVA; Time: F1,7 = 3.5, P = 0.1036; Group: F1,7 = 3.15, P = 0.1192; Interaction: F1,7 = 1.75, P = 0.2275). (C) PWMT (two-way ANOVA; Time: F1,7 = 18.68, P < 0.01; Group: F1,7 = 0.0179, P = 0.8973; Interaction: F1,7 = 4.343, P = 0.0756). (D) TFL (two-way ANOVA; Time: F1,7 = 8.547, P < 0.05; Group: F1,7 = 4.706, P = 0.0667; Interaction: F1,7 = 7.29, P = 0.0306). Data are shown as mean ± SEM (n = 8). *P < 0.05, **P < 0.01. ARS: acute restraint stress; MWMT: Morris water maze test; N.S.: Not significant; PWMT: Paw withdrawal mechanical threshold; TFL: Tail-flick latency.
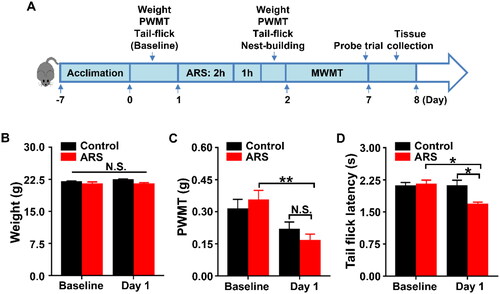
3.2. Effects of ARS on the results of nest building and MWMT behaviors
Nest building is widely utilized for assessing the quality of daily living, and is crucial for heat preservation, reproduction and shelter throughout the animal kingdom (Deacon, Citation2006; Li et al., Citation2019). In this study, we revealed that there was no notable change in nest building scores between the groups (). MWMT is applied to evaluating spatial learning and memory function in rodents (Gao et al., Citation2018). There were no changes in escape latency and platform crossing in ARS mice as compared with that of control (), indicating that cognitive function was similar between the groups.
Figure 2. Comparisons of Nest-building score and MWMT between the Control and ARS groups. (A) Representative results of nest building behavior assay are shown. (B) Nest-building score (t-test, P > 0.05). (C) Representative trace graphs of Control and ARS mice in the MWMT. (D) Escape latency (two-way ANOVA; Time: F4,28 = 13.91, P < 0.001; Group: F1,7 = 1.328, P = 0.2870; Interaction: F4,28 = 0.8338, P = 0.5152). (E) Platform crossing (t-test, P > 0.05). Data are shown as mean ± SEM (n = 8). ARS: acute restraint stress; N.S.: Not significant.
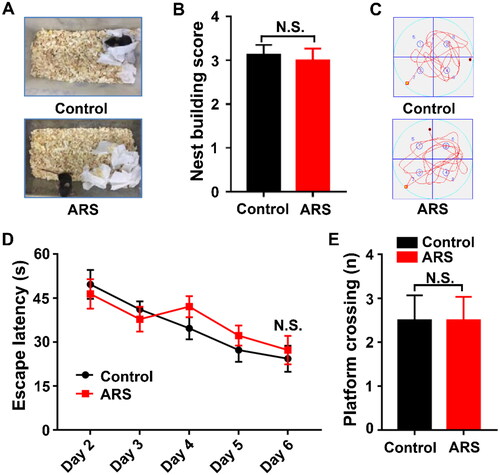
3.3. Effects of ARS on the protein levels of UPR and the Keap1-Nrf2 pathway in hippocampus
GRP78, a marker of ER stress, could dissociate from the sensors (PERK, IRE1 and ATF6) resulting in the activation of UPR. We detected the protein expression levels of GRP78, p-PERK, PERK, p-IRE1, IRE1, and ATF6, in hippocampus of control and ARS mice. We found the levels of all these proteins were not substantially different from the control mice (). Additionally, the levels of Keap1 and Nrf2 in hippocampus also did not significantly differ between the two groups ().
Figure 3. Levels of UPR and Keap1/Nrf2 pathway in hippocampus between control and ARS mice. (A) Representative immunoblot bands of GRP78, p-PERK, PERK, p-IRE1, IRE1, ATF6, Keap1 and Nrf2 expression in the hippocampus in control and ARS groups. (B-I) Western blot analysis of GRP78 (t = 0.2041, P = 0.8424), p-PERK/PERK ratio (t = 0.2476, P = 0.8095), PERK (t = 1.752, P = 0.1103), p-IRE1/IRE1 ratio (t = 0.03252, P = 0.9747), IRE1 (t = 0.2047, P = 0.8419), ATF6 (t = 0.2144, P = 0.8345), Keap1 (t = 0.1692, P = 0.8690), Nrf2 (t = 0.2491, P = 0.8083). Data are shown as mean ± SEM (n = 6).
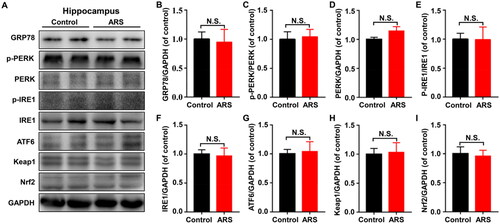
3.4. Comparisons of PWMT and TFL between control and CRS mice
To mimic the CRS model, mice were restrained for 6 h/day in a 50-ml centrifuge tube for 2 weeks (). On days 1, 8, and 15, the mice’s body weights in the two groups did not differ significantly from one another (). Interestingly, CRS mice showed a considerably lower PWMT and TFL score on day 15 than that of control mice ().
Figure 4. Effects of CRS on PWMT and TFL in mice. (A) The schedule of CRS and behavioral tests. Behavioral tests, including the PWMT and TFL were performed on day 0 after acclimation (baseline), and 24 hours after CRS on day 15. Nest-building behavioral test was performed on day 15 (16:00 p.m.) and measured on day 16 (09:00 a.m.). Mice were scheduled for MWMT and probe trial on days 16–21. Tissue samples were collected for western blotting on day 22. (B) Body weight (two-way ANOVA; Time: F2,16 = 1.636, P = 0.2257; Group: F1,8 = 0.07088, P = 0.7968; Interaction: F2,16 = 3.726, P = 0.0469). (C) PWMT (two-way ANOVA; Time: F1,8 = 34.73, P < 0.001; Group: F1,8 = 5.624, P < 0.05; Interaction: F1,8 = 30.65, P < 0.001). (D) TFL (two-way ANOVA; Time: F1,8 = 2.482, P = 0.1538; Group: F1,8 = 5.359, P < 0.05; Interaction: F1,8 = 7.343, P < 0.05). Data are shown as mean ± SEM (n = 9). *P < 0.05, ***P < 0.001.
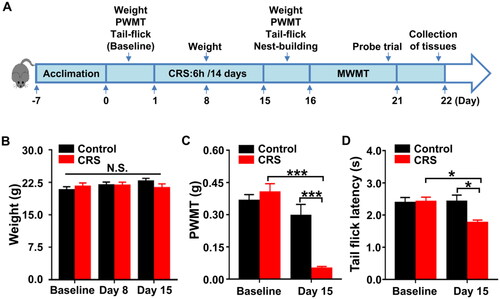
3.5. Alterations in nest building and MWMT behaviors after CRS in mice
Nest construction behavior was assessed by rating the nest’s quality on a 5-point scale (Woo et al., Citation2018). As compared with control group, CRS mice had more trouble establishing a nest with nestlets (). Next, cognitive behaviors in the two groups were measured by the MWMT. CRS mice showed a noticeably longer escape latency on day 19 and day 20 (). Platform crossing times during the probe trial were markedly lower in CRS mice compared with control mice (). Thus, compared to control mice, CRS animals were predicted to have deficiencies in spatial learning and memory.
Figure 5. Comparisons of Nest-building score and MWMT between the Control and CRS groups. (A) Nest-building behavior assay. Representative results are shown. (B) Nest-building score (t-test, P < 0.05). (C) Representative trace graphs of Control and CRS mice in the MWMT. (D) Escape latency (two-way ANOVA; Time: F4,32 = 1.65, P = 0.1859; Group: F1,8 = 4.097, P = 0.0776; Interaction: F4,32 = 5.091, P < 0.01). (E) Platform crossing (t-test, P < 0.05). Data are shown as mean ± SEM (n = 9). *P < 0.05, **P < 0.01.
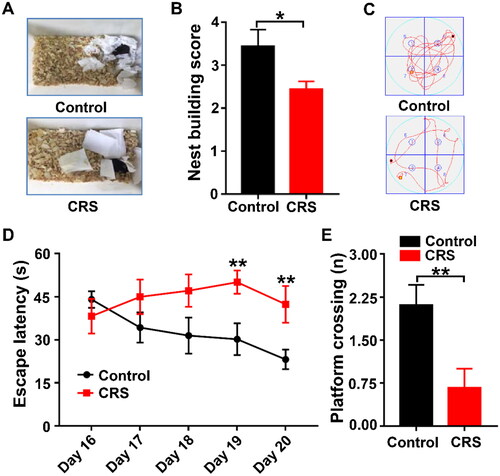
3.6. Effects of CRS on the protein levels of UPR and the Keap1-Nrf2 in spinal cord and hippocampus
We determined the expression levels of GRP78, p-PERK, PERK, p-IRE1, IRE1, ATF6, Keap1 and Nrf2 in spinal cord and hippocampus of control and CRS mice. The levels of Keap1 and Nrf2 were significantly lower in the spinal cord of CRS mice than that of the control mice, while the levels of the ERS-related proteins showed no significant difference (). Moreover, the levels of Keap1 and Nrf2 in hippocampus of CRS mice were significantly decreased, but the levels of the other proteins had no significant difference ()
Figure 6. Levels of UPR and Keap1/Nrf2 pathway in spinal cord and hippocampus between control and CRS mice. (A) Representative immunoblot bands of GRP78, p-PERK, PERK, p-IRE1, IRE1, ATF6, Keap1, and Nrf2 expression in the spinal cord in control and CRS groups. (B-I) Western blot analysis of GRP78 (t = 1.616, P = 0.1372), p-PERK/PERK ratio (t = 0.595, P = 0.5651), PERK (t = 1.093, P = 0.3002), p-IRE1/IRE1 ratio (t = 1.014, P = 0.3343), IRE1 (t = 0.129, P = 0.8999), ATF6 (t = 0.2401, P = 0.8151), Keap1 (t = 6.201, P < 0.001), and Nrf2 (t = 3.446, P < 0.01) in the spinal cord. (J) Representative immunoblot bands of GRP78, p-PERK, PERK, p-IRE1, IRE1, ATF6, Keap1 and Nrf2 expression in the hippocampus in control and CRS groups. (K-S) Western blot analysis of GRP78 (t = 0.09809, P = 0.9238), p-PERK/PERK ratio (t = 0.0761, P = 0.9408), PERK (t = 0.1743, P = 0.8651), p-IRE1/IRE1 ratio (t = 0.3999, P = 0.6976), IRE1 (t = 0.4633, P = 0.6531), ATF6 (t = 0.08465, P = 0.9342), Keap1 (t = 2.252, P < 0.05), Nrf2 (t = 2856, P < 0.05) in the hippocampus. Data are shown as mean ± SEM (n = 6). *P < 0.05, **P < 0.01, ***P < 0.001.
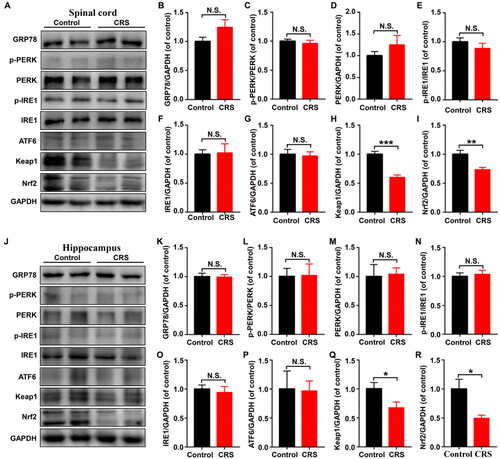
3.7. Correlation analysis between escape latency and pain sensitivity
Escape latency, a reflection of spatial learning and memory, was chosen to represent MWMT behaviors. Correlations among escape latency, PWMT and TFL were evaluated, respectively. The results revealed that PWMT (), but not TFL (), was negatively correlated with escape latency in CRS group, while in ARS group, there was no meaningful correlation between escape latency and pain sensitivity ().
Figure 7. Correlation analysis between escape latency and pain sensitivity in ARS and CRS. (A) Correlation between escape latency and PWMT in ARS (r = −0.0006, P = 0.9981). (B) Correlation between escape latency and TFL in ARS (r = 0.2198, P = 0.4134). (C) Correlation between escape latency and PWMT in CRS (r = −0.4784, P = 0.0446). (D) Correlation between escape latency and TFL in CRS (r = −0.3591, P = 0.1433). PWMT: Paw withdrawal mechanical threshold.
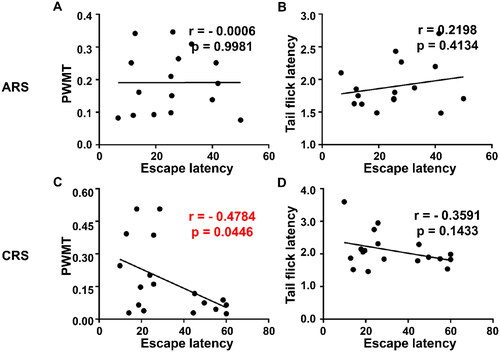
4. Discussion
Chronic restraint stress is a harmful stimulus that can result from human living conditions, such as crowding and frustration (Huang et al., Citation2019). Rodent models are commonly used to construct and study psychological stress include restraint, footshock, and defeat, etc. (Musazzi et al., Citation2019; Woo et al., Citation2018; Yang et al., Citation2018). The following are the study’s principle findings (as shown in ): Firstly, CRS, but not ARS, showed deficiencies in spatial learning and memory as well as impairments in daily living activity. Secondly, Keap1/Nrf2 signaling pathway, but not UPR pathway, in spinal cord and hippocampus of CRS mice were markedly lower than those of control mice, whereas there was no significant difference in ARS mice. Additionally, escape latency in MWMT and PWMT was significantly correlated in CRS. These findings suggest that stress can induce abnormality in pain sensitivity and cognitive function, and its mechanisms are probably related to alterations in Keap1-Nrf2 signaling pathways.
Growing evidence demonstrates that exposure to stressor obviously impacts pain sensitivity (Chen et al., Citation2011; Reinhardt et al., Citation2013), a previous study from our team also revealed that CRS significantly altered pain sensitivity (Huang et al., Citation2019). Consistent with this, we found in this study that exposure to CRS for 14 consecutive days markedly affect mechanical allodynia and thermal nociceptive thresholds, as measured by PWMT and TFL in mice. Interestingly, mice after ARS exposure for 2 hours one time significantly decreased TFL, while there was no obvious difference in PWMT scores compared with that of control group. Robson et al reported that thiopental, raised the thermal threshold while decreasing the mechanical threshold (Robson et al., Citation1965). In this regard, these studies with our findings suggest that sensory and transmission mechanisms of pain sensitivity might be distinct between ARS and CRS. We previously indicated that sulforaphane (SFN), an activator of Nrf2, exerted beneficial effects on neuropathic pain induced anhedonia (Li et al., Citation2018). Keap1 is an adapter protein that represses Nrf2 by promoting its degradation under normal conditions (Li et al., Citation2018). It has been discovered that absence of the Nrf2 gene resulted in changes to the expression of genes associated with various functions, including metabolism, transport activity, signal transduction, cell adhesion, and transcriptional regulation (Muramatsu et al., Citation2013). Additionally, it has been reported that the Keap1/Nrf2 pathway is involved in chronic social defeat stress induced depression behavior (Yao et al., Citation2016). In the study, we discovered that protein levels of Keap1 and Nrf2 in spinal cord were significantly decreased in CRS mice, but not in ARS mice. These findings indicated that alterations in Keap1/Nrf2 signaling may contribute to pain sensitivity induced by CRS, although further detailed studies are greatly needed.
Nesting behaviors are importantly dependent on and are closely related to hippocampal function (Deacon, Citation2006). It has been established that chronic stress causes lesions and damages in the hippocampus. In addition, several lines of evidence suggest that CRS would induce deficits in hippocampus memory by alterations of neural activity and synaptic plasticity (Huang et al., Citation2015; Kim & Diamond, Citation2002; Nasca et al., Citation2015; Wei et al., Citation2010). Consistent with these findings, we observed that CRS significantly caused deficits in spatial learning and memory with decreased Keap1/Nrf2 pathway in hippocampus in CRS mice. Moreover, it has been noted that Nrf2 knockout mice showed depression-like symptoms and increased Aβ production and worsen cognitive deficits (Bahn et al., Citation2019; Yao et al., Citation2016). Therefore, it seems that Keap1/Nrf2 pathway in the hippocampus may be indispensable in the cognitive dysfunction induced by CRS. However, further detailed studies regarding the effects of Sulforaphane (Nrf2 activator) on CRS-induced cognitive dysfunction are warranted.
Very recently, Hargis et al reported that ARS for 3 hours caused cognitive impairments in middle-aged Fischer mice (Hargis et al., Citation2018). By contrast, in this study, we revealed that escape latency and platform crossing in the MWMT showed no noticeable changes between ARS and control group. Although the exact mechanisms are elusive, the inconsistent results might be partially due to varying animal strains, model durations and strengths; further detailed studies are greatly needed.
ERS is pivotal for the synthesis, modification, and delivery of cellular protein in order to maintain cell homeostasis. Accumulating evidence demonstrated that overexpression of UPR signaling proteins correlate with several neurodegenerative diseases and hyperalgesia, influencing behaviors of pain sensitivity and cognitive function (Ii Timberlake & Dwivedi, Citation2019; Zhang et al., Citation2015). However, as presented in this study, we demonstrated that there were no significant differences in the proteins of the three UPR pathways in spinal cord and hippocampus of ARS and CRS mice, inconsistent with previously study (Zhang et al., Citation2014). The variations between the models may account for the differences, as they may have compensatory mechanisms in place. Further studies on the difference in protein levels of UPR signaling pathway are required.
The causal linkage between pain and abnormal functions in central nervous system has recently attracted increasing scientific attentions. Surgery, a stressful stimulus, combined with pain, hypothermia, aging and frailty, has a detrimental potential to induce cognitive dysfunction postoperatively (Czyż-Szypenbejl et al., Citation2019; Gong et al., Citation2018). Furthermore, pain and anhedonia (a key symptom of depression) might share a common pathogenesis and aggravate symptoms reciprocally (Yang et al., Citation2019). In this study, we demonstrated that changes in escape latency of MWMT were markedly correlated with mechanical threshold in CRS, whereas escape latency and TFL did not show any significant correlation. Consequently, stress-induced pain may be an aggravating factor for the onset of cognitive dysfunction.
In conclusion, this study suggests that both ARS and CRS alter pain behaviors, whereas only CRS affects cognitive dysfunction and activity of daily living, and the alteration of cognitive dysfunction in CRS may be associated with the Keap1/Nrf2 pathway. It is therefore likely that effective strategy targeting Keap1/Nrf2 pathway may contribute to prevent and treat hyperalgesia and cognitive dysfunction in CRS. This study provides a roadmap for further exploration in this field. However, additional research is still greatly needed.
Author contributions
NY: conceptualization; methodology; formal analysis; investigation; software; writing-original draft; visualization. YW: formal analysis; investigation; software; writing-original draft. XXL: conceptualization; writing-review and editing; supervision. GFZ: conceptualization; investigation; visualization; writing-review and editing; supervision; funding acquisition.
Disclosure statement
All the authors declared no conflict of interest.
Additional information
Funding
Notes on contributors
Ning Yang
Ning Yang received an M.S. degree in Anesthesiology from Huazhong University of Science and Technology in 2019, and a B.S. degree in Anesthesiology from Shanxi Medical College in 2016. Currently, he works as a resident physician in the Anesthesiology Department of Union Hospital in Wuhan, China. Her professional interests lie in the mechanism of stress-induced behavior.
Yue Wang
Yue Wang received a Ph.D. degree in Anesthesiology from Huazhong University of Science and Technology in 2023, and a B.S. degree in clinical medicine from Zhengzhou University in 2018. Currently, she works as a resident physician at the Fujian Medical College in Fuzhou, China. Her professional interests lie in perioperative brain protection.
Xiaoxiao Luo
Xiaoxiao Luo received a Ph.D. degree in Oncology, and a B.S. degree in clinical medicine from Huazhong University of Science and Technology. Currently, she works as an attending physician in the oncology Department of Tongji Hospital in Wuhan, China.
Gaofeng Zhan
Gaofeng Zhan received a Ph.D. degree in Anesthesiology from Huazhong University of Science and Technology in 2021, and a B.S. degree in clinical medicine from Nanchang University in 2016. Currently, he works as an attending physician in the Anesthesiology Department of Tongji Hospital in Wuhan, China. His professional interests lie in perioperative brain protection.
References
- Abdallah, C. G., & Geha, P. (2017). Chronic pain and chronic stress: Two sides of the same coin? Chronic Stress (Thousand Oaks, Calif.), 1, 1. https://doi.org/10.1177/2470547017704763
- Bahn, G., Park, J.-S., Yun, U. J., Lee, Y. J., Choi, Y., Park, J. S., Baek, S. H., Choi, B. Y., Cho, Y. S., Kim, H. K., Han, J., Sul, J. H., Baik, S.-H., Lim, J., Wakabayashi, N., Bae, S. H., Han, J.-W., Arumugam, T. V., Mattson, M. P., & Jo, D.-G. (2019). NRF2/ARE pathway negatively regulates BACE1 expression and ameliorates cognitive deficits in mouse Alzheimer’s models. Proceedings of the National Academy of Sciences of the United States of America, 116(25), 12516–11. https://doi.org/10.1073/pnas.1819541116
- Bardin, L., Malfetes, N., Newman-Tancredi, A., & Depoortère, R. (2009). Chronic restraint stress induces mechanical and cold allodynia, and enhances inflammatory pain in rat: Relevance to human stress-associated painful pathologies. Behavioural Brain Research, 205(2), 360–366. https://doi.org/10.1016/j.bbr.2009.07.005
- Branca, C., Ferreira, E., Nguyen, T. V., Doyle, K., Caccamo, A., & Oddo, S. (2017). Genetic reduction of Nrf2 exacerbates cognitive deficits in a mouse model of Alzheimer’s disease. Human Molecular Genetics, 26(24), 4823–4835. https://doi.org/10.1093/hmg/ddx361
- Castany, S., Gris, G., Vela, J. M., Verdú, E., & Boadas-Vaello, P. (2018). Critical role of sigma-1 receptors in central neuropathic pain-related behaviours after mild spinal cord injury in mice. Scientific Reports, 8(1), 3873. https://doi.org/10.1038/s41598-018-22217-9
- Chen, X., Green, P. G., & Levine, J. D. (2011). Stress enhances muscle nociceptor activity in the rat. Neuroscience, 185, 166–173. https://doi.org/10.1016/j.neuroscience.2011.04.020
- Cui, Y., Liao, X.-X., Liu, W., Guo, R.-X., Wu, Z.-Z., Zhao, C.-M., Chen, P.-X., & Feng, J.-Q. (2008). A novel role of minocycline: Attenuating morphine antinociceptive tolerance by inhibition of p38 MAPK in the activated spinal microglia. Brain, Behavior, and Immunity, 22(1), 114–123. https://doi.org/10.1016/j.bbi.2007.07.014
- Czyż-Szypenbejl, K., Mędrzycka-Dąbrowska, W., Kwiecień-Jaguś, K., & Lewandowska, K. (2019). The occurrence of postoperative cognitive dysfunction (POCD)—Systematic review. Psychiatria Polska, 53(1), 145–160. https://doi.org/10.12740/PP/90648
- Daudelin-Peltier, C., Forget, H., Blais, C., Deschênes, A., & Fiset, D. (2017). The effect of acute social stress on the recognition of facial expression of emotions. Scientific Reports, 7(1), 1036. https://doi.org/10.1038/s41598-017-01053-3
- De Gregorio, D., Inserra, A., Enns, J. P., Markopoulos, A., Pileggi, M., El Rahimy, Y., Lopez-Canul, M., Comai, S., & Gobbi, G. (2022). Repeated lysergic acid diethylamide (LSD) reverses stress-induced anxiety-like behavior, cortical synaptogenesis deficits and serotonergic neurotransmission decline. Neuropsychopharmacology: Official Publication of the American College of Neuropsychopharmacology, 47(6), 1188–1198. https://doi.org/10.1038/s41386-022-01301-9
- Deacon, R. M. (2006). Assessing nest building in mice. Nature Protocols, 1(3), 1117–1119. https://doi.org/10.1038/nprot.2006.170
- Dhingra, M. S., Dhingra, S., Kumria, R., Chadha, R., Singh, T., Kumar, A., & Karan, M. (2014). Effect of trimethylgallic acid esters against chronic stress-induced anxiety-like behavior and oxidative stress in mice. Pharmacological Reports: PR, 66(4), 606–612. https://doi.org/10.1016/j.pharep.2014.01.004
- Fong, H. K., Sands, L. P., & Leung, J. M. (2006). The role of postoperative analgesia in delirium and cognitive decline in elderly patients: A systematic review. Anesthesia and Analgesia, 102(4), 1255–1266. https://doi.org/10.1213/01.ane.0000198602.29716.53
- Fregni, F., Macedo, I. C., Spezia-Adachi, L. N., Scarabelot, V. L., Laste, G., Souza, A., Sanches, P. R. S., Caumo, W., & Torres, I. L. S. (2018). Transcranial direct current stimulation (tDCS) prevents chronic stress-induced hyperalgesia in rats. Brain Stimulation, 11(2), 299–301. https://doi.org/10.1016/j.brs.2017.11.009
- Gao, J., Xiong, B., Zhang, B., Li, S., Huang, N., Zhan, G., Jiang, R., Yang, L., Wu, Y., Miao, L., Zhu, B., Yang, C., & Luo, A. (2018). Sulforaphane alleviates lipopolysaccharide-induced spatial learning and memory dysfunction in mice: The role of BDNF-mTOR signaling pathway. Neuroscience, 388, 357–366. https://doi.org/10.1016/j.neuroscience.2018.07.052
- Gong, G. L., Liu, B., Wu, J. X., Li, J. Y., Shu, B. Q., & You, Z. J. (2018). Postoperative cognitive dysfunction induced by different surgical methods and its risk factors. The American Surgeon, 84(9), 1531–1537. https://doi.org/10.1177/000313481808400963
- Gu, H., Deng, X., Lv, Y., Chen, Q., & Yu, W. (2019). Preoperational chronic pain impairs the attention ability before surgery and recovery of attention and memory abilities after surgery in non-elderly patients. Journal of Pain Research, 12, 151–158. https://doi.org/10.2147/JPR.S178118
- Hargis, K., Buechel, H. M., Popovic, J., & Blalock, E. M. (2018). Acute psychosocial stress in mid-aged male rats causes hyperthermia, cognitive decline, and increased deep sleep power, but does not alter deep sleep duration. Neurobiology of Aging, 70, 78–85. https://doi.org/10.1016/j.neurobiolaging.2018.06.009
- Hetz, C., Zhang, K., & Kaufman, R. J. (2020). Mechanisms, regulation and functions of the unfolded protein response. Nature Reviews. Molecular Cell Biology, 21(8), 421–438. https://doi.org/10.1038/s41580-020-0250-z
- Howland, J. G., & Cazakoff, B. N. (2010). Effects of acute stress and GluN2B-containing NMDA receptor antagonism on object and object-place recognition memory. Neurobiology of Learning and Memory, 93(2), 261–267. https://doi.org/10.1016/j.nlm.2009.10.006
- Huang, N., Yang, C., Hua, D., Li, S., Zhan, G., Yang, N., Luo, A., & Xu, H. (2019). Alterations in the BDNF-mTOR signaling pathway in the spinal cord contribute to hyperalgesia in a rodent model of chronic restraint stress. Neuroscience, 409, 142–151. https://doi.org/10.1016/j.neuroscience.2019.03.052
- Huang, P., Li, C., Fu, T., Zhao, D., Yi, Z., Lu, Q., Guo, L., & Xu, X. (2015). Flupirtine attenuates chronic restraint stress-induced cognitive deficits and hippocampal apoptosis in male mice. Behavioural Brain Research, 288, 1–10. https://doi.org/10.1016/j.bbr.2015.04.004
- Ii Timberlake, M., & Dwivedi, Y. (2019). Linking unfolded protein response to inflammation and depression: Potential pathologic and therapeutic implications. Molecular Psychiatry, 24(7), 987–994. https://doi.org/10.1038/s41380-018-0241-z
- Imbe, H., Iwai-Liao, Y., & Senba, E. (2006). Stress-induced hyperalgesia: Animal models and putative mechanisms. Frontiers in Bioscience: A Journal and Virtual Library, 11(1), 2179–2192. https://doi.org/10.2741/1960
- Kim, J. J., & Diamond, D. M. (2002). The stressed hippocampus, synaptic plasticity and lost memories. Nature Reviews. Neuroscience, 3(6), 453–462. https://doi.org/10.1038/nrn849
- Kopp, M. C., Larburu, N., Durairaj, V., Adams, C. J., & Ali, M. M. U. (2019). UPR proteins IRE1 and PERK switch BiP from chaperone to ER stress sensor. Nature Structural & Molecular Biology, 26(11), 1053–1062. https://doi.org/10.1038/s41594-019-0324-9
- Li, D., Jing, D., Liu, Z., Chen, Y., Huang, F., & Behnisch, T. (2019). Enhanced expression of secreted alpha-klotho in the hippocampus alters nesting behavior and memory formation in mice. Frontiers in Cellular Neuroscience, 13, 133. https://doi.org/10.3389/fncel.2019.00133
- Li, S., Yang, C., Fang, X., Zhan, G., Huang, N., Gao, J., Xu, H., Hashimoto, K., & Luo, A. (2018). Role of Keap1-Nrf2 signaling in anhedonia symptoms in a rat model of chronic neuropathic pain: Improvement with sulforaphane. Frontiers in Pharmacology, 9, 887. https://doi.org/10.3389/fphar.2018.00887
- Marciniak, S. J., Chambers, J. E., & Ron, D. (2022). Pharmacological targeting of endoplasmic reticulum stress in disease. Nature Reviews. Drug Discovery, 21(2), 115–140. https://doi.org/10.1038/s41573-021-00320-3
- Mitsumoto, Y., & Mori, A. (2018). Acute restraint stress augments 1-Methyl-4-phenyl-1,2,3,6-tetrahydropyridine neurotoxicity via increased toxin uptake into the brain in C57BL/6 Mice. Neuroscience Bulletin, 34(5), 849–853. https://doi.org/10.1007/s12264-018-0254-2
- Muneer, A., & Shamsher Khan, R. M. (2019). Endoplasmic reticulum stress: Implications for neuropsychiatric disorders. Chonnam Medical Journal, 55(1), 8–19. https://doi.org/10.4068/cmj.2019.55.1.8
- Muramatsu, H., Katsuoka, F., Toide, K., Shimizu, Y., Furusako, S., & Yamamoto, M. (2013). Nrf2 deficiency leads to behavioral, neurochemical and transcriptional changes in mice. Genes to Cells: devoted to Molecular & Cellular Mechanisms, 18(10), 899–908. https://doi.org/10.1111/gtc.12083
- Musazzi, L., Sala, N., Tornese, P., Gallivanone, F., Belloli, S., Conte, A., Di Grigoli, G., Chen, F., Ikinci, A., Treccani, G., Bazzini, C., Castiglioni, I., Nyengaard, J. R., Wegener, G., Moresco, R. M., & Popoli, M. (2019). Acute inescapable stress rapidly increases synaptic energy metabolism in prefrontal cortex and alters working memory performance. Cerebral Cortex (New York, N.Y.: 1991), 29(12), 4948–4957. https://doi.org/10.1093/cercor/bhz034
- Nasca, C., Zelli, D., Bigio, B., Piccinin, S., Scaccianoce, S., Nisticò, R., & McEwen, B. S. (2015). Stress dynamically regulates behavior and glutamatergic gene expression in hippocampus by opening a window of epigenetic plasticity. Proceedings of the National Academy of Sciences of the United States of America, 112(48), 14960–14965. https://doi.org/10.1073/pnas.1516016112
- O’Gara, B. P., Gao, L., Marcantonio, E. R., & Subramaniam, B. (2021). Sleep, pain, and cognition: Modifiable targets for optimal perioperative brain health. Anesthesiology, 135(6), 1132–1152. https://doi.org/10.1097/ALN.0000000000004046
- Rafa-Zabłocka, K., Zelek-Molik, A., Tepper, B., Chmielarz, P., Kreiner, G., Wilczkowski, M., & Nalepa, I. (2021). Chronic restraint stress induces changes in the cerebral Galpha 12/13 and Rho-GTPase signaling network. Pharmacological Reports: PR, 73(4), 1179–1187. https://doi.org/10.1007/s43440-021-00294-4
- Raghavendra, V., Rutkowski, M. D., & DeLeo, J. A. (2002). The role of spinal neuroimmune activation in morphine tolerance/hyperalgesia in neuropathic and sham-operated rats. The Journal of Neuroscience: The Official Journal of the Society for Neuroscience, 22(22), 9980–9989. https://doi.org/10.1523/JNEUROSCI.22-22-09980.2002
- Ramsey, C. P., Glass, C. A., Montgomery, M. B., Lindl, K. A., Ritson, G. P., Chia, L. A., Hamilton, R. L., Chu, C. T., & Jordan-Sciutto, K. L. (2007). Expression of Nrf2 in neurodegenerative diseases. Journal of Neuropathology and Experimental Neurology, 66(1), 75–85. https://doi.org/10.1097/nen.0b013e31802d6da9
- Reinhardt, T., Kleindienst, N., Treede, R. D., Bohus, M., & Schmahl, C. (2013). Individual modulation of pain sensitivity under stress. Pain Medicine (Malden, Mass.), 14(5), 676–685. https://doi.org/10.1111/pme.12090
- Robson, J. G., Davenport, H. T., & Sugiyama, R. (1965). Differentiation of Two Types of Pain by Anesthetics. Anesthesiology, 26(1), 31–36. https://doi.org/10.1097/00000542-196501000-00006
- Suri, P., Boyko, E. J., Smith, N. L., Jarvik, J. G., Jarvik, G. P., Williams, F. M. K., Williams, R., Haselkorn, J., & Goldberg, J. (2019). Post-traumatic stress disorder symptoms are associated with incident chronic back pain: A longitudinal twin study of older male veterans. Spine, 44(17), 1220–1227. https://doi.org/10.1097/BRS.0000000000003053
- Wang, W., Guan, C., Sun, X., Zhao, Z., Li, J., Fu, X., Qiu, Y., Huang, M., Jin, J., & Huang, Z. (2016). Tanshinone IIA protects against acetaminophen-induced hepatotoxicity via activating the Nrf2 pathway. Phytomedicine: International Journal of Phytotherapy and Phytopharmacology, 23(6), 589–596. https://doi.org/10.1016/j.phymed.2016.02.022
- Weger, M., & Sandi, C. (2018). High anxiety trait: A vulnerable phenotype for stress-induced depression. Neuroscience and Biobehavioral Reviews, 87, 27–37. https://doi.org/10.1016/j.neubiorev.2018.01.012
- Wei, L., David, A., Duman, R. S., Anisman, H., & Kaffman, A. (2010). Early life stress increases anxiety-like behavior in Balb c mice despite a compensatory increase in levels of postnatal maternal care. Hormones and Behavior, 57(4-5), 396–404. https://doi.org/10.1016/j.yhbeh.2010.01.007
- Woo, H., Hong, C. J., Jung, S., Choe, S., & Yu, S. W. (2018). Chronic restraint stress induces hippocampal memory deficits by impairing insulin signaling. Molecular Brain, 11(1), 37. https://doi.org/10.1186/s13041-018-0381-8
- Yamamoto, M., Kensler, T. W., & Motohashi, H. (2018). The KEAP1-NRF2 system: A thiol-based sensor-effector apparatus for maintaining redox homeostasis. Physiological Reviews, 98(3), 1169–1203. https://doi.org/10.1152/physrev.00023.2017
- Yang, C., Fang, X., Zhan, G., Huang, N., Li, S., Bi, J., Jiang, R., Yang, L., Miao, L., Zhu, B., Luo, A., & Hashimoto, K. (2019). Key role of gut microbiota in anhedonia-like phenotype in rodents with neuropathic pain. Translational Psychiatry, 9(1), 57. https://doi.org/10.1038/s41398-019-0379-8
- Yang, C., Qu, Y., Fujita, Y., Ren, Q., Ma, M., Dong, C., & Hashimoto, K. (2017). Possible role of the gut microbiota-brain axis in the antidepressant effects of (R)-ketamine in a social defeat stress model. Translational Psychiatry, 7(12), 1294. https://doi.org/10.1038/s41398-017-0031-4
- Yang, Z., Li, C., Wang, Y., Yang, J., Yin, Y., Liu, M., Shi, Z., Mu, N., Yu, L., & Ma, H. (2018). Melatonin attenuates chronic pain related myocardial ischemic susceptibility through inhibiting RIP3-MLKL/CaMKII dependent necroptosis. Journal of Molecular and Cellular Cardiology, 125, 185–194. https://doi.org/10.1016/j.yjmcc.2018.10.018
- Yao, W., Zhang, J.-C., Ishima, T., Dong, C., Yang, C., Ren, Q., Ma, M., Han, M., Wu, J., Suganuma, H., Ushida, Y., Yamamoto, M., & Hashimoto, K. (2016). Role of Keap1-Nrf2 signaling in depression and dietary intake of glucoraphanin confers stress resilience in mice. Scientific Reports, 6(1), 30659. https://doi.org/10.1038/srep30659
- Yuede, C. M., Timson, B. F., Hettinger, J. C., Yuede, K. M., Edwards, H. M., Lawson, J. E., Zimmerman, S. D., & Cirrito, J. R. (2018). Interactions between stress and physical activity on Alzheimer’s disease pathology. Neurobiology of Stress, 8, 158–171. https://doi.org/10.1016/j.ynstr.2018.02.004
- Zhan, G., Hua, D., Huang, N., Wang, Y., Li, S., Zhou, Z., Yang, N., Jiang, R., Zhu, B., Yang, L., Yu, F., Xu, H., Yang, C., & Luo, A. (2019). Anesthesia and surgery induce cognitive dysfunction in elderly male mice: The role of gut microbiota. Aging, 11(6), 1778–1790. https://doi.org/10.18632/aging.101871
- Zhang, E., Yi, M.-H., Shin, N., Baek, H., Kim, S., Kim, E., Kwon, K., Lee, S., Kim, H.-W., Chul Bae, Y., Kim, Y., Kwon, O.-Y., Lee, W. H., & Kim, D. W. (2015). Endoplasmic reticulum stress impairment in the spinal dorsal horn of a neuropathic pain model. Scientific Reports, 5(1), 11555. https://doi.org/10.1038/srep11555
- Zhang, J. C., Yao, W., Dong, C., Han, M., Shirayama, Y., & Hashimoto, K. (2018). Keap1-Nrf2 signaling pathway confers resilience versus susceptibility to inescapable electric stress. European Archives of Psychiatry and Clinical Neuroscience, 268(8), 865–870. https://doi.org/10.1007/s00406-017-0848-0
- Zhang, W.-J., Cao, W.-Y., Huang, Y.-Q., Cui, Y.-H., Tu, B.-X., Wang, L.-F., Zou, G.-J., Liu, Y., Hu, Z.-L., Hu, R., Li, C.-Q., Xing, X.-W., & Li, F. (2019). The role of miR-150 in stress-induced anxiety-like behavior in mice. Neurotoxicity Research, 35(1), 160–172. https://doi.org/10.1007/s12640-018-9943-x
- Zhang, Y., Liu, W., Zhou, Y., Ma, C., Li, S., & Cong, B. (2014). Endoplasmic reticulum stress is involved in restraint stress-induced hippocampal apoptosis and cognitive impairments in rats. Physiology & Behavior, 131, 41–48. https://doi.org/10.1016/j.physbeh.2014.04.014