Abstract
Both obesity and high fat diets (HFD) have been associated with an increase in inflammatory gene expression within the brain. Microglia play an important role in early cortical development and may be responsive to HFD, particularly during sensitive windows, such as adolescence. We hypothesized that HFD during adolescence would increase proinflammatory gene expression in microglia at baseline and potentiate the microglial stress response. Two stressors were examined, a physiological stressor [lipopolysaccharide (LPS), IP] and a psychological stressor [15 min restraint (RST)]. From 3 to 7 weeks of age, male and female mice were fed standard control diet (SC, 20% energy from fat) or HFD (60% energy from fat). On P49, 1 h before sacrifice, mice were randomly assigned to either stressor exposure or control conditions. Microglia from the frontal cortex were enriched using a Percoll density gradient and isolated via fluorescence-activated cell sorting (FACS), followed by RNA expression analysis of 30 genes (27 target genes, three housekeeping genes) using Fluidigm, a medium throughput qPCR platform. We found that adolescent HFD induced sex-specific transcriptional response in cortical microglia, both at baseline and in response to a stressor. Contrary to our hypothesis, adolescent HFD did not potentiate the transcriptional response to stressors in males, but rather in some cases, resulted in a blunted or absent response to the stressor. This was most apparent in males treated with LPS. However, in females, potentiation of the LPS response was observed for select proinflammatory genes, including Tnfa and Socs3. Further, HFD increased the expression of Itgam, Ikbkb, and Apoe in cortical microglia of both sexes, while adrenergic receptor expression (Adrb1 and Adra2a) was changed in response to stressor exposure with no effect of diet. These data identify classes of genes that are uniquely affected by adolescent exposure to HFD and different stressor modalities in males and females.
Introduction
Chronic consumption of a high fat diet (HFD) has been linked to adverse health outcomes, including obesity, diabetes, and cardiovascular disease (Astrup et al., Citation2008), as well as exacerbation of neurodegenerative diseases (Fakih et al., Citation2022) and cognitive deficits (Beilharz et al., Citation2015). In animal models, both acute (days) and chronic (weeks) consumption of HFD have been shown to alter behavior, including reward-related behaviors, social behaviors, and cognition (Carlin et al., Citation2016; Dantzer et al., Citation2008; Lasselin et al., Citation2020; Spencer et al., Citation2017). HFD-induced inflammation has been linked to many of these adverse outcomes, and HFD-induced proinflammatory responses have been documented in the brain (Alexaki, Citation2021; Butler, Citation2021; Butler et al., Citation2020; Kim et al., Citation2019; Milanova et al., Citation2019; Paolicelli & Angiari, Citation2019).
In both rodents and humans, the development of the prefrontal cortex (PFC) extends through adolescence (Caballero et al., Citation2016), which renders this area of the brain particularly vulnerable to environmental exposures during childhood and adolescence. In a study that compared 16 weeks of HFD exposure starting in adolescence or adulthood, adolescent—but not adult—onset HFD was shown to potentiate hippocampal inflammatory responses (Il1b and Tnfa responses to LPS injection) (Boitard et al., Citation2014; Vinuesa et al., Citation2016). In a report of 6-week HFD exposure beginning at postnatal day (P21), HFD exposed mice showed deficits in social preference and social recognition, that were not related to weight gain. Further, increases in Il1b, Il6, and senescence-related gene expression in the PFC were observed in microglia and astrocytes, but not oligodendrocytes or neurons (Yang et al., Citation2020). Similarly, increases in Itgam, Cx3cr1, Nlrp3, and Il1b mRNA expression were shown in microglia isolated from male rat hippocampus after only 3 days on HFD (Butler et al., Citation2020), suggesting that these changes in microglial function may be due to the diet itself, and not necessarily subsequent alterations to adiposity or body mass.
The goal of the present experiments was to assess the effect of HFD on microglial gene expression in the PFC, both at baseline, as well as in response to either a physiological (LPS) or a psychological stressor (acute restraint). Stressors can be differentiated based on the nature of the sensory input that drives the challenge, the extent to which they invoke an affective response, and the circuitry that mediates certain adaptive responses to them (Dayas et al., Citation2001; Herman & Cullinan, Citation1997; Sawchenko et al., Citation1996, Citation2000), and both categories of stressors have been found to engage microglia. Here, we assessed a broad panel of microglial genes critical in inflammatory signaling, stress responsivity, and homeostatic maintenance. Microglia express a variety of “sensome” receptors, including P2ry12, Csf1r, Tgfbr1, Cx3cr1, CD68, CD14, TLR4, Itgam, Slc2a5, and Tmem119, which allow them to dynamically survey their microenvironment and engage with homeostatic threats (e.g. microbes, fluctuations in neuronal activity) (Hickman et al., Citation2013). Genes related to the stress response were also examined, including glucocorticoid (GR) and mineralocorticoid (MR) receptors, and beta-adrenergic receptors (β-AR). We hypothesized that HFD exposure would increase inflammatory markers in microglia and potentiate mRNA expression of inflammatory factors stimulated by either LPS or acute restraint. Additionally, we hypothesized that female microglia would be less reactive than males, based on prior reports that HFD increases microglia count specifically in males (Cai et al., Citation2014) and that female microglia exhibit a more ramified morphology early in development (Schwarz et al., Citation2012).
Methods
Housing and conditions
Female C57BL/6J mice (stock number 000664, Jackson) were mated to male DBA mice (stock number 000671, Jackson), and male and female offspring were used for this study. Litter size ranged from 5 to 9 animals/litter, and pups were randomly assigned to experimental groups. This hybrid strategy enhances genetic diversity to increase the generalizability of our findings. Animals were housed in a climate-controlled facility with a 12:12 light-dark cycle (lights on at 0700). Food (LabDiet #5053, St. Louis, MO, USA, except for experimental diet conditions detailed below) and water were available ad libitum. Animals were cared for in accordance with the NIH guidelines for Care and Use of Animals and the University of Cincinnati Institutional Animal Care and Use Committee.
Experimental groups
At weaning, offspring were housed with non-siblings in groups of 3 or 4 and randomly assigned to one of two diet conditions. Control animals (SC; standard control) were fed a diet containing 20% energy from fat (TestDiet 5755, St. Louis, MO, USA), while high fat diet fed animals (HFD) received a diet containing 60% energy from fat (TestDiet 58G9, St. Louis, MO, USA). The diets were micronutrient matched and both contained 19% energy from protein. Animals remained on the diets for the entirety of the study. Body weight was measured weekly (Supplemental Figure 1). In separate experiments, animals were exposed to two different stressors, LPS injection or restraint, both of which reliably activate both the hypothalamic-pituitary-adrenal axis and sympathetic nervous system stress-responsive pathways. See for the experimental timeline.
Figure 1. Experimental timeline. High fat diet or control diet was administered from 3 to 7 weeks of age. On the experimental day, stressor exposure (LPS injection or 15′ RST) occurred 1 h before brain collection/microglial isolation/RNA preparation.
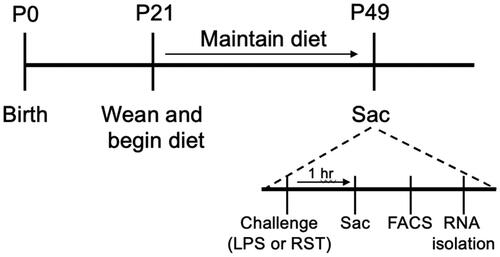
Experiment 1: LPS challenge and sacrifice
At 7 weeks of age, animals (N = 8 per group, per sex) were randomly assigned to receive either an injection of lipopolysaccharide (LPS) (10 μg in 100 μL, i.p., Sigma-Aldrich L2880) or 0.9% sterile saline (100 µl) 1 h before sacrifice by cervical dislocation. The brain was then removed and rapidly dissected. To obtain a PFC-enriched sample, a razor blade was used to make two coronal cuts, one to remove the olfactory bulbs, and a second behind the PFC (before corpus callosum joining; see Figure S2).
Experiment 2: Restraint stress challenge and sacrifice
Between 6.5 and 7.5 weeks of age animals were randomly assigned to receive either an acute stress (15-min RST) in a hard plastic restrainer and then returned to the home cage) 1 h before sacrifice by cervical dislocation or to serve as undisturbed controls where animals remained in their home cage. Group sizes: Male: control/control n = 5, HFD/control n = 6, control/RST n = 7, HFD/RST n = 7; female: control/control n = 4, HFD/control n = 7, control/RST n = 7, HFD/RST n = 7. Brains were dissected as described above and stored in PBS.
Microglia enrichment and fluorescence activated cell sorting (FACS)
Percoll gradient enrichment of microglia and FACS were performed as previously described (Bollinger et al., Citation2020). Tissue samples were immediately stored on ice, with all subsequent procedures performed at 4 °C. Both mechanical tissue homogenization and reduced temperatures (4 °C) have been shown to limit isolation-associated shifts in microglial gene expression (Marsh et al., Citation2022; Ocañas et al., Citation2022). Therefore, dissected frontal cortex was passed through a 70 µm nylon mesh cell strainer (BD Falcon 35-2350) and mixed with 10 mL PBS, then centrifuged at 800 g for 8 min at 4 °C. The pellet was then resuspended in 70% isotonic Percoll (SIP, GE Healthcare, Uppsala, Sweden), and then layered with 30 and 0% Percoll (PBS). The gradient was centrifuged at 2000 g for 20 min at 4 °C. Enriched microglia were collected from the interface between the 70 and 30% Percoll layers and washed in PBS. Cells were then incubated with conjugated antibodies (PerCP-Cy5.5 Rat Anti-CD11b and PE-CF594 Rat Anti-Mouse CD45, BD Biosciences) at 4 °C for 1 h. Microglial cells collected were CD11b+/CD45low as seen in the representative gating graphs (Figure S2). Using CD45 as a secondary marker ensures that we exclude macrophage populations as their expression pattern is CD11b+/CD45high, as previously published (Bollinger et al., Citation2020; Horchar & Wohleb, Citation2019; Wohleb et al., Citation2018, Bollinger et al., Citation2024). The proportion of CD11b+/CD45low microglia collected relative to the total number of events did not differ between experimental groups. The cells were then washed and resuspended in FACS buffer. Approximately 30,000 microglia were collected per sample using a BioRad S3e four-color cytometer/cell sorter (Hercules, CA, USA).
Fluidigm
RNA was extracted using the Norgen Single Cell RNA Purification Kit (51800). cDNA was prepared using the Applied Biosystems High-Capacity cDNA Kit. Preamplification was run according to manufacturer’s directions (see Supplemental Table 1 for gene lists) (Fluidigm, South San Francisco, CA, USA) and cDNA was analyzed on a 96.96 Dynamic Array Integrated Fluidic Circuit on the Biomark HD machine (Fluidigm), a high-performance PCR system that can analyze 96 samples × 96 markers (in this case 30 genes in triplicate).
Analysis
Gene expression was normalized to the geomean of two housekeeping genes from the control group using the 2ΔΔCT method. Actb, Gapdh, Ppia, and Ywhaz were included in both experiments as potential housekeepers. For each set of data (experiment and sex), Normfinder (Andersen et al., Citation2004) was used to identify stable housekeeping genes, such that there were no main effects or interactions in the expression of the housekeeping genes.
Gene expression normalization
Gene expression normalization and analysis were completed within each sex independently for both experiments. Both sexes of Experiment 1 were normalized to the geomean of Gapdh and Ppia in the SC/saline group of the same sex. In Experiment 2, male gene expression was normalized to the geomean of Gapdh and Ppia in the SC/undisturbed group, and female gene expression was normalized to the geomean of Ppia and Ywhaz in the SC/undisturbed group. Within each sex, 2-way ANOVAs were conducted (factors of diet and stressor) on log-transformed fold change data. Planned post-hoc comparisons were used to clarify interactions (1-control/control vs. control/stressor, 2-control/control vs. HFD/control, 3- HFD/control vs. HFD/stressor). Outliers were identified using the methods: 1.5 IQR and 1.96 mean and standard deviation. Outliers removed were one male Il6 con/RST, one female con/RST for the following genes [Ikbkb, Cd14, lipoprotein lipase (Lpl), apolipoprotein e (Apoe), Itgam, Socs3]. Body weights were analyzed using 2-way repeated measures ANOVAs (factors of diet and time) followed by Sidak’s multiple comparisons test. Two-way ANOVAs were conducted using GraphPad Prism 9. p ≤ 0.05 was considered significant. Fold change values are expressed as mean ± SEM.
Heatmap preparation and clustering
Heatmaps were prepared using Morpheus (https://software.broadinstitute.org/morpheus). Fold change values of individual samples were log-transformed and then averaged by condition and sex. Spearman Rank correlations were used to cluster the samples by genes which identified 6–7 clusters per experiment.
Results
Expression of 27 gene targets was investigated, and the response patterns differed by sex and stressor. provides an overview of genes for which a significant interaction or main effect (for stress or diet) was identified, and the degree to which there was overlap in the male and female response. With regard to stress × diet interactions, these were most evident in male animals exposed to LPS, while P2ry12 demonstrated the only RST × diet interaction. Notably, there were more genes that showed a stress × diet interaction in males (18) vs. females (6). In terms of stressor effects, females had twice as many genes with a main effect of LPS, while the main effects of RST stress were largely observed in males. The main effects of diet were mostly seen in the RST experiment, owing in part to the interactions observed in the LPS experiment. Five genes were unchanged in all sexes and conditions in both experiments (Cox2, Cybb, Nos2, Nr3c2, Tlr4).
Figure 2. Venn diagrams showing ANOVA interactions (stress × diet) and main effects (stress or diet) in males and females. LPS treatment resulted in more stress × diet interactions in males vs. females, and more in LPS vs. restraint (only P2ry12). Main effects of stress or diet differed by sex, with main effects of stress or diet largely observed in males in response to restraint (LPS: lipopolysaccharide; RST: restraint). For main effects, arrows indicate up- or down-regulated genes.
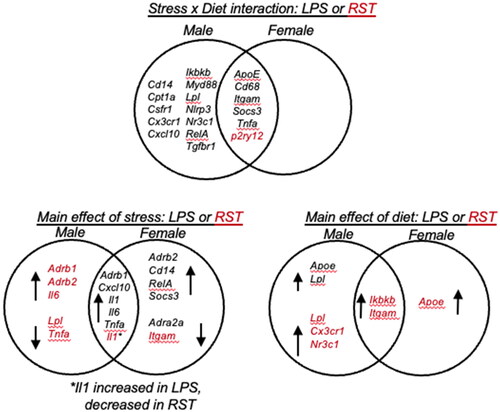
Microglia from males exposed to adolescent HFD are non-responsive to LPS
To better understand the effects of stress and diet across genes and sexes, patterns of gene expression were clustered and visualized in a heatmap (). The largest cluster in the LPS experiment (A) was defined by the HFD × LPS interaction, such that in males both LPS and HFD increased gene expression, however, the response to LPS was absent in HFD-fed mice (cluster 1; light green bar). This response pattern is illustrated with two examples, Tnfa and Socs3 (, see for full statistics and Supplemental Figure 3 for additional examples of significant interactions). In male mice fed the control diet, LPS injection or HFD independently led to an increase in gene expression. However, in mice fed the HFD during adolescence, the response to LPS was absent. Notably, a nearly opposite pattern of gene expression was observed in females. For Tnfa and Socs3, LPS did not change the expression in control-fed females and HFD alone led to a decrease in gene expression. However, in females fed the HFD during adolescence, LPS induced a significant increase in the expression of both genes.
Figure 3. (A,B) Heatmap of gene expression changes in isolated microglia from LPS experiment (A, left) and restraint experiment (B, right). Genes are clustered by similarities in response patterns, with different colored bars to the right of the gene names indicating distinct clusters. The scale is log fold change. Cluster 1: genes increased by LPS and HFD in males only; cluster 2: HFD alone increases expression in males; cluster 3: HFD increases expression in both sexes; cluster 4: stress effect increases expression in LPS, decreases expression in RST across both sexes.
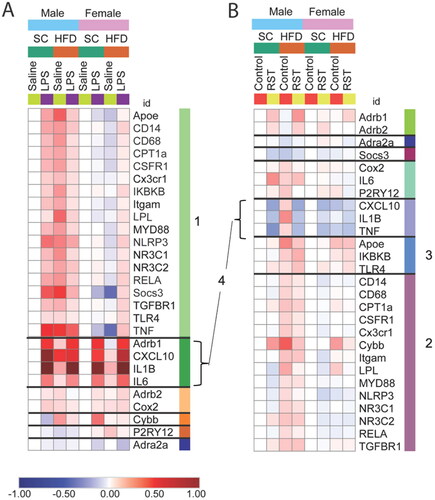
Figure 4. Diet and stressor (LPS) interact to affect Tnfa and Socs3 gene expression. (A,C) Tnfa (top) and Socs3 (bottom) gene expression in males was increased by HFD and LPS exposure separately, and HFD-fed males failed to increase gene expression in response to LPS. (B,D) In females, Tnfa and Socs3 gene expression decreased by HFD compared to controls, while HFD-fed females had an increase in gene expression, not seen in control-fed animals (HFD: high fat diet; LPS: lipopolysaccharide) (# interaction between diet and LPS, Graph legend = * main effect of stress; *p < 0.05, ***p < 0.001).
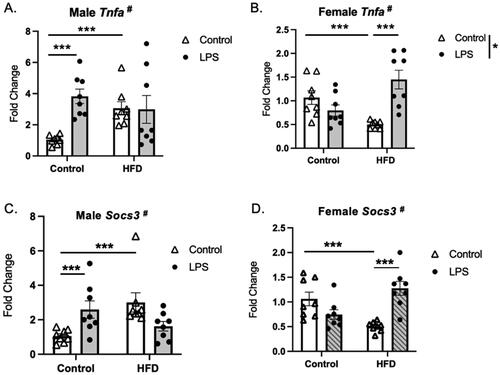
Table 1. Statistical results of gene expression analyses.
HFD alone increases gene expression
A second notable pattern is a main effect of diet within the RST experiment ( and ), seen more often in males (cluster 2, purple bar) than in both sexes (cluster 3, medium blue bar). In all cases where a main effect of diet was detected, the direction of the effect was such that HFD increased the expression of the transcript. Examples are shown in , with Itgam and Lpl from cluster 2 and Apoe from cluster 3.
Stressor specific effects on gene transcription
Regardless of sex or diet, exposure to LPS led to an increase in the expression of proinflammatory molecules Il1b, Il6, Cxcl10, and Tnfa (Cluster 4, , Il1b shown as an example). In contrast, mRNA levels of Il1b (, male and female) and Tnfa and Cxcl10 (males only, Supplemental Figure 5) were decreased following RST.
Figure 6. Gene expression of IL1β in response to stressor exposure. (A) Male and (B) female mice exposed to LPS show an increased expression Il1b. (C) Male and (D) female mice exposed to restraint stress show a significant decrease in Il1b expression. HFD did not affect the Il1b response to stress (HFD: high fat diet; LPS: lipopolysaccharide) (Graph legend = Control HFD * main effect of diet; *p < 0.05, **p < 0.01, ***p < 0.001).
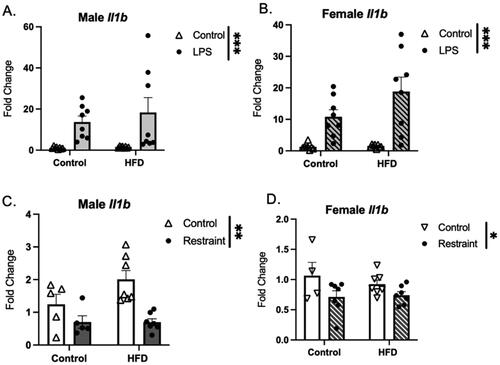
Stress signaling gene expression is responsive to stressors
The expression patterns of genes related to stress signaling were also impacted by stressor and diet (). Transcript levels of Adrb1 were increased by LPS (both sexes) and RST (males) only, regardless of adolescent diet, while expression of Adra2a was decreased by LPS (females only). Further, expression of Adrb2 was also increased by RST in males only. GR expression levels were altered in males only, with HFD leading to an increase in expression in both experiments (Supplemental Figure 6).
Figure 7. Stressor effects on adrenergic receptor expression. (A,B) Adrb1 expression was increased in response to LPS (A-males and B-females) and restraint (males only-E), and this response was not affected by HFD exposure. (C,D) Adra2a expression was decreased in females exposed to LPS (D), but not males. (F) Adrb2 was increased in males in response to RST (HFD: high fat diet; LPS: lipopolysaccharide; *p < 0.01, **p < 0.01, ***p < 0.001).
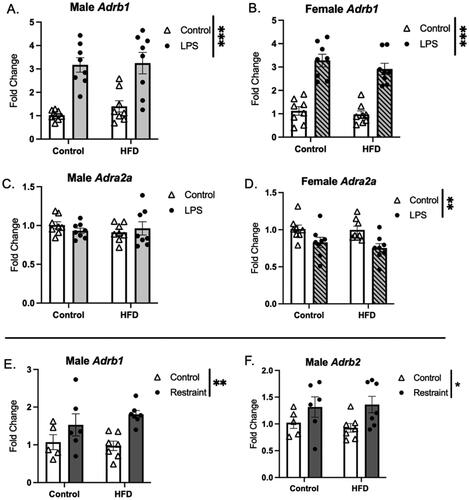
Discussion
In the present studies, we found that adolescent HFD can alter the transcriptional response of microglia to a stressor and that these effects vary by sex. Particularly for proinflammatory genes, adolescent HFD blunted microglial responses to LPS but potentiated this response in females. Further, RST alone affected more genes in male microglia vs. female microglia.
Adolescent HFD alone increases gene expression in males more so than females
In the LPS experiment, HFD in males increased expression of a wide range of genes, including those related to the microglial sensome (Csf1r, Tgfbr1, Itgam, Cd68, Cd14) and inflammatory responses (Nlrp3, Socs3, Rela) that were unaffected by HFD exposure in females. In the RST experiment, there were a few genes that were increased by HFD in both sexes: Itgam, Ikbkb, and Apoe. Itgam codes for one subunit of the complement receptor CR3, which is a cell surface receptor that recognizes and binds to complement proteins, part of the innate immune system that helps to clear pathogens and cellular debris. In addition to its role in innate immunity, CR3 also plays a role in synaptic pruning during development and regulation of neuronal activity (Schafer et al., Citation2012). And across both experiments, there were several genes that were similarly increased by HFD (Apoe, Ikbkb, Itgam, Lpl, Nr3c1). Genes in the NF-κB pathway were also affected by HFD, including Ikbkb (activates NF-κB via phosphorylation of IkB inhibitors) which was increased in all groups except females in the LPS experiment and Rela (males only), an NF-κB subunit. Genes that play a role in lipid metabolism were also affected by HFD exposure, including Apoe, which was increased in all groups except for the LPS females. Apoe is involved in lipid metabolism and cholesterol transport and upregulation of Apoe is observed in disease-associated microglia (DAM) (Deczkowska et al., Citation2018; Keren-Shaul et al., Citation2017), particularly in the context of Alzheimer’s disease and/or other neurodegenerative disorders (Krasemann et al., Citation2017). Further, Apoe levels are positively correlated with disease severity in mouse models of EAE, SOD1, and APP-PS1 (Krasemann et al., Citation2017). Additionally, Lpl was also induced by HFD (in males only in both experiments). Lpl is an enzyme involved in lipid metabolism and has been shown to be essential in the maintenance of microglial function upon exposure to a high carbohydrate/high fat diet (Gao et al., Citation2017). Collectively, these data highlight the broad responsiveness of cortical microglia to HFD, with effects more prominently seen in males.
Stressor specific effects on gene transcription
Exposure to LPS or RST induced opposing effects on the expression of proinflammatory cytokines in both males and females. For instance, LPS significantly upregulated microglial Il1b expression in the PFC in both sexes, whereas acute RST stress reduced levels of Il1b transcript. LPS has been shown to induce proinflammatory cytokines (Il1b, Cxcl10, Tnfa) in the PFC (Gibb et al., Citation2008; Makinson et al., Citation2017; Osborne et al., Citation2017), and in microglia specifically (Wohleb et al., Citation2012). Likewise, most of the research that has examined proinflammatory changes following restraint stress have focused on the hippocampus, hypothalamus, or the amygdala (McWhirt et al., Citation2019; Reyes et al., Citation2003; Sathyanesan et al., Citation2017). The current results are consistent with others in the literature that have examined the effect of acute stress on IL-1B in the PFC [e.g. decreased IL-1B protein in PFC after 10 min restraint (Gądek-Michalska et al., Citation2011)]. Interestingly, the effect of acute stress on Il1b appears to be region dependent, with stress increasing Il1b expression in HPC (Iwata et al., Citation2016). Further, the duration of stress [acute vs. chronic (Lovelock & Deak, Citation2018)] and strain differences (Porterfield et al., Citation2011) have also been shown to affect the Il1b response to stressors.
HFD affected microglial response to LPS differently depending on sex
Microglia from both males and females increased expression of specific proinflammatory genes in response to in vivo LPS administration, including Il1b, Il6, and Cxcl10. Additionally, in males, LPS led to the upregulation of genes related to the microglial sensome (e.g. Cd14, Cd68, Csf1r, Tgfbr1, Cx3cr1, Itgam), as well as inflammasome-related genes (e.g. Ikbkb, Myd88, Nlrp3, Rela, and Socs3), however, this response was less robust in females (). These findings (in males) are consistent with other reports of LPS-induced microglial specific gene expression changes (Hirbec et al., Citation2018). Interestingly, the proinflammatory cytokine response was not affected by HFD in either males or females, however, in HFD-fed males, a number of the sensome and inflammasome-related genes showed a blunted or absent response to LPS. Interestingly, in females, a very different pattern was observed, such that HFD consumption led to an exaggerated response for certain microglial genes (Tnfa, Cd68, and Socs3). A potentiating effect of HFD on LPS-induced cytokine expression has been observed repeatedly in hippocampus (Boitard et al., Citation2014; Sobesky et al., Citation2016) in male animals, and it has also been reported that exposure to HFD for 3 days (in adulthood) potentiated the neuronal response to LPS (cfos expression in the PVN) in both male and female rats (Cai et al., Citation2014). These data add to the literature and indicate that the potentiating effect of HFD on LPS-induced gene expression varies by both brain region and sex.
Stress signaling genes are responsive to both physiological and psychological stressors
Microglia express several receptors for stress-related neurotransmitters and hormones, including AR’s, GR’s, and MR’s (Johnson et al., Citation2012; Ramirez et al., Citation2017; Sugama et al., Citation2019; Tanaka et al., Citation2002; Wang et al., Citation2010; Xu et al., Citation2018); these receptors bind the stress-responsive molecules epinephrine/norepinephrine and corticosterone, respectively. Irrespective of diet, the β1-AR (Adrb1) was increased by both stressors in males and by LPS in females and β2-AR by restraint in males. The effect of β-AR activation on inflammatory responses is complex, with evidence to support both proinflammatory (Johnson et al., Citation2012; Sugama et al., Citation2019) or anti-inflammatory responses (Ishii et al., Citation2015; Xu et al., Citation2018) depending on the experimental context. Expression of α2A-AR (Adra2a) was decreased by LPS, but only in females, and this response was also not affected by HFD. Activation of α2A-AR has been associated with a largely anti-inflammatory profile. The downregulation of α2A-AR gene expression could indicate that microglia were more activated and/or primed, as α2A-AR blockade with yohimbine increases microglial activation [e.g. retraction of processes (Sugama et al., Citation2019)]. These findings would be in line with our data showing increased LPS-induced microglial proinflammatory cytokine expression in females. As opposed to the AR’s, GR expression was increased by HFD in males only. The role of microglial GR is not yet fully described, as studies using a microglia-specific GR knockout indicate an increase in inflammatory responses to intraparenchymal LPS (Carrillo-de Sauvage et al., Citation2013), yet a reduction in proinflammatory gene expression following chronic mild stress (in females only) (Picard et al., Citation2021). Understanding the consequence of increased GR expression by HFD in adolescence will require further study and will likely be influenced by age and condition (e.g. baseline, in response to psychological stress, or in response to an inflammatory challenge).
Sex specific microglial responses
We identified several sex differences in the current study, with female microglia showing overall fewer transcriptional changes in response to stress or HFD as compared to male microglia. Sex differences in the transcriptional profiles of male and female microglia have been well documented (Bollinger & Wohleb, Citation2019; Guneykaya et al., Citation2018), and result from both chromosomal sex and sex hormone-dependent mechanisms (VanRyzin et al., Citation2020). Estrogens have well characterized anti-inflammatory properties within the brain (Sárvári et al., Citation2012; Vegeto et al., Citation2006), and in fact, estradiol treatment has been shown to reverse HFD-induced microglial changes in females (Butler et al., Citation2020). Furthermore, both estradiol and testosterone can modulate basal aspects of microglial morphology in the medial PFC alongside microglial responses to psychological stressors (Bollinger & Wohleb, Citation2019). During the time of HFD exposure (P21–49), estradiol and testosterone levels are still increasing to reach their eventual adult levels. Given that microglia are highly attuned to their microenvironment, it is likely that significant fluctuations in sex hormones exert various effects on microglial phenotype and function during this sensitive developmental window. Indeed, future studies examining how sex hormones impact microglial responses to HFD, immune stimulation, and psychological stress during adolescence are warranted.
Conclusions, limitations, and future directions
The present study shows that HFD exposure spanning the time of adolescence alters the transcriptional response of microglia in the PFC following different stressors. Microglia from male mice were more affected by HFD, which resulted in a blunted transcriptional response to LPS. Females were overall less affected by HFD but did show instances of potentiated transcriptional responses to LPS. Of note, a limitation of the current study is that the two control groups in the RST experiment had small sample sizes (n = 4–5). It is important to evaluate studies with small sample sizes with caution, recognizing the limitations of generalizability and the importance of future replication studies. It would also be valuable for future studies to include a direct comparison with adult exposure to HFD, to determine similarities and differences of exposures during these different time windows, as time of exposure to HFD has differential effects on the brain, and neuroimmune responses are known to differ between adolescent and adult animals (Boitard et al., Citation2012). Additionally, investigations into how HFD affects the peripheral immune responses would illuminate whether the observed effects are specific to microglia or represent a broader effect on the immune system. A study with male rats did not find an effect of adolescent HFD on basal or stimulated cytokine levels in plasma (Doremus-Fitzwater et al., Citation2015), but effects in mice or with females remain to be determined. Future studies directed at studying microglial function, as part of the local immune response and/or with a role in synaptic pruning, will be important to contextualize the current findings.
Authors contributions
A.D., K.L., and J.B. completed the experiments. A.D., K.L., and T.R. wrote initial drafts of the manuscript and prepared the figures. J.B. and E.W. edited and contributed to the final drafts. All authors reviewed the manuscript.
Supplemental Material
Download Zip (589.2 KB)Disclosure statement
No potential conflict of interest was reported by the author(s).
Additional information
Funding
Notes on contributors
Alyshia B. Davis
Alyshia B. Davis (B.S. in neuroscience, University of Alabama at Birmingham, 2020) is a PhD candidate in Systems Biology and Physiology at the University of Cincinnati. Her work focuses on the role of astrocytes and microglia in adolescent chemotherapy induced cognitive impairments.
Kelsey R. Lloyd
Kelsey R. Lloyd (PhD in neuroscience, University of Cincinnati, 2021) is a Research Scientist at CTI Clinical Trial and Consulting Services, Covington, Kentucky. Her focus is on medical writing and clinical research.
Justin L. Bollinger
Justin L. Bollinger (Ph.D. in psychology and neuroscience, Indiana University, 2018) is a research scientist at the University of Cincinnati College of Medicine. His work is focused on glial mechanisms in stress-induced brain and behavioral dysfunction.
Eric S. Wohleb
Eric S. Wohleb, Ph.D. is an Associate Professor in the Department of Pharmacology and Systems Physiology at the University of Cincinnati College of Medicine. His work focuses on how neuroimmune systems influence synaptic function and complex behavior in preclinical models of neurological disease and psychiatric disorders.
Teresa M. Reyes
Teresa M. Reyes PhD is a Professor of Pharmacology and Systems Physiology and Senior Associate Dean for Basic and Translational Research at the University of Cincinnati, College of Medicine. Her research interest is effects of adverse early life environment on brain development and cognition.
References
- Alexaki, V. I. (2021). The impact of obesity on microglial function: Immune, metabolic and endocrine perspectives. Cells, 10(7), 1. https://doi.org/10.3390/cells10071584
- Andersen, C. L., Jensen, J. L., & Ørntoft, T. F. (2004). Normalization of real-time quantitative reverse transcription-PCR data: A model-based variance estimation approach to identify genes suited for normalization, applied to bladder and colon cancer data sets. Cancer Research, 64(15), 5245–14. https://doi.org/10.1158/0008-5472.CAN-04-0496
- Astrup, A., Dyerberg, J., Selleck, M., & Stender, S. (2008). Nutrition transition and its relationship to the development of obesity and related chronic diseases. Obesity Reviews, 9 Suppl 1, 48–52. https://doi.org/10.1111/j.1467-789X.2007.00438.x
- Beilharz, J. E., Maniam, J., & Morris, M. J. (2015). Diet-induced cognitive deficits: The role of fat and sugar, potential mechanisms and nutritional interventions. Nutrients, 7(8), 6719–6738. https://doi.org/10.3390/nu7085307
- Boitard, C., Cavaroc, A., Sauvant, J., Aubert, A., Castanon, N., Layé, S., & Ferreira, G. (2014). Impairment of hippocampal-dependent memory induced by juvenile high-fat diet intake is associated with enhanced hippocampal inflammation in rats. Brain, Behavior, and Immunity, 40, 9–17. https://doi.org/10.1016/j.bbi.2014.03.005
- Boitard, C., Etchamendy, N., Sauvant, J., Aubert, A., Tronel, S., Marighetto, A., Layé, S., & Ferreira, G. (2012). Juvenile, but not adult exposure to high-fat diet impairs relational memory and hippocampal neurogenesis in mice. Hippocampus, 22(11), 2095–2100. https://doi.org/10.1002/hipo.22032
- Bollinger, J. L., & Wohleb, E. S. (2019). The formative role of microglia in stress-induced synaptic deficits and associated behavioral consequences. Neuroscience Letters, 711, 134369. https://doi.org/10.1016/j.neulet.2019.134369
- Bollinger, J. L., Horchar, M. J., & Wohleb, E. S. (2020). Diazepam limits microglia-mediated neuronal remodeling in the prefrontal cortex and associated behavioral consequences following chronic unpredictable stress. Neuropsychopharmacology, 45(10), 1766–1776. https://doi.org/10.1038/s41386-020-0720-1
- Bollinger, J. L., Horchar, M. J., & Wohleb, E. S. (2024). Repeated activation of pyramidal neurons in the prefrontal cortex alters microglial phenotype in male mice. The Journal of Pharmacology and Experimental Therapeutics, 388(2), 715–723. https://doi.org/10.1124/jpet.123.001759
- Butler, M. J. (2021). The role of Western diets and obesity in peripheral immune cell recruitment and inflammation in the central nervous system. Brain, Behavior, and Immunity-Health, 16, 100298. https://doi.org/10.1016/j.bbih.2021.100298
- Butler, M. J., Cole, R. M., Deems, N. P., Belury, M. A., & Barrientos, R. M. (2020). Fatty food, fatty acids, and microglial priming in the adult and aged hippocampus and amygdala. Brain, Behavior, and Immunity, 89, 145–158. https://doi.org/10.1016/j.bbi.2020.06.010
- Butler, M. J., Perrini, A. A., & Eckel, L. A. (2020). Estradiol treatment attenuates high fat diet-induced microgliosis in ovariectomized rats. Hormones and Behavior, 120, 104675. https://doi.org/10.1016/j.yhbeh.2020.104675
- Caballero, A., Granberg, R., & Tseng, K. Y. (2016). Mechanisms contributing to prefrontal cortex maturation during adolescence. Neuroscience and Biobehavioral Reviews, 70, 4–12. https://doi.org/10.1016/j.neubiorev.2016.05.013
- Cai, G., Dinan, T., Barwood, J. M., De Luca, S. N., Soch, A., Ziko, I., Chan, S. M. H., Zeng, X.-Y., Li, S., Molero, J., & Spencer, S. J. (2014). Neonatal overfeeding attenuates acute central pro-inflammatory effects of short-term high fat diet. Frontiers in Neuroscience, 8, 446. https://doi.org/10.3389/fnins.2014.00446
- Carlin, J. L., McKee, S. E., Hill-Smith, T., Grissom, N. M., George, R., Lucki, I., & Reyes, T. M. (2016). Removal of high-fat diet after chronic exposure drives binge behavior and dopaminergic dysregulation in female mice. Neuroscience, 326, 170–179. https://doi.org/10.1016/j.neuroscience.2016.04.002
- Carrillo-de Sauvage, M. Á., Maatouk, L., Arnoux, I., Pasco, M., Sanz Diez, A., Delahaye, M., Herrero, M. T., Newman, T. A., Calvo, C. F., Audinat, E., Tronche, F., & Vyas, S. (2013). Potent and multiple regulatory actions of microglial glucocorticoid receptors during CNS inflammation. Cell Death and Differentiation, 20(11), 1546–1557. https://doi.org/10.1038/cdd.2013.108
- Dantzer, R., O’Connor, J. C., Freund, G. G., Johnson, R. W., & Kelley, K. W. (2008). From inflammation to sickness and depression: When the immune system subjugates the brain. Nature Reviews. Neuroscience, 9(1), 46–56. https://doi.org/10.1038/nrn2297
- Dayas, C. V., Buller, K. M., Crane, J. W., Xu, Y., & Day, T. A. (2001). Stressor categorization: Acute physical and psychological stressors elicit distinctive recruitment patterns in the amygdala and in medullary noradrenergic cell groups. The European Journal of Neuroscience, 14(7), 1143–1152. https://doi.org/10.1046/j.0953-816x.2001.01733.x
- Deczkowska, A., Keren-Shaul, H., Weiner, A., Colonna, M., Schwartz, M., & Amit, I. (2018). Disease-associated microglia: A universal immune sensor of neurodegeneration. Cell, 173(5), 1073–1081. https://doi.org/10.1016/j.cell.2018.05.003
- Doremus-Fitzwater, T. L., Gano, A., Paniccia, J. E., & Deak, T. (2015). Male adolescent rats display blunted cytokine responses in the CNS after acute ethanol or lipopolysaccharide exposure. Physiology & Behavior, 148, 131–144. https://doi.org/10.1016/j.physbeh.2015.02.032
- Fakih, W., Zeitoun, R., AlZaim, I., Eid, A. H., Kobeissy, F., Abd-Elrahman, K. S., & El-Yazbi, A. F. (2022). Early metabolic impairment as a contributor to neurodegenerative disease: Mechanisms and potential pharmacological intervention. Obesity, 30(5), 982–993. https://doi.org/10.1002/oby.23400
- Gądek-Michalska, A., Tadeusz, J., Rachwalska, P., Spyrka, J., & Bugajski, J. (2011). Effect of prior stress on interleukin-1β and HPA axis responses to acute stress. Pharmacological Reports, 63(6), 1393–1403. https://doi.org/10.1016/s1734-1140(11)70703-4
- Gao, Y., Vidal-Itriago, A., Kalsbeek, M. J., Layritz, C., García-Cáceres, C., Tom, R. Z., Eichmann, T. O., Vaz, F. M., Houtkooper, R. H., van der Wel, N., Verhoeven, A. J., Yan, J., Kalsbeek, A., Eckel, R. H., Hofmann, S. M., & Yi, C.-X. (2017). Lipoprotein lipase maintains microglial innate immunity in obesity. Cell Reports, 20(13), 3034–3042. https://doi.org/10.1016/j.celrep.2017.09.008
- Gibb, J., Hayley, S., Gandhi, R., Poulter, M. O., & Anisman, H. (2008). Synergistic and additive actions of a psychosocial stressor and endotoxin challenge: Circulating and brain cytokines, plasma corticosterone and behavioral changes in mice. Brain, Behavior, and Immunity, 22(4), 573–589. https://doi.org/10.1016/j.bbi.2007.12.001
- Guneykaya, D., Ivanov, A., Hernandez, D. P., Haage, V., Wojtas, B., Meyer, N., Maricos, M., Jordan, P., Buonfiglioli, A., Gielniewski, B., Ochocka, N., Cömert, C., Friedrich, C., Artiles, L. S., Kaminska, B., Mertins, P., Beule, D., Kettenmann, H., & Wolf, S. A. (2018). Transcriptional and translational differences of microglia from male and female brains. Cell Reports, 24(10), 2773–2783.e6. https://doi.org/10.1016/j.celrep.2018.08.001
- Herman, J. P., & Cullinan, W. E. (1997). Neurocircuitry of stress: Central control of the hypothalamo-pituitary-adrenocortical axis. Trends in Neurosciences, 20(2), 78–84. https://doi.org/10.1016/s0166-2236(96)10069-2
- Hickman, S. E., Kingery, N. D., Ohsumi, T. K., Borowsky, M. L., Wang, L-C., Means, T. K., & El Khoury, J. (2013). The microglial sensome revealed by direct RNA sequencing. Nature Neuroscience, 16(12), 1896–1905. https://doi.org/10.1038/nn.3554
- Hirbec, H., Marmai, C., Duroux-Richard, I., Roubert, C., Esclangon, A., Croze, S., Lachuer, J., Peyroutou, R., & Rassendren, F. (2018). The microglial reaction signature revealed by RNAseq from individual mice. Glia, 66(5), 971–986. https://doi.org/10.1002/glia.23295
- Horchar, M. J., & Wohleb, E. S. (2019). Glucocorticoid receptor antagonism prevents microglia-mediated neuronal remodeling and behavioral despair following chronic unpredictable stress. Brain, Behavior, and Immunity, 81, 329–340. https://doi.org/10.1016/j.bbi.2019.06.030
- Ishii, Y., Yamaizumi, A., Kawakami, A., Islam, A., Choudhury, M. E., Takahashi, H., Yano, H., & Tanaka, J. (2015). Anti-inflammatory effects of noradrenaline on LPS-treated microglial cells: Suppression of NFκB nuclear translocation and subsequent STAT1 phosphorylation. Neurochemistry International, 90, 56–66. https://doi.org/10.1016/j.neuint.2015.07.010
- Iwata, M., Ota, K. T., Li, X.-Y., Sakaue, F., Li, N., Dutheil, S., Banasr, M., Duric, V., Yamanashi, T., Kaneko, K., Rasmussen, K., Glasebrook, A., Koester, A., Song, D., Jones, K. A., Zorn, S., Smagin, G., & Duman, R. S. (2016). Psychological stress activates the inflammasome via release of adenosine triphosphate and stimulation of the purinergic type 2X7 receptor. Biological Psychiatry, 80(1), 12–22. https://doi.org/10.1016/j.biopsych.2015.11.026
- Johnson, J. D., Zimomra, Z. R., & Stewart, L. T. (2012). Beta-adrenergic receptor activation primes microglia cytokine production. Journal of Neuroimmunology, 254(1–2), 161–164. https://doi.org/10.1016/j.jneuroim.2012.08.007
- Keren-Shaul, H., Spinrad, A., Weiner, A., Matcovitch-Natan, O., Dvir-Szternfeld, R., Ulland, T. K., David, E., Baruch, K., Lara-Astaiso, D., Toth, B., Itzkovitz, S., Colonna, M., Schwartz, M., & Amit, I. (2017). A unique microglia type associated with restricting development of Alzheimer’s disease. Cell, 169(7), 1276–1290.e17. https://doi.org/10.1016/j.cell.2017.05.018
- Kim, J. D., Yoon, N. A., Jin, S., & Diano, S. (2019). Microglial UCP2 mediates inflammation and obesity induced by high-fat feeding. Cell Metabolism, 30(5), 952–962.e5. https://doi.org/10.1016/j.cmet.2019.08.010
- Krasemann, S., Madore, C., Cialic, R., Baufeld, C., Calcagno, N., El Fatimy, R., Beckers, L., O’Loughlin, E., Xu, Y., Fanek, Z., Greco, D. J., Smith, S. T., Tweet, G., Humulock, Z., Zrzavy, T., Conde-Sanroman, P., Gacias, M., Weng, Z., Chen, H., … Butovsky, O. (2017). The TREM2-APOE pathway drives the transcriptional phenotype of dysfunctional microglia in neurodegenerative diseases. Immunity, 47(3), 566–581.e9. https://doi.org/10.1016/j.immuni.2017.08.008
- Lasselin, J., Schedlowski, M., Karshikoff, B., Engler, H., Lekander, M., & Konsman, J. P. (2020). Comparison of bacterial lipopolysaccharide-induced sickness behavior in rodents and humans: Relevance for symptoms of anxiety and depression. Neuroscience and Biobehavioral Reviews, 115, 15–24. https://doi.org/10.1016/j.neubiorev.2020.05.001
- Lovelock, D. F., & Deak, T. (2018). Neuroendocrine and neuroimmune adaptation to chronic escalating distress (CED): A novel model of chronic stress. Neurobiology of Stress, 9, 74–83. https://doi.org/10.1016/j.ynstr.2018.08.007
- Makinson, R., Lloyd, K., Rayasam, A., McKee, S., Brown, A., Barila, G., Grissom, N., George, R., Marini, M., Fabry, Z., Elovitz, M., & Reyes, T. M. (2017). Intrauterine inflammation induces sex-specific effects on neuroinflammation, white matter, and behavior. Brain, Behavior, and Immunity, 66, 277–288. https://doi.org/10.1016/j.bbi.2017.07.016
- Marsh, S. E., Walker, A. J., Kamath, T., Dissing-Olesen, L., Hammond, T. R., de Soysa, T. Y., Young, A. M. H., Murphy, S., Abdulraouf, A., Nadaf, N., Dufort, C., Walker, A. C., Lucca, L. E., Kozareva, V., Vanderburg, C., Hong, S., Bulstrode, H., Hutchinson, P. J., Gaffney, D. J., … Stevens, B. (2022). Dissection of artifactual and confounding glial signatures by single-cell sequencing of mouse and human brain. Nature Neuroscience, 25(3), 306–316. https://doi.org/10.1038/s41593-022-01022-8
- McWhirt, J., Sathyanesan, M., Sampath, D., & Newton, S. S. (2019). Effects of restraint stress on the regulation of hippocampal glutamate receptor and inflammation genes in female C57BL/6 and BALB/c mice. Neurobiology of Stress, 10, 100169. https://doi.org/10.1016/j.ynstr.2019.100169
- Milanova, I. V., Kalsbeek, M. J. T., Wang, X.-L., Korpel, N. L., Stenvers, D. J., Wolff, S. E. C., de Goede, P., Heijboer, A. C., Fliers, E., la Fleur, S. E., Kalsbeek, A., & Yi, C.-X. (2019). Diet-induced obesity disturbs microglial immunometabolism in a time-of-day manner. Frontiers in Endocrinology, 10, 424. https://doi.org/10.3389/fendo.2019.00424
- Ocañas, S. R., Pham, K. D., Blankenship, H. E., Machalinski, A. H., Chucair-Elliott, A. J., & Freeman, W. M. (2022). Minimizing the ex vivo confounds of cell-isolation techniques on transcriptomic and translatomic profiles of purified microglia. eNeuro, 9(2), ENEURO.0348-21.2022. https://doi.org/10.1523/ENEURO.0348-21.2022
- Osborne, B. F., Caulfield, J. I., Solomotis, S. A., & Schwarz, J. M. (2017). Neonatal infection produces significant changes in immune function with no associated learning deficits in juvenile rats. Developmental Neurobiology, 77(10), 1221–1236. https://doi.org/10.1002/dneu.22512
- Paolicelli, R. C., & Angiari, S. (2019). Microglia immunometabolism: From metabolic disorders to single cell metabolism. Seminars in Cell & Developmental Biology, 94, 129–137. https://doi.org/10.1016/j.semcdb.2019.03.012
- Picard, K., Bisht, K., Poggini, S., Garofalo, S., Golia, M. T., Basilico, B., Abdallah, F., Ciano Albanese, N., Amrein, I., Vernoux, N., Sharma, K., Hui, C. W., C Savage, J., Limatola, C., Ragozzino, D., Maggi, L., Branchi, I., & Tremblay, M.-È. (2021). Microglial-glucocorticoid receptor depletion alters the response of hippocampal microglia and neurons in a chronic unpredictable mild stress paradigm in female mice. Brain, Behavior, and Immunity, 97, 423–439.
- Porterfield, V. M., Zimomra, Z. R., Caldwell, E. A., Camp, R. M., Gabella, K. M., & Johnson, J. D. (2011). Rat strain differences in restraint stress-induced brain cytokines. Neuroscience, 188, 48–54. https://doi.org/10.1016/j.neuroscience.2011.05.023
- Ramirez, K., Fornaguera-Trías, J., & Sheridan, J. F. (2017). Stress-induced microglia activation and monocyte trafficking to the brain underlie the development of anxiety and depression. Current Topics in Behavioral Neurosciences, 31, 155–172. https://doi.org/10.1007/7854_2016_25.
- Reyes, T. M., Walker, J. R., DeCino, C., Hogenesch, J. B., & Sawchenko, P. E. (2003). Categorically distinct acute stressors elicit dissimilar transcriptional profiles in the paraventricular nucleus of the hypothalamus. The Journal of Neuroscience, 23(13), 5607–5616. https://doi.org/10.1523/JNEUROSCI.23-13-05607.2003
- Sárvári, M., Hrabovszky, E., Kalló, I., Solymosi, N., Likó, I., Berchtold, N., Cotman, C., & Liposits, Z. (2012). Menopause leads to elevated expression of macrophage-associated genes in the aging frontal cortex: Rat and human studies identify strikingly similar changes. Journal of Neuroinflammation, 9(1), 264. https://doi.org/10.1186/1742-2094-9-264
- Sathyanesan, M., Haiar, J. M., Watt, M. J., & Newton, S. S. (2017). Restraint stress differentially regulates inflammation and glutamate receptor gene expression in the hippocampus of C57BL/6 and BALB/c mice. Stress, 20(2), 197–204. https://doi.org/10.1080/10253890.2017.1298587
- Sawchenko, P. E., Brown, E. R., Chan, R. K. W., Ericsson, A., Li, H.-Y., Roland, B. L., & Kovács, K. J. (1996). Chapter 12: The paraventricular nucleus of the hypothalamus and the functional neuroanatomy of visceromotor responses to stress. In G. Holstege, R. Bandler, & C. B. Saper (Eds.), Progress in brain research (Vol. 107, pp. 201–222). Elsevier.
- Sawchenko, P. E., Li, H.-Y., & Ericsson, A. (2000). Chapter 6 – Circuits and mechanisms governing hypothalamic responses to stress: A tale of two paradigms. In E. A. Mayer & C. B. Saper (Eds.), Progress in brain research (Vol. 122, pp. 61–78). Elsevier.
- Schafer, D. P., Lehrman, E. K., Kautzman, A. G., Koyama, R., Mardinly, A. R., Yamasaki, R., Ransohoff, R. M., Greenberg, M. E., Barres, B. A., & Stevens, B. (2012). Microglia sculpt postnatal neural circuits in an activity and complement-dependent manner. Neuron, 74(4), 691–705. https://doi.org/10.1016/j.neuron.2012.03.026
- Schwarz, J. M., Sholar, P. W., & Bilbo, S. D. (2012). Sex differences in microglial colonization of the developing rat brain. Journal of Neurochemistry, 120(6), 948–963. https://doi.org/10.1111/j.1471-4159.2011.07630.x
- Sobesky, J. L., D’Angelo, H. M., Weber, M. D., Anderson, N. D., Frank, M. G., Watkins, L. R., Maier, S. F., & Barrientos, R. M. (2016). Glucocorticoids mediate short-term high-fat diet induction of neuroinflammatory priming, the NLRP3 inflammasome, and the danger signal HMGB1. eNeuro, 3(4), ENEURO.0113-16.2016. https://doi.org/10.1523/ENEURO.0113-16.2016
- Spencer, S. J., D’Angelo, H., Soch, A., Watkins, L. R., Maier, S. F., & Barrientos, R. M. (2017). High-fat diet and aging interact to produce neuroinflammation and impair hippocampal- and amygdalar-dependent memory. Neurobiology of Aging, 58, 88–101. https://doi.org/10.1016/j.neurobiolaging.2017.06.014
- Sugama, S., Takenouchi, T., Hashimoto, M., Ohata, H., Takenaka, Y., & Kakinuma, Y. (2019). Stress-induced microglial activation occurs through β-adrenergic receptor: Noradrenaline as a key neurotransmitter in microglial activation. Journal of Neuroinflammation, 16(1), 266. https://doi.org/10.1186/s12974-019-1632-z
- Tanaka, K. F., Kashima, H., Suzuki, H., Ono, K., & Sawada, M. (2002). Existence of functional β1- and β2-adrenergic receptors on microglia. Journal of Neuroscience Research, 70(2), 232–237. https://doi.org/10.1002/jnr.10399
- VanRyzin, J. W., Marquardt, A. E., Pickett, L. A., & McCarthy, M. M. (2020). Microglia and sexual differentiation of the developing brain: A focus on extrinsic factors. Glia, 68(6), 1100–1113. https://doi.org/10.1002/glia.23740
- Vegeto, E., Belcredito, S., Ghisletti, S., Meda, C., Etteri, S., & Maggi, A. (2006). The endogenous estrogen status regulates microglia reactivity in animal models of neuroinflammation. Endocrinology, 147(5), 2263–2272. https://doi.org/10.1210/en.2005-1330
- Vinuesa, A., Pomilio, C., Menafra, M., Bonaventura, M. M., Garay, L., Mercogliano, M. F., Schillaci, R., Lux Lantos, V., Brites, F., Beauquis, J., & Saravia, F. (2016). Juvenile exposure to a high fat diet promotes behavioral and limbic alterations in the absence of obesity. Psychoneuroendocrinology, 72, 22–33. https://doi.org/10.1016/j.psyneuen.2016.06.004
- Wang, J., Li, J., Sheng, X., Zhao, H., Cao, X.-D., Wang, Y.-Q., & Wu, G.-C. (2010). β-adrenoceptor mediated surgery-induced production of pro-inflammatory cytokines in rat microglia cells. Journal of Neuroimmunology, 223, 77–83.
- Wohleb, E. S., Fenn, A. M., Pacenta, A. M., Powell, N. D., Sheridan, J. F., & Godbout, J. P. (2012). Peripheral innate immune challenge exaggerated microglia activation, increased the number of inflammatory CNS macrophages, and prolonged social withdrawal in socially defeated mice. Psychoneuroendocrinology, 37(9), 1491–1505. https://doi.org/10.1016/j.psyneuen.2012.02.003
- Wohleb, E. S., Terwilliger, R., Duman, C. H., & Duman, R. S. (2018). Stress-induced neuronal colony stimulating factor 1 provokes microglia-mediated neuronal remodeling and depressive-like behavior. Biological Psychiatry, 83(1), 38–49. https://doi.org/10.1016/j.biopsych.2017.05.026
- Xu, H., Rajsombath, M. M., Weikop, P., & Selkoe, D. J. (2018). Enriched environment enhances β-adrenergic signaling to prevent microglia inflammation by amyloid-β. EMBO Molecular Medicine, 10. https://doi.org/10.15252/emmm.201808931.
- Yang, Y., Duan, C., Huang, L., Xia, X., Zhong, Z., Wang, B., Wang, Y., & Ding, W. (2020). Juvenile high–fat diet–induced senescent glial cells in the medial prefrontal cortex drives neuropsychiatric behavioral abnormalities in mice. Behavioural Brain Research, 395, 112838. https://doi.org/10.1016/j.bbr.2020.112838