Abstract
Stress has been linked to the development of irritable bowel syndrome (IBS), and various methods have been explored to model IBS in combination with other stimuli. However, it remains unclear whether stress alone can induce IBS in animals. This study aimed to investigate the impact of chronic unpredictable mild stress (CUMS) on gastrointestinal sensation and function in mice and assess the potential of CUMS as a modeling approach for IBS. To evaluate the mice’s behavior, we conducted open field test, sucrose preference test and weighed the mice, revealing that CUMS indeed induced anxiety and depression in the mice and caused weight loss. Further analyses, including fecal analysis, a total gastrointestinal transport test, and a colon propulsion test, demonstrated that CUMS led to abnormal defecation and disruptions in gastrointestinal motility in the mice. Additionally, the abdominal withdrawal reflex test indicated an increase in visceral sensitivity in CUMS-exposed mice. Histological examination using hematoxylin and eosin staining revealed no significant histological alterations in the colons of CUMS-exposed mice, but it did show a minor degree of inflammatory cell infiltration. In summary, the findings suggest that CUMS can replicate IBS-like symptoms in mice, offering a novel top-down approach to modeling IBS.
1. Introduction
Irritable bowel syndrome (IBS) is a complex gut–brain interaction disorder characterized by recurrent abdominal pain, altered bowel habits, and frequent abdominal distension, ranking among the most prevalent functional gastrointestinal disorders (Chey et al., Citation2015; Staudacher et al., Citation2023). Its overall prevalence, reported to reach as high as 9.2%, has seen an increased risk post-COVID-19, significantly impacting patients’ quality of life (Marasco et al., Citation2023; Oka et al., Citation2020). Nevertheless, the precise etiology of IBS remains enigmatic, with various factors potentially contributing to its development, such as genetic predisposition, dietary intolerance, immune activation, and psychological factors (Gao et al., Citation2023). Studies have long found that people with IBS have significantly higher levels of anxiety and depression than healthy people, and IBS is associated with a high prevalence of psychological disorders (Fond et al., Citation2014). More and more researches have spotlighted the co-occurrence of IBS with psychiatric and gastrointestinal conditions (Hochstrasser & Angst, Citation1996; Tesfaye et al., Citation2023), underscoring the growing interest in the psychosomatic connection to IBS. A study indicates that depressive disorders may heighten the susceptibility to IBS (Chen et al., Citation2023).
The chronic unpredictable mild stress (CUMS) model, which subjects animals to prolonged exposure to diverse stressors, effectively simulating unpredictable and variable physiological and psychological stress, stands as the classic animal model of depression (Yang et al., Citation2023; Zhang et al., Citation2023). It has been demonstrated that CUMS not only induces depressive-like behaviors in animals but also triggers alterations in the intestinal barrier, heightened visceral sensitivity, changes in the fecal microbiome, and metabolic disruptions (Li et al., Citation2023; Xu et al., Citation2023). Although there is evidence that CUMS, either alone or in combination with other techniques, can induce IBS-like symptoms in animals (Ma et al., Citation2019), the choice of stressors employed in CUMS can vary significantly among studies, some of which can be excessively severe, such as hot water baths, leading to animal mortality (Li et al., Citation2021). Moreover, many investigations have predominantly focused on the elicited alterations in visceral sensitivity, often neglecting the broader impact of CUMS on factors like gastrointestinal motility (Xu et al., Citation2023).
In this study, we probed the consequences of CUMS on behavior, visceral sensitivity, gastrointestinal motility, and colonic histopathology in mice. Our findings affirm the suitability of CUMS as a dependable animal model for IBS. Importantly, the techniques we employed for modeling are straightforward and readily accessible, accompanied by a detailed account of the method and timeline, providing a strong foundation for the successful replication of this model.
2. Materials and methods
2.1. Animal housing and husbandry
SPF C57BL/6 male mice, aged 6–7 weeks (total of 32), were sourced from the Hubei Laboratory Animal Research Center. The mice were carefully accommodated in sterilized, individually ventilated racks, with four mice housed in each cage. The animal housing facility was meticulously controlled to maintain a stable environment, with a temperature set at 23 ± 1 °C, humidity at 50 ± 5%, a consistent 12-h light/12-h dark cycle, and the lights were turned on at 7 a.m. and turned off at 7 p.m. These mice had unrestricted access to both food (LAD 1000; Trophic Animal Feed High-Tech Co., Ltd., China) and water throughout the study period.
Prior to any experimental procedures, all protocols were ethically reviewed and approved by the Animal Ethics Committee of Hubei University of Traditional Chinese Medicine. Furthermore, the mice were allowed a period of one week to acclimate to their new environment before the commencement of the experiments.
2.2. Experimental design
The mice were allocated randomly into two equivalent groups: the control group and the CUMS group, with 16 mice in each group (n = 16 for each group). The CUMS group was subjected to daily exposure to CUMS for a duration of 7 weeks, while the control mice remained unexposed to any form of stimulation. Starting from D0, we weighed the mice once a week, with weighing taking place before modeling on that day. Following the behavioral assessments, tissue samples from the colon were collected. The comprehensive schedule for the entire experimental design is visually depicted in . We excluded unavailable data resulting from human errors. The overall mortality rate of animals throughout the experiment was approximately 31% (13 mice remaining in the control group and 9 mice in the CUMS group prior to sampling).
Figure 1. Illustration of the experimental design. After 7 days of acclimation, the CUMS group began to undergo CUMS procedures, which lasted for 7 weeks in total. Once a week, fecal collection and disposal were performed on all mice starting from Day 14. The mice underwent behavioral tests in the following order: OFT on Day 50, SPT from Day 50 to Day 53, distal colorectal propulsion test on Day 54, TGIT on Day 54, and AWR on Day 55. Colon tissue samples were collected after the final behavioral test on Day 55. Note: OFT: open field test, SPT: sucrose preference test, TGIT: total gastrointestinal transit time, AWR: abdominal withdrawal reflex test, CUMS: Chronic unpredictable mild stress, H&E: hematoxylin and eosin.
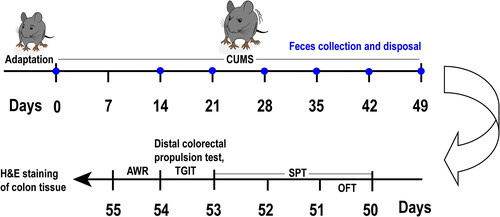
2.3. Model establishment
In accordance with a prior investigation (Li et al., Citation2022), the detailed procedures for CUMS induction consisted of the following stressors: In a specific behavioral room, the procedures included light-dark cycle reversal (for 24 h), physical restraint (for 2 h), forced swimming in cold water (at 4 °C, for 5 min), and tail pinch (for 1 min). In the animal facility, the procedures involved food deprivation (for 24 h), water deprivation (for 24 h), cage tilt (at a 45° angle for 7 h), and wet bedding exposure (for 24 h). A comprehensive timetable of these procedures is presented in . Below, we outline the specific operational steps and precautions for each type of stimulation:
Table 1. Chronic unpredictable mild stress schedule
Food deprivation: The mice were subjected to a period of food deprivation, starting at 9 a.m., and concluding at 9 a.m. the following day, lasting for a total duration of 24 h;
Water deprivation: The water bottles were removed from the cages at 9 a.m. and then reprovided to the mice at 9 a.m. the following day, resulting in a 24-hour period of water deprivation;
Cage tilt: The procedure involved tilting the cages by 45° at 9 a.m. of the day. Bedding within the cages was removed, and the cage lids were securely fastened to prevent the mice from escaping. Additionally, the mice’s food was positioned at an elevated point within the cage, making it more challenging for the mice to access their food. This arrangement was maintained for a continuous period of 7 hours;
Wet bedding: Commencing at 9 a.m., a specific quantity of water was introduced into the mouse cage bedding. The water was meticulously blended with the bedding at a ratio of 100 g bedding (clean grade wood shavings) to 200 mL water. This wet bedding configuration was sustained for a period of 24 h. At 9 o’clock the following day, the wet bedding was removed, and a dry bedding pad was substituted in its place;
Light-dark reversal: The procedure commenced around 7 a.m., with the mouse cages being enveloped by a double-thick black plastic bag. Careful attention was paid to ensuring that the bag did not come into close proximity to the cages, preventing the mice from damaging the bag while ensuring proper ventilation. Approximately at 7 p.m., the black bag was removed, and the cage area was illuminated by turning on the lamps equivalent to daylight housing conditions. This light-dark reversal cycle concluded around 7 a.m. the following day, making the entire process span a continuous 24-h period;
Physical constraint: To implement this procedure, holes were carefully created in advance in the tip (one hole), walls (six holes), and cap (one hole) of 50 mL EP tubes (Biosharp, Hefei, China). It was essential that these holes had smooth, rounded edges with an approximate diameter of 6 mm. Moreover, it was imperative to maintain a certain distance between each hole to prevent mice from biting or escaping. The mice were then gently placed into the suitably perforated 50 mL EP tubes at 2 p.m. Their orientation within the tubes ensured that the mice’s heads faced the bottom of the centrifuge tubes, while their tails protruded from the holes in the lid. The holes surrounding the tubes were positioned as upward as possible to ensure adequate ventilation. This constraint procedure was executed for a duration of two hours;
Forced swimming: During this procedure, the mice were compelled to swim in a spacious, open plastic box measuring 50 cm in length, width, and height at 2 p.m. The water in the box was maintained at a frigid temperature of 4 °C, with a water height of 3 cm. The mice were required to swim in this chilled water for a period of 5 minutes and were covered with transparent, perforated plastic plates to prevent them from leaping out of the container. Following the cold swim, each mouse was carefully removed from the water and gently dried with a towel. Measuring temperature in real-time by placing a thermometer in the water and add crushed ice when the temperature rises to cool it down. After forced swimming, excess water is dried off with towels, animals are placed on heated pads, and gentle heat from a hair dryer is used to help them regain body temperature. After ensuring the mice’s vital signs were stable and in good condition, it was then returned to its respective cages;
Tail pinch: A hemostatic clamp was applied to the mouse’s tail at 2 p.m., gripping it with the appropriate force, ensuring not to exert excessive pressure to prevent injury or tail breakage. Each mouse was clamped in this manner for one minute, during which time, do not touch the mouse to avoid being bitten. It’s crucial to emphasize that the tail pinch was consistently executed by a single person each time to maintain uniform stimulation and prevent variations in response due to human factors.
2.4 Behavior testing
2.4.1. Open field test (OFT)
The mice were subjected to observation to assess their movement and anxiety-related behavior. Before the actual testing, the mouse cages were introduced to the specialized behavior room and the mice were allowed to acclimate to the surroundings for a period of 2 h. Subsequently, each mouse was positioned at the center of an open-field experimental apparatus measuring 50 × 50 × 50 cm3, where they were granted five minutes of free exploration in a dimly lit environment (about 30 lux). Their behaviors were meticulously recorded through a video tracking system positioned directly above the enclosure, and the time spent in the central area as well as the surrounding regions was analyzed using SuperMaze software (version 2.0; Shanghai Xinruan Information Tech Co., Shanghai, China).
2.4.2. Sucrose preference test (SPT)
Referring to the previous method (Leng et al., Citation2018), the mice were individually placed in cages and initially trained to consume a 1% sucrose solution from two separate bottles, a process conducted 48 hours prior to the official experiment. After this 24-h period, the animals were granted unrestricted access to both a 1% sucrose solution and water from two distinct bottles, each of which had been weighed and recorded in advance. To prevent potential bottle placement preferences, the positions of the two bottles were swapped after 12 h. During the experiment, the mice were provided with regular food and were not subjected to CUMS. Upon completion, the mice were returned to their original cages. The quantities consumed from both bottles were measured 24 h later, and the sucrose preference was calculated using the following formula: sucrose preference (%) = [sucrose consumption/(sucrose consumption + water consumption)] × 100%.
2.5. Feces collection and disposal
We introduced a minor modification to the preceding experimental procedure (Rastelli et al., Citation2022). Starting at 9 am, the mice were individually relocated to clean, unlined cages and observed separately. We recorded the number of fecal particles expelled spontaneously by each mouse within a 60-min period and used cotton balls to absorb any urine promptly. After an hour we put the mice back in their original cage and employed tweezers to collect the excreted feces. The cumulative weight of the collected feces represented the "wet" weight for each animal. Subsequently, the fecal particles were subjected to a drying process at 45 °C, maintained for a minimum of 48 h, until a consistent mass was achieved, and the resulting “dry” weight was recorded. The fecal moisture content percentage was calculated using the formula: [(“wet” − “dry”)/“wet”] × 100%; and the average mass of fecal particles was determined as "wet" weight divided by the number of particles.
2.6. Gastrointestinal motility
2.6.1. Total gastrointestinal transit time (TGIT)
Carmine dye (Aladdin, C1110713) was dissolved in a 0.5% carboxymethyl cellulose solution (Aladdin, G2107073), and each mouse received 300 μL of a 6% carmine solution via gavage (Israelyan et al., Citation2019). We removed the mice’s food from their original cage and fasted them for 16 h before the procedure began. The mice were individually placed in separate cages lined with white paper for monitoring. The duration from gavage to the initial appearance of carmine in the feces was recorded as the total intestinal transit time for the animal.
2.6.2. Distal colorectal propulsion test
Animals were anesthetized with isoflurane, and a 3 mm diameter glass bead was gently inserted through the anus into the colon using a polished glass rod, positioned 2 cm from the anal margin. The time taken for the animal to expel the bead was measured and utilized to estimate colorectal propulsion in vivo (Israelyan et al., Citation2019).
2.7. Abdominal withdrawal reflex (AWR)
As previously detailed (Lee et al., Citation2020), visceral sensitivity was evaluated using the AWR test (Al-Chaer et al., Citation2000). The AWR procedure started at 9:00 a.m. and ended at 12:00 p.m. Mice were briefly anesthetized with isoflurane, and a 6-French balloon catheter (with a 2 mm outer diameter) was gently inserted through the anus into the descending colon. Subsequently, the mice were placed in individual cages for a 30-min adaptation period. After the adaptation period, a series of colonic distensions commenced: the distensions were divided into gradients based on the inflation volume of the balloon (0.25, 0.35, and 0.50 mL), each gradient lasting for 20 s (with a gradual pressure increase over 5 s, maintaining pressure for 10 s, and slowly releasing pressure over 5 s). The same gradient was repeated twice before moving on to the next gradient. Note that to ensure the comfort of the mice and reduce pain, there was a 30-s rest after every 20-s distension. During the distension period, observe the mice’s condition, record scores, and calculate the average score for each gradient. The specific scoring criteria were as follows: 0 – no behavioral response to colorectal distention; 1 – minimal head movement observed; 2 – abdominal muscle contraction; 3 – elevation of the abdomen; 4 – arching of the body and lifting of the pelvis.
2.8. Histopathologic evaluation
Following a 18-hour fasting period, the mice were humanely euthanized via cervical dislocation. The distal colon, approximately 2 cm in length, was incised longitudinally, and its contents were washed away with chilled normal saline at 4 °C. Subsequently, the colon tissues were fixed with 4% paraformaldehyde for 24 h, embedded in paraffin, and sectioned into 4 μm slices. Follow the methods outlined in the previous study for Hematoxylin and Eosin (H&E) staining (Gao et al., Citation2019). Two independent pathologists conducted a semi-quantitative blind evaluation based on previously established criteria (Asseman et al., Citation1999).
2.9. Statistical analysis
We conducted statistical analysis using GraphPad Prism 9.0 (San Diego, CA, USA). For normally distributed measurement data, we employed t-tests, while for data not conforming to normal distribution, we utilized the Wilcoxon rank-sum test (also known as the Mann-Whitney U test). All data are presented as mean ± SD deviation.
To assess the impact of treatment and time on fecal sample analysis results and the AWR score, we performed two-factor repeated measure analysis of variance (Two-way ANOVA) and conducted Bonferroni’s multiple comparisons test for multiple comparisons. All statistical tests were two-tailed, and differences were considered statistically significant at P < 0.05.
3. Results
3.1. Body weight
During the modeling period, we measured the weight of mice every other week (). The results indicated a significant decrease in the weight of mice in the CUMS group at both the second week (D14: P = 0.0199) and the seventh week (D49: P = 0.0280).
3.2. Behavior testing
We examined the impact of CUMS on the depression-like behavior of mice using the OFT and SPT (), starting from day 50. In comparison to the control group, CUMS-exposed mice spent more time and covered a greater distance in the peripheral area of the OFT chamber ( and (, t(18) =3.954, P = 0.0009), indicative of depression-like symptoms. Furthermore, in the SPT, CUMS mice exhibited a significant reduction in preference for the 1% sucrose solution (, t(12) =2.512, P = 0.0273).
3.3. Fecal analysis
To explore the impact of CUMS on the fecal characteristics of mice, feces were collected from the first day of the model, between 9 am and 10 am, once a week (except for the first week). These samples were counted and weighed. The results demonstrated that fecal water content, fecal pellet output, and fecal weight in the CUMS group began to fluctuate in a manner consistent with the control group. However, a gradual and significant decrease was observed on the 42nd day, reaching statistical significance on the 49th day ( = 0.0215; = 0.0461; = 0.0277). This suggests that CUMS can reduce fecal water content in mice, resulting in constipation.
3.4. Gastrointestinal motility
Building upon our observations of abnormal defecation in CUMS-exposed mice, we further examined the total gastrointestinal transport time and colonic propulsion time to provide stronger evidence regarding the impact of CUMS on gastrointestinal motility. The results indicated a significant increase in the total transit time within the gastrointestinal tract of CUMS mice (U = 11, P = 0.0270) (). Moreover, colonic propulsion time was also significantly increased (U = 8, P = 0.0028) (). These findings suggest that CUMS diminishes motility in both the gastrointestinal tract and the colon.
Figure 5. Dynamic detection of gastrointestinal tract. (A) Total transit time in the gastrointestinal tract; (B) Colonic propulsion time. Wilcoxon rank-sum test, n = 8–9 mice/group. Boxplots: lower, middle and upper hinges correspond to 25th, 50th and 75th percentiles. Upper and lower whiskers extend to largest and smallest value no further than 1.5 * IQR from respective hinge. *P < 0.05, **P < 0. 01. Note: GI: gastrointestinal.
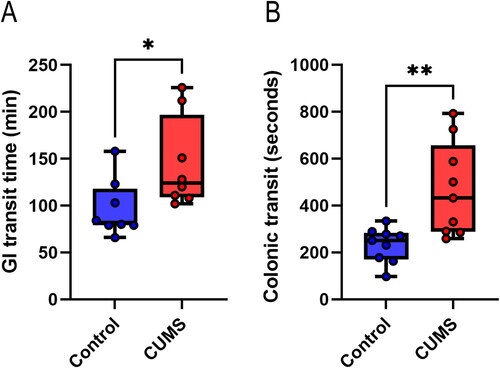
3.5. Visceral sensitivity
To investigate whether CUMS influences gut sensitivity, we conducted AWR tests. The findings revealed a significant enhancement in AWR scores for mice subjected to CUMS (0.35 mL: P = 0.0019) (), indicating an augmentation of visceral sensitivity due to CUMS.
3.6. Histological analysis of colon
To further investigate whether any histological changes occurred in the colon, we examined histological sections of the distal colon. As illustrated in , the distal colon of the Control group exhibited a structurally intact appearance with well-arranged intestinal glands. The lamina propria remained intact, the crypt structure was neatly organized, the cup cells were intact, and there was no edema or submucosal detachment. In contrast, the CUMS group displayed a reduction in the number of intestinal glands ( = 0, P = 0.1000), along with a small amount of observed inflammatory cell infiltration (= 0, P = 0.1000). However, none of these differences were statistically significant. These results suggest that while CUMS leads to decreased gastrointestinal motility and heightened visceral sensitivity, it does not exert significant effects on the histological structure of the intestine.
Figure 7. Histological examination of colon. (A) Representative hematoxylin and eosin-stained sections of distal colon (The red arrow represents abnormal inflammatory infiltration; scale bar = 50 μm); (B) Histological score; (C) Number of intestinal glands per unit of visual field (400×). Wilcoxon rank-sum test. Results are presented as mean ± SD, n = 3 mice/group.
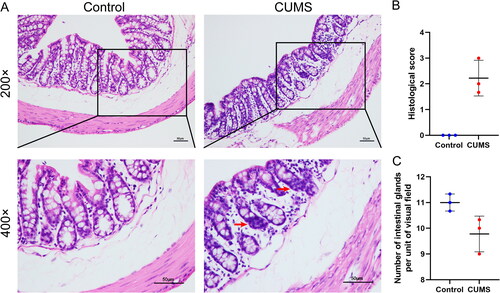
4. Discussion
IBS is not a singular disease but a collection of symptoms with various contributing factors, including changes in the intestinal microbiome, intestinal permeability, immune function, motility, visceral sensation, brain-gut interaction, and psychological state (Chey et al., Citation2015). Among these factors, psychological elements play a significant role in regulating the severity and persistence of IBS symptoms. Research has demonstrated that anxiety and depression levels are significantly higher in IBS patients compared to healthy individuals (Fond et al., Citation2014). Stress-related nervous and endocrine system disruptions, immune irregularities, and brain-gut axis dysfunction all seem to be crucial predisposing factors for IBS (Chong et al., Citation2019). However, studies examining the effects of stress, either alone or in combination, on gastrointestinal sensation and function have been limited and often poorly documented. Although a comprehensive stress model could better replicate the human disease process, it is more challenging to reproduce due to the complex interactions among stressors and the variable outcomes that depend on the chosen approach (Accarie & Vanuytsel, Citation2020). Consequently, we have provided a detailed description of the sequence and implementation of each stressor in . We found that chronic unpredictable mild stress (CUMS) can lead to a decrease in mouse body weight (). Interestingly, significant weight reduction in mice occurred only in the second week of modeling, with no statistical difference in weight thereafter, but significant weight reduction was observed again in the seventh week. This may be because the stress we applied was relatively mild, resulting in a slow decrease in mouse body weight, hence the difference appearing in the second week. Based on the weight trend after the second week, we speculate that mice have a certain tolerance to this mild stimulus (D21), but prolonged stress can still lead to mice becoming underweight (D49). As a classic chronic stress model commonly employed to induce depression, CUMS has been shown to produce symptoms of anxiety and depression in mice, which include increased peripheral activity in the open field and reduced sucrose preference (Sun et al., Citation2023), findings consistent with our results (). In this study, we have demonstrated that CUMS induces sensory and functional dysfunction in the gastrointestinal tract in mice, resembling IBS symptoms, suggesting that stress alone can lead to IBS.
Clinically, IBS can be categorized into four different subtypes based on stool shape and consistency: constipation-predominant IBS (IBS-C), diarrhea-predominant IBS (IBS-D), mixed bowel habits IBS, and unclassified IBS (Chey et al., Citation2015). However, most studies, both in animals and clinically, have primarily focused on IBS-D, while largely ignoring another common subtype, IBS-C (Du et al., Citation2023; Lu et al., Citation2023; Wang et al., Citation2023). Interestingly, in some regions, IBS-C has a higher incidence than IBS-D (Alghamdi et al., Citation2023). Nonetheless, the modeling method for this subtype in animal experiments remains inadequately described. On one hand, stress has been shown to stimulate the sympathetic nervous system and inhibit the vagus nerve (VN) (Ducarouge et al., Citation2017), while patients with major depression exhibit decreased VN activity (Schreiber et al., Citation2023). On the other hand, the main symptoms of IBS-C patients, including constipation and abdominal pain, can be ameliorated by stimulating the VN (Shi et al., Citation2021). The VN, which consists of approximately 4/5 afferent fibers and 1/5 efferent fibers, serves as a bidirectional communication channel between the brain and the gastrointestinal tract, playing a pivotal role in the brain-gut axis (Bonaz et al., Citation2017). These findings suggest that the impact of mental and emotional disturbances on intestinal function may be connected to the vagus nerve. Inhibition of vagus nerve tone has also been identified as a significant factor in reduced gastrointestinal motility (Bonaz et al., Citation2016). Our study reveals that CUMS reduces fecal water content (), fecal pellet output (), and average weight of fecal pellets () in mice. This indicates that CUMS-induced IBS may lean toward IBS-C, and stress may affect gastrointestinal motility by inhibiting the VN. Interestingly, we observed that CUMS mice initially display IBS-D-like behavior on the 14th day of stress exposure, but by the 21st day, fecal water content decreases once more, with a significant reduction in the number of fecal particles per hour. However, these parameters then recover until the 49th day when CUMS mice become constipated again. This fluctuation might be associated with the acute stress’s transient acceleration of colon movement (West et al., Citation2016). Additionally, during the experimental phase, both the Control group and the CUMS group experienced a decrease in fecal moisture content. We believe this may be related to the aging of the mice (Patel et al., Citation2014). Following the observation of fecal changes, we aimed to verify if there were actual differences in gastrointestinal transit in mice. We measured the total transit time of the entire gastrointestinal tract, and the results confirmed our hypothesis (). Studies have indicated that alterations in whole-colon and segmental colon transit times in IBS patients are vital contributors to changes in abnormal bowel habits (Törnblom et al., Citation2012). To further assess potential changes in colonic propulsion, we measured colon propulsion time in both groups seven weeks after modeling and found a significant increase in colon propulsion time in the CUMS group (), consistent with previous research (Bonaz et al., Citation2016). This phenomenon might be linked to reduced VN activity (Huang et al., Citation2022).
Furthermore, IBS is associated with the development of visceral sensitivity (Delvaux, Citation2002; Icenhour et al., Citation2019), and heightened perception of visceral stimulation is a key characteristic of IBS (Icenhour et al., Citation2017). Research has shown that abdominal pain is the primary symptom of IBS-C patients, while urgency in defecation is the main symptom in IBS-D patients (Caldarella et al., Citation2005). Chemical and mechanical stimulation of the intestinal wall can initiate neural reflexes within the enteric nervous system (ENS) (Spencer & Hu, Citation2020). The ENS is a complex network of neurons and glial cells embedded in the walls of the gastrointestinal tract, often referred to as the “brain in the gut” or the “second brain” (Wang, Citation2018). It governs all intestinal functions, including motor capacity (Rueckert & Ganz, Citation2022). The brain-gut axis serves as the communication pathway between the gut and the brain, facilitated by the VN, which plays a role in enhancing this communication (Irum et al., Citation2023). On the other hand, chronic exposure to stressors can lead to central dysregulation of the hypothalamic-pituitary-adrenal (HPA) axis, resulting in increased expression of corticotropin-releasing hormone (CRH). This further activates the HPA axis and sensitizes neurons in the central pain matrix. Local release of CRH in the enteric nervous system can also alter the sensitivity of primary afferents to distension (Greenwood-Van Meerveld & Johnson, Citation2017). Additionally, stress can affect the brain and gastrointestinal tract by acting on G-protein-coupled CRH receptors through the release of CRH and its related peptide urocorticosterone. This can increase intestinal permeability and alter the intestinal microbiota (Bonaz et al., Citation2018). Changes in intestinal permeability can lead to ecological dysregulation and sensitize individuals to visceral hypersensitivity (Moussaoui et al., Citation2017). Conversely, the VN can inhibit macrophages (Yuan & Taché, Citation2017) and exert anti-inflammatory effects, altering intestinal permeability. In this study, we have demonstrated that CUMS can increase visceral sensitivity in mice (). This is likely due to the influence of stressors on the release of factors within the brain-gut axis.
Functional gastrointestinal disorders (FGID) have traditionally been regarded as purely psychosomatic conditions. However, there is growing evidence of low-grade mucosal inflammation in FGIDs (Accarie & Vanuytsel, Citation2020). IBS, as a common FGID, exhibits persistent mucosal inflammation at both microscopic and molecular levels (Ng et al., Citation2018). Low-grade inflammation of the intestinal mucosa may play a significant role in its pathogenesis (Braak et al., Citation2012; Ohman & Simrén, Citation2010). In IBS subgroups, elevated innate immune activation is observed in both the intestinal mucosa and the bloodstream. A defining feature of the disease is a leaky gut, where the integrity of the intestinal blood barrier is compromised, allowing intestinal contents (such as immune cells and microbiota) to enter circulation, leading to low-grade systemic inflammation (Yuan et al., Citation2023). Conversely, inflammatory activity in the HPA axis can trigger the systemic release of glucocorticoids, thereby altering intestinal function (Agirman et al., Citation2021). Furthermore, the inflammatory state can activate intestinal glial cells, promoting the secretion of proteins like S100β, which then triggers inducible nitric oxide synthase (iNOS) production, increasing oxidative stress and inducing neuronal damage. The heightened activation of iNOS in intestinal glial cells also contributes to impaired epithelial permeability in patients with colitis (Fung & Vanden Berghe, Citation2020). In our study, CUMS did not induce significant organic lesions in the colon of mice, but rather led to a small amount of inflammatory cell infiltration (). This aligns with the histological status of functional diseases like IBS. These findings indicate that CUMS serves as a suitable model for top-down induction of IBS.
In the specific implementation of this experiment, we took precautions to ensure that the animals were not subjected to fasting or water deprivation on the day before feces collection. This was done to prevent any temporary food or water shortage from affecting the experimental results. Male animals were specifically chosen to avoid potential effects of hormonal fluctuations in female animals on the experimental data. Moreover, to minimize human error, we ensured consistency among operators during the fecal collection process. Nevertheless, this experiment still has some limitations: (1) The study focused solely on the impact of CUMS on male mice and did not explore its effects on female animals; (2) Feces from the mice were not collected immediately upon expulsion due to limited manpower. Instead, they were collected at a uniform time. However, efforts were made to maintain stable temperature and humidity in the collection room to mitigate the potential impact of excessive fluctuations on the experimental results. Additionally, the urine of the animals was cleaned promptly with cotton balls, minimizing the risk of errors arising from the mixing of urine and feces. (3) Fecal collection was not performed during the first week of modeling, which means that data related to the acute response of animals to stressors is missing.
This study is the first to provide a detailed examination of the effects of CUMS on gut sensation and function. It demonstrates that CUMS alone can be used to model IBS, allowing researchers to investigate the mechanisms of chronic stress on the gastrointestinal tract. Detailed modeling methods and timeframes have been presented, making it possible for others to replicate this model in future studies.
5. Conclusions
This study not only confirms that CUMS can induce IBS in animals but also observes the evolving patterns of fecal changes and body weight during the modeling process. Based on the results obtained, the following key findings emerge: (1) CUMS leads to anxiety and depression in mice, as evidenced by reduced peripheral access ratios and sucrose preferences in the open field test; (2) Long-term CUMS exposure prolongs gastrointestinal transit times, alters defecation patterns, reduces body weight and results in changes in stool quality; (3) Visceral sensitivity in CUMS-exposed mice is heightened; (4) While no significant histological changes were noted in the colons of CUMS-exposed mice, some degree of inflammatory cell infiltration was observed. In summary, this study presents an alternative model for the top-down modeling of IBS induced by chronic stress. Each existing model has its unique strengths and limitations. Researchers should carefully consider their specific experimental parameters and overall research objectives when selecting the most suitable model for their studies.
Author contributions
Fangyuan Liang: conceptualization, methodology, formal analysis, writing- original draft, writing-review and editing, project administration. Suzhen Liu: conceptualization, investigation, data curation, writing-original draft, writing-review and editing, supervision. Heng Zhang: conceptualization, formal analysis, investigation, visualization, writing-original draft, writing-review and editing. Ronglan Xiang: conceptualization, formal analysis, investigation, visualization, resources, writing-review and editing. Mengting Xie: resources, validation. Xiaoru He: data curation. Sunyi Wang: validation. Song Wu: conceptualization, supervision, project administration, funding acquisition. Jia Li: conceptualization, supervision, project administration, funding acquisition.
Ethics statement
The animal study was reviewed and approved by Animal Ethical and Welfare Committee of Hubei University of Chinese Medicine.
Acknowledgement
The authors acknowledge Professor Guang Yang, who helped review the study design.
Disclosure statement
No potential conflict of interest was reported by the authors.
Data availability statement
The dataset presented in this study can be obtained by contacting the authors.
Additional information
Funding
Notes on contributors
Fangyuan Liang
Fangyuan Liang is a master’s student at the College of Acupuncture and Orthopedics, Hubei University of Chinese Medicine, specializing in the regulatory effects of acupuncture on the nervous, immune, and endocrine systems.
Suzhen Liu
Suzhen Liu is a master’s student at the College of Acupuncture and Orthopedics, Hubei University of Chinese Medicine, specializing in the regulatory effects of acupuncture on the nervous, immune, and endocrine systems.
Heng Zhang
Heng Zhang is the director of the Traditional Chinese Medicine Department at Henan Provincial Workers’ Hospital, focusing on acupuncture treatment for brain diseases.
Ronglan Xiang
Ronglan Xiang is a master’s student at the College of Acupuncture and Orthopedics, Hubei University of Chinese Medicine, specializing in the regulatory effects of acupuncture on the nervous, immune, and endocrine systems.
Mengting Xie
Mengting Xie is a master’s student at the College of Acupuncture and Orthopedics, Hubei University of Chinese Medicine, specializing in the regulatory effects of acupuncture on the nervous, immune, and endocrine systems.
Xiaoru He
Xiaoru He is a doctoral student at the College of Acupuncture and Orthopedics, Hubei University of Chinese Medicine, specializing in the regulatory effects of acupuncture on the nervous, immune, and endocrine systems.
Sunyi Wang
Sunyi Wang is a master’s student at the College of Acupuncture and Orthopedics, Hubei University of Chinese Medicine, specializing in the regulatory effects of acupuncture on the nervous, immune, and endocrine systems.
Song Wu
Song Wu is a professor at the College of Acupuncture and Orthopedics, Hubei University of Chinese Medicine, specializing in the regulatory effects of acupuncture on the nervous, immune, and endocrine systems.
Jia Li
Jia Li is the director and a physician at Xianning Hospital of Traditional Chinese Medicine, specializing in the regulatory effects of acupuncture on the nervous, immune, and endocrine systems.
References
- Accarie, A., & Vanuytsel, T. (2020). Animal models for functional gastrointestinal disorders. Frontiers in Psychiatry, 11, 1. https://doi.org/10.3389/fpsyt.2020.509681
- Agirman, G., Yu, K. B., & Hsiao, E. Y. (2021). Signaling inflammation across the gut-brain axis. Science (New York, N.Y.), 374(6571), 1087–11. https://doi.org/10.1126/science.abi6087
- Al-Chaer, E. D., Kawasaki, M., & Pasricha, P. J. (2000). A new model of chronic visceral hypersensitivity in adult rats induced by colon irritation during postnatal development. Gastroenterology, 119(5), 1276–1285. https://doi.org/10.1053/gast.2000.19576
- Alghamdi, A. A., Alghamdi, A. M., Alshareef, M. A., AlGhamdi, A. A., Alghamdi, R. A., AlAmri, A. A., & Alzahrani, G. T. (2023). Assessment of sleep quality among adolescents and adults with self-diagnosed irritable bowel syndrome, in Jeddah, Saudi Arabia. Cureus, 15(7), e42778. https://doi.org/10.7759/cureus.42778
- Asseman, C., Mauze, S., Leach, M. W., Coffman, R. L., & Powrie, F. (1999). An essential role for interleukin 10 in the function of regulatory T cells that inhibit intestinal inflammation. The Journal of Experimental Medicine, 190(7), 995–1004. https://doi.org/10.1084/jem.190.7.995
- Bonaz, B., Bazin, T., & Pellissier, S. (2018). The vagus nerve at the interface of the microbiota-gut-brain axis. Frontiers in Neuroscience, 12, 49. https://doi.org/10.3389/fnins.2018.00049
- Bonaz, B., Sinniger, V., & Pellissier, S. (2016). Vagal tone: Effects on sensitivity, motility, and inflammation. Neurogastroenterology and Motility, 28(4), 455–462. https://doi.org/10.1111/nmo.12817
- Bonaz, B., Sinniger, V., & Pellissier, S. (2017). The vagus nerve in the neuro-immune axis: implications in the pathology of the gastrointestinal tract. Frontiers in Immunology, 8, 1452. https://doi.org/10.3389/fimmu.2017.01452
- Braak, B., Klooker, T. K., Wouters, M. M., Welting, O., van der Loos, C. M., Stanisor, O. I., van Diest, S., van den Wijngaard, R. M., & Boeckxstaens, G. E. (2012). Mucosal immune cell numbers and visceral sensitivity in patients with irritable bowel syndrome: is there any relationship? The American Journal of Gastroenterology, 107(5), 715–726. https://doi.org/10.1038/ajg.2012.54
- Caldarella, M. P., Milano, A., Laterza, F., Sacco, F., Balatsinou, C., Lapenna, D., Pierdomenico, S. D., Cuccurullo, F., & Neri, M. (2005). Visceral sensitivity and symptoms in patients with constipation- or diarrhea-predominant irritable bowel syndrome (IBS): Effect of a low-fat intraduodenal infusion. The American Journal of Gastroenterology, 100(2), 383–389. https://doi.org/10.1111/j.1572-0241.2005.40100.x
- Chen, D., Zhang, Y., Huang, T., & Jia, J. (2023). Depression and risk of gastrointestinal disorders: A comprehensive two-sample Mendelian randomization study of European ancestry. Psychological Medicine, 53(15), 7309–7321. https://doi.org/10.1017/S0033291723000867
- Chey, W. D., Kurlander, J., & Eswaran, S. (2015). Irritable bowel syndrome: a clinical review. JAMA, 313(9), 949–958. https://doi.org/10.1001/jama.2015.0954
- Chong, P. P., Chin, V. K., Looi, C. Y., Wong, W. F., Madhavan, P., & Yong, V. C. (2019). The microbiome and irritable bowel syndrome – A review on the pathophysiology, current research and future therapy. Frontiers in Microbiology, 10, 1136. https://doi.org/10.3389/fmicb.2019.01136
- Delvaux, M. (2002). Role of visceral sensitivity in the pathophysiology of irritable bowel syndrome. Gut, 51 Suppl 1(Suppl 1), i67–71. https://doi.org/10.1136/gut.51.suppl_1.i67
- Du, L., Zhang, Z., Zhai, L., Xu, S., Yang, W., Huang, C., Lin, C., Zhong, L. L. D., Bian, Z., & Zhao, L. (2023). Altered gut microbiota-host bile acid metabolism in IBS-D patients with liver depression and spleen deficiency pattern. Chinese Medicine, 18(1), 87. https://doi.org/10.1186/s13020-023-00795-9
- Ducarouge, B., Pelissier-Rota, M., Powell, R., Buisson, A., Bonaz, B., & Jacquier-Sarlin, M. (2017). Involvement of CRF2 signaling in enterocyte differentiation. World Journal of Gastroenterology, 23(28), 5127–5145. https://doi.org/10.3748/wjg.v23.i28.5127
- Fond, G., Loundou, A., Hamdani, N., Boukouaci, W., Dargel, A., Oliveira, J., Roger, M., Tamouza, R., Leboyer, M., & Boyer, L. (2014). Anxiety and depression comorbidities in irritable bowel syndrome (IBS): A systematic review and meta-analysis. European Archives of Psychiatry and Clinical Neuroscience, 264(8), 651–660. https://doi.org/10.1007/s00406-014-0502-z
- Fung, C., & Vanden Berghe, P. (2020). Functional circuits and signal processing in the enteric nervous system. Cellular and Molecular Life Sciences: CMLS, 77(22), 4505–4522. https://doi.org/10.1007/s00018-020-03543-6
- Gao, W., Wang, C., Yu, L., Sheng, T., Wu, Z., Wang, X., Zhang, D., Lin, Y., & Gong, Y. (2019). Chlorogenic acid attenuates dextran sodium sulfate-induced ulcerative colitis in mice through MAPK/ERK/JNK pathway. BioMed Research International, 2019, 6769713–6769789. https://doi.org/10.1155/2019/6769789
- Gao, Y., Ding, P., Wang, J., Zhang, C., Ji, G., & Wu, T. (2023). Application of metabolomics in irritable bowel syndrome in recent 5 years. International Immunopharmacology, 124(Pt A), 110776. https://doi.org/10.1016/j.intimp.2023.110776
- Greenwood-Van Meerveld, B., & Johnson, A. C. (2017). Stress-induced chronic visceral pain of gastrointestinal origin. Frontiers in Systems Neuroscience, 11, 86. https://doi.org/10.3389/fnsys.2017.00086
- Hochstrasser, B., & Angst, J. (1996). The Zurich Study: XXII. Epidemiology of gastrointestinal complaints and comorbidity with anxiety and depression. European Archives of Psychiatry and Clinical Neuroscience, 246(5), 261–272. https://doi.org/10.1007/BF02190278
- Huang, Z., Lin, Z., Lin, C., Chu, H., Zheng, X., Chen, B., Du, L., Chen, J. D. Z., & Dai, N. (2022). Transcutaneous electrical acustimulation improves irritable bowel syndrome with constipation by accelerating colon transit and reducing rectal sensation using autonomic mechanisms. The American Journal of Gastroenterology, 117(9), 1491–1501. https://doi.org/10.14309/ajg.0000000000001882
- Icenhour, A., Labrenz, F., Roderigo, T., Siebert, C., Elsenbruch, S., & Benson, S. (2019). Are there sex differences in visceral sensitivity in young healthy men and women? Neurogastroenterology and Motility, 31(9), e13664. https://doi.org/10.1111/nmo.13664
- Icenhour, A., Witt, S. T., Elsenbruch, S., Lowén, M., Engström, M., Tillisch, K., Mayer, E. A., & Walter, S. (2017). Brain functional connectivity is associated with visceral sensitivity in women with Irritable Bowel Syndrome. NeuroImage. Clinical, 15, 449–457. https://doi.org/10.1016/j.nicl.2017.06.001
- Irum, N., Afzal, T., Faraz, M. H., Aslam, Z., & Rasheed, F. (2023). The role of gut microbiota in depression: an analysis of the gut-brain axis. Frontiers in Behavioral Neuroscience, 17, 1185522. https://doi.org/10.3389/fnbeh.2023.1185522
- Israelyan, N., Del Colle, A., Li, Z., Park, Y., Xing, A., Jacobsen, J. P. R., Luna, R. A., Jensen, D. D., Madra, M., Saurman, V., Rahim, R., Latorre, R., Law, K., Carson, W., Bunnett, N. W., Caron, M. G., & Margolis, K. G. (2019). Effects of serotonin and slow-release 5-hydroxytryptophan on gastrointestinal motility in a mouse model of depression. Gastroenterology, 157(2), 507–521.e4. https://doi.org/10.1053/j.gastro.2019.04.022
- Lee, J.-Y., Cevallos, S. A., Byndloss, M. X., Tiffany, C. R., Olsan, E. E., Butler, B. P., Young, B. M., Rogers, A. W. L., Nguyen, H., Kim, K., Choi, S.-W., Bae, E., Lee, J. H., Min, U.-G., Lee, D.-C., & Bäumler, A. J. (2020). High-fat diet and antibiotics cooperatively impair mitochondrial bioenergetics to trigger dysbiosis that exacerbates pre-inflammatory bowel disease. Cell Host & Microbe, 28(2), 273–284.e6. https://doi.org/10.1016/j.chom.2020.06.001
- Leng, L., Zhuang, K., Liu, Z., Huang, C., Gao, Y., Chen, G., Lin, H., Hu, Y., Wu, D., Shi, M., Xie, W., Sun, H., Shao, Z., Li, H., Zhang, K., Mo, W., Huang, T. Y., Xue, M., Yuan, Z., … Zhang, J. (2018). Menin deficiency leads to depressive-like behaviors in mice by modulating astrocyte-mediated neuroinflammation. Neuron, 100(3), 551–563.e7. https://doi.org/10.1016/j.neuron.2018.08.031
- Li, H., Xiang, Y., Zhu, Z., Wang, W., Jiang, Z., Zhao, M., Cheng, S., Pan, F., Liu, D., Ho, R. C. M., & Ho, C. S. H. (2021). Rifaximin-mediated gut microbiota regulation modulates the function of microglia and protects against CUMS-induced depression-like behaviors in adolescent rat. Journal of Neuroinflammation, 18(1), 254. https://doi.org/10.1186/s12974-021-02303-y
- Li, Y., Li, J., Cheng, R., Liu, H., Zhao, Y., Liu, Y., Chen, Y., Sun, Z., Zhai, Z., Wu, M., Yan, Y., Sun, Y., & Zhang, Z. (2023). Alteration of the gut microbiome and correlated metabolism in a rat model of long-term depression. Frontiers in Cellular and Infection Microbiology, 13, 1116277. https://doi.org/10.3389/fcimb.2023.1116277
- Li, Y., Su, P., Chen, Y., Nie, J., Yuan, T. F., Wong, A. H., & Liu, F. (2022). The Eph receptor A4 plays a role in demyelination and depression-related behavior. Journal of Clinical Investigation, 132(8), e152187. https://doi.org/10.1172/JCI152187
- Lu, J., Chen, Y., Shi, L., Li, X., Fei, G., Li, J., Yang, A., & Fang, X. (2023). Cognition of abdominal pain and abdominal discomfort in Chinese patients with irritable bowel syndrome with diarrhea. BioPsychoSocial Medicine, 17(1), 31. https://doi.org/10.1186/s13030-023-00286-1
- Ma, J., Li, J., Qian, M., He, N., Cao, Y., Liu, Y., Wu, K., & He, S. (2019). The comprehensive pathophysiological changes in a novel rat model of postinflammatory visceral hypersensitivity. FASEB Journal: Official Publication of the Federation of American Societies for Experimental Biology, 33(12), 13560–13571. https://doi.org/10.1096/fj.201901489R
- Marasco, G., Maida, M., Cremon, C., Barbaro, M. R., Stanghellini, V., & Barbara, G. (2023). Meta-analysis: Post-COVID-19 functional dyspepsia and irritable bowel syndrome. Alimentary Pharmacology & Therapeutics, 58(1), 6–15. https://doi.org/10.1111/apt.17513
- Moussaoui, N., Jacobs, J. P., Larauche, M., Biraud, M., Million, M., Mayer, E., & Taché, Y. (2017). Chronic early-life stress in rat pups alters basal corticosterone, intestinal permeability, and fecal microbiota at weaning: influence of sex. Journal of Neurogastroenterology and Motility, 23(1), 135–143. https://doi.org/10.5056/jnm16105
- Ng, Q. X., Soh, A. Y. S., Loke, W., Lim, D. Y., & Yeo, W.-S. (2018). The role of inflammation in irritable bowel syndrome (IBS). Journal of Inflammation Research, 11, 345–349. https://doi.org/10.2147/JIR.S174982
- Ohman, L., & Simrén, M. (2010). Pathogenesis of IBS: role of inflammation, immunity and neuroimmune interactions. Nature Reviews. Gastroenterology & Hepatology, 7(3), 163–173. https://doi.org/10.1038/nrgastro.2010.4
- Oka, P., Parr, H., Barberio, B., Black, C. J., Savarino, E. V., & Ford, A. C. (2020). Global prevalence of irritable bowel syndrome according to Rome III or IV criteria: A systematic review and meta-analysis. The Lancet Gastroenterology & Hepatology, 5(10), 908–917. https://doi.org/10.1016/S2468-1253(20)30217-X
- Patel, B. A., Patel, N., Fidalgo, S., Wang, C., Ranson, R. N., Saffrey, M. J., & Yeoman, M. S. (2014). Impaired colonic motility and reduction in tachykinin signalling in the aged mouse. Experimental Gerontology, 53, 24–30. https://doi.org/10.1016/j.exger.2014.02.007
- Rastelli, D., Robinson, A., Lagomarsino, V. N., Matthews, L. T., Hassan, R., Perez, K., Dan, W., Yim, P. D., Mixer, M., Prochera, A., Shepherd, A., Sun, L., Hall, K., Ballou, S., Lembo, A., Nee, J., & Rao, M. (2022). Diminished androgen levels are linked to irritable bowel syndrome and cause bowel dysfunction in mice. The Journal of Clinical Investigation, 132(2), e150789. https://doi.org/10.1172/JCI150789
- Rueckert, H., & Ganz, J. (2022). How to Heal the Gut’s Brain: Regeneration of the Enteric Nervous System. International Journal of Molecular Sciences, 23(9), 23.
- Schreiber, L. S., Wozniak, D., Scheller, E., Böttcher, E., Pelz, J. O., & Schmidt, F. M. (2023). Enlarged cross-sectional area of the left vagus nerve in patients with major depressive disorder. Frontiers in Psychiatry, 14, 1237983. https://doi.org/10.3389/fpsyt.2023.1237983
- Shi, X., Hu, Y., Zhang, B., Li, W., Chen, J. D., & Liu, F. (2021). Ameliorating effects and mechanisms of transcutaneous auricular vagal nerve stimulation on abdominal pain and constipation. JCI Insight, 6(14), 13265. https://doi.org/10.1172/jci.insight.150052
- Spencer, N. J., & Hu, H. (2020). Enteric nervous system: Sensory transduction, neural circuits and gastrointestinal motility. Nature Reviews. Gastroenterology & Hepatology, 17(6), 338–351. https://doi.org/10.1038/s41575-020-0271-2
- Staudacher, H. M., Black, C. J., Teasdale, S. B., Mikocka-Walus, A., & Keefer, L. (2023). Irritable bowel syndrome and mental health comorbidity: Approach to multidisciplinary management. Nature Reviews. Gastroenterology & Hepatology, 20(9), 582–596. https://doi.org/10.1038/s41575-023-00794-z
- Sun, C., Shen, Y., Liu, P., et al. (2023). NLRC5 deficiency reduces LPS-induced microglial activation via inhibition of NF-κB signaling and ameliorates mice’s depressive-like behavior. International Journal of Molecular Sciences, 24(17), 13265. https://doi.org/10.3390/ijms241713265
- Tesfaye, M., Jaholkowski, P., Hindley, G. F. L., Shadrin, A. A., Rahman, Z., Bahrami, S., Lin, A., Holen, B., Parker, N., Cheng, W., Rødevand, L., Frei, O., Djurovic, S., Dale, A. M., Smeland, O. B., O’Connell, K. S., & Andreassen, O. A. (2023). Shared genetic architecture between irritable bowel syndrome and psychiatric disorders reveals molecular pathways of the gut-brain axis. Genome Medicine, 15(1), 60. https://doi.org/10.1186/s13073-023-01212-4
- Törnblom, H., Van Oudenhove, L., Sadik, R., Abrahamsson, H., Tack, J., & Simrén, M. (2012). Colonic transit time and IBS symptoms: What’s the link? The American Journal of Gastroenterology, 107(5), 754–760. https://doi.org/10.1038/ajg.2012.5
- Wang, W. (2018). Optogenetic manipulation of ENS – The brain in the gut. Life Sciences, 192, 18–25. https://doi.org/10.1016/j.lfs.2017.11.010
- Wang, Y., Ke, W., Gan, J., Zhu, H., Xie, X., He, G., Liu, S., Huang, Y., & Tang, H. (2023). MicroRNA-29b-3p promotes intestinal permeability in IBS-D via targeting TRAF3 to regulate the NF-κB-MLCK signaling pathway. PloS One, 18(7), e0287597. https://doi.org/10.1371/journal.pone.0287597
- West, C., Stanisz, A. M., Wong, A., & Kunze, W. A. (2016). Effects of Saccharomyces cerevisiae or boulardii yeasts on acute stress induced intestinal dysmotility. World Journal of Gastroenterology, 22(48), 10532–10544. https://doi.org/10.3748/wjg.v22.i48.10532
- Xu, Y., Yao, R., Zhao, W., Zhu, J., Yao, J., Zhang, G., & Liu, D. (2023). Spirocyclopiperazinium salt compound DXL-A-24 improves visceral sensation and gut microbiota in a rat model of irritable bowel syndrome. Heliyon, 9(6), e16544. https://doi.org/10.1016/j.heliyon.2023.e16544
- Yang, L., Huang, Y., Chen, F., Wang, Y., Su, K., Zhao, M., Tao, W., & Liu, W. (2023). Berberine attenuates depression-like behavior by modulating the hippocampal NLRP3 ubiquitination signaling pathway through Trim65. International Immunopharmacology, 123, 110808. https://doi.org/10.1016/j.intimp.2023.110808
- Yuan, P. Q., & Taché, Y. (2017). Abdominal surgery induced gastric ileus and activation of M1-like macrophages in the gastric myenteric plexus: prevention by central vagal activation in rats. American Journal of Physiology. Gastrointestinal and Liver Physiology, 313(4), G320–g329. https://doi.org/10.1152/ajpgi.00121.2017
- Yuan, Y., Wang, X., Huang, S., Wang, H., & Shen, G. (2023). Low-level inflammation, immunity, and brain-gut axis in IBS: unraveling the complex relationships. Gut Microbes, 15(2), 2263209. https://doi.org/10.1080/19490976.2023.2263209
- Zhang, Y., Sun, Y., Liu, Y., Liu, J., Sun, J., Liu, X., Fan, B., Lu, C., & Wang, F. (2023). Polygonum sibiricum polysaccharides exert the antidepressant-like effects in chronic unpredictable mild stress-induced depressive mice by modulating microbiota-gut-brain axis. Phytotherapy Research: PTR, 37(8), 3408–3423. https://doi.org/10.1002/ptr.7813