Abstract
There is strong evidence about the importance of catecholamines and calcium signaling in heart function. Also, interaction of these two systems is well documented. Catecholamines signal through adrenergic receptors, and further activate calcium transport either from the extracellular space, or from the intracellular calcium stores. This review summarizes current knowledge on catecholamine production in the heart, with special focus on the final enzyme in the catecholamine synthesizing pathway, phenylethanolamine N-methyltransferase (PNMT), in different cell types in the heart. Further, signaling through different types of adrenergic receptors in physiological conditions and after exposure to different stressors is discussed. Also, part of this review considers activation of an intracellular calcium transport system via inositol 1,4,5-trisphosphate receptor and to possible functional consequences in control and stress conditions.
Catecholamines and the heart
The catecholamines epinephrine and norepinephrine have profound influence on cardiac function, beginning almost as soon as the heart starts beating and continuing through the remaining stages of life. The cardiovascular augmentation during the classical stress “fight or flight” response is thought to be primarily due to catecholamine release from sympathetic nerve endings and from the adrenal medulla. Furthermore, the obligatory role of catecholamines in fetal cardiac development has been underscored by the finding that mice with knock-out of the genes of enzymes for catecholamine synthesis die in utero due to cardiac failure at an early developmental stage, before cardiac sympathetic innervation occurs (Thomas et al. Citation1995; Zhou et al. Citation1995). Under physiological conditions the principal catecholamine that regulates cardiac function is norepinephrine, since it is the main mediator of the sympathetic neural influence on the heart. Nevertheless, epinephrine is also important in heart function, since it increases heart rate and force of contraction, alters blood flow and uptake of glucose, oxygen, potassium and phosphate (Elayan et al. Citation1990a).
It has been generally assumed that epinephrine affects the heart by passage through the bloodstream from the adrenal medulla (Elayan et al. Citation1990a; Esler et al. Citation1990). Nevertheless, there is some evidence that epinephrine can be synthesized directly in the cardiac tissue. Kvetnansky et al. (Citation1979) found a slight but significant elevation of plasma epinephrine levels in adrenal medullectomized rats exposed to stress, suggesting an extra-adrenal source of epinephrine. Esler et al. (Citation1991) showed that epinephrine is released by the human heart in vivo, and it is also present in human plasma and urine after bilateral adrenalectomy (Shah et al. Citation1984). Moreover, patients with heart transplants maintain adequate cardiac function even in the absence of sympathetic reinnervation, although epinephrine from the adrenal medulla is still available (Rowan and Billingham Citation1988; Beckers et al. Citation2004).
Phenylethanolamine N-methyltransferase in the heart
The final enzyme in the catecholamine synthesizing cascade that converts norepinephrine to epinephrine is phenylethanolamine N-methyltransferase (PNMT, EC 2.1.1.28). Although PNMT activity is largely restricted to the adrenal medulla, where the majority of epinephrine is synthesized, findings of low PNMT activity in the rat heart have been reported by several authors (Axelrod Citation1962; Culman et al. Citation1987; Torda et al. Citation1987; Elayan et al. Citation1990b; Kennedy and Ziegler Citation1991, Citation2000; Kennedy et al. Citation1995; Ebert et al. Citation1996). PNMT mRNA was detected for the first time in embryonic rat hearts (Ebert et al. Citation1996) and subsequently in adult rat hearts (Krizanova et al. Citation2001; Slavikova et al. Citation2003). Moreover, recently we have shown that PNMT mRNA is expressed also in transplanted human hearts (Goncalvesova et al. Citation2004). Interestingly, distribution of PNMT mRNA levels within the heart seems not to be uniform. The left cardiac atrium has a much greater abundance of the PNMT mRNA compared to the right atrium, whereas levels in the ventricles are about 10-times lower (Krizanova et al. Citation2001). These data are in good agreement with previously published reports concerning localization of the PNMT activity in the cardiac atria and ventricles (Culman et al. Citation1987; Torda et al. Citation1987; Elayan et al. Citation1990b; Kennedy et al. Citation1995). PNMT activity in the adult rat heart was found to be greatest in the left atrium and many times lower in the ventricles (Culman et al. Citation1987; Torda et al. Citation1987). Despite the presence of the PNMT mRNA in the heart being confirmed by many authors (Ebert et al. Citation1996; Krizanova et al. Citation2001; Slavikova et al. Citation2003; Micutkova et al. Citation2004), the cell types that are able to express the PNMT in the heart are still a matter of investigation and discussion. Huang et al. (Citation1996, Citation2005) identified special cardiac cells capable of adrenergic paracrine signaling in mammalian hearts. These cells, which do not have neuronal or chromaffin cell ultrastructural morphology, were described as intrinsic cardiac adrenergic (ICA) cells. ICA cells containing mRNA and protein of enzymes involved in catecholamine biosynthesis could be identified in fetal human hearts at a developmental stage before sympathetic innervation occurs (Huang et al. Citation1996, Citation2005), but also in the adult heart. These findings provide evidence for the existence of an endogenous system responsible for catecholamine production in the fetal heart. Ebert and Thompson (Citation2001) have shown that cardiac cells expressing catecholamine biosynthetic enzymes are present in the rat embryonic heart, where they seem to be progressively associated with regions of the cardiac conduction system. Slavikova et al. (Citation2003) identified two types of catecholamine-handling intrinsic ganglion neurons: small intensely fluorescent (SIF) cells and large-diameter neurons. SIF cells exhibited tyrosine hydroxylase (TH)-immunoreactivity, but they were not positive for dopamine-β-hydroxylase (DBH). In contrast, large-diameter intrinsic TH-positive neurons displayed also DBH-immunoreactivity, thus indicating the capacity for the synthesis of norepinephrine. Presence of the DBH mRNA in rat atrial samples was also verified using reverse transcription and subsequent polymerase chain reaction (Slavikova et al. Citation2003). This finding of DBH expression in rat heart was confirmed by several authors (Kvetnansky et al. Citation2004; Micutkova et al. Citation2004). On the other hand, the presence of TH mRNA in the heart is still under investigation.
Based on data confirming much higher abundance of the PNMT mRNA in the cardiac atria compared to the ventricles, it seems most likely that the majority of the PNMT is expressed predominantly in neuronal cardiac ganglia, which are localized in the atria (Krizanova et al. Citation2001; Slavikova et al. Citation2003). On the other hand, a small amount of the PNMT mRNA was observed also in the cardiac ventricles, so the possibility that also cardiomyocytes express PNMT could not be excluded. Moreover, dual origin of the cardiac PNMT mRNA was supported by the finding that immobilization provoked different effects on the PNMT mRNA in the ganglionic (the part of the atria containing cardiac neuronal ganglia) and nonganglionic (the part of the atria without cardiac neuronal ganglia) parts of the atrium (Kvetnansky et al. Citation2004). Recently, we observed different effects of hypoxia and also cold stress on the PNMT mRNA in the cardiac atria and ventricles (Tillinger et al. Citation2006a). Finally, we have clearly confirmed PNMT gene expression in isolated cardiomyocytes and also in primary culture of isolated cardiomyocytes (Tillinger et al. Citation2006b). These results were confirmed by demonstrating PNMT-immunoreactivity in isolated cardiomyocytes (Tillinger et al. Citation2006a). Taking together, it becomes clear that PNMT in the heart is expressed in several different types of cells in the heart.
Phenylethanolamine N-methyltransferase and stress
The significance of PNMT gene expression in the heart was studied also under stress conditions. Single and also repeated immobilization stress significantly increases the PNMT mRNA levels in both, cardiac atria and ventricles (Krizanova et al. Citation2001; Kvetnansky et al. Citation2004; Micutkova et al. Citation2004). Since immobilization stress markedly activates many cardiovascular, metabolic, neuroendocrine and other processes, the highly stress-induced increase in cardiac PNMT mRNA levels suggests participation of the cardiac adrenergic system in physiological and/or pathophysiological processes (Krizanova et al. Citation2001). Subsequently we have found different modulation of PNMT gene expression in the heart by various stressors (cold, immobilization and hypoxia). Cold exposure for seven days significantly decreased PNMT mRNA levels in the left and right atrium of the rat compared to controls. Another stress stimulus, hypoxia for 6 h, significantly increased PNMT mRNA levels in cardiac atria compared to normoxic controls (Tillinger et al. Citation2006a). In contrast, PNMT gene expression in the ventricles was not affected by cold or the hypoxic stimulus. Thus, PNMT expression in the cardiac atria seems to be more vulnerable to different stressors compared to the ventricles. Moreover, these data indicated different regulatory mechanisms by which PNMT gene expression is modulated in different parts of the heart.
It is well known that glucocorticoids are critical regulators of PNMT gene expression (Bohn et al. Citation1981; Teitelman et al. Citation1982; Schmid et al. Citation1995; Tai et al. Citation2002) and also induction of PNMT in response to acute and chronic stress (Sabban et al. Citation1995; Citation1998). The final enzyme in epinephrine synthesis is regulated by glucocorticoids in two ways: postranscriptionally via regulation of PNMT co-substrate S-adenosylmethionine levels and transcriptionally through stimulation of glucocorticoid responsive elements (GREs), which occur in the proximal 5′- flanking sequences of the PNMT gene promoter (Jeong et al. Citation2000; Tai et al. Citation2002; Wong et al. Citation2002). Besides GREs, a synergistic activation of the PNMT promoter by EGR-1, AP-2 and the glucocorticoid receptor has been observed (Wong et al. Citation1998). Considering the important regulatory role of glucocorticoids, Kvetnansky et al. (Citation2004, Citation2006), used corticotropin-releasing hormone (CRH) knockout mice (CRH KO) as an experimental model. These mice, unable to synthesize a functional CRH, can help to define mechanisms that are involved in regulation of the PNMT during stress. CRH is the major driver of the hypothalamic pituitary adrenocortical (HPA) system and it triggers both basal and stress-induced release of ACTH from the anterior pituitary, subsequently leading to release of glucocorticoid hormones from the adrenal cortex (Bruhn et al. Citation1984; Venihaki and Majzoub Citation2002). CRH KO mice exhibit impaired endogenous glucocorticoid synthesis and secretion (Muglia et al. Citation2001). Kvetnansky et al. (Citation2004, Citation2006), have shown that single immobilization and also repeated stress exposure induce a significant increase in PNMT mRNA levels in the heart atria and ventricles of wild-type (WT) mice. In contrast to these findings, neither single immobilization, nor seven daily repeated immobilizations were able to induce a significant elevation of PNMT gene expression in the cardiac atria of CRH KO mice. A different picture was observed in the cardiac ventricles. Surprisingly, PNMT mRNA levels were significantly increased in both ventricles of CRH KO mice exposed to a single immobilization and a decrease was found only after repeated stress. These findings are in good agreement with a previously published report about different responses of PNMT gene expression in the atria and ventricles of adrenalectomised or hypophysectomised rats after stress exposure (Krizanova et al. Citation2001). In conclusion, all these data strongly indicate that the HPA axis plays a crucial role in regulation of the PNMT gene expression in the cardiac atria under stress conditions; however, PNMT gene expression in the cardiac ventricles is probably regulated by a different mechanism(s).
Adrenergic receptors and their occurrence in the heart
Both epinephrine and norepinephrine bind to adrenergic receptors (adrenoceptors). Adrenergic receptors are members of the G-protein-coupled receptor (GPCR) family. In the heart, several subtypes are expressed both at the gene and protein levels (Brodde and Michel Citation1999; Gauthier et al. Citation2000). The β1 subtype is considered to be the main subtype, since about 80% of cardiac β-adrenoceptors are β1 subtype. β2- and β3-adrenoceptor subtypes are also present in the cardiac tissue (Gauthier et al. Citation1998; Brodde and Michel Citation1999). Although human β3-adrenoceptors share 51% identity with β1-adrenoceptors and 46% identity with β2-adrenoceptor amino acid sequences, and there is 54% homology between β1- and β2-adrenoceptor amino acid sequences, the receptor subtypes show closer relationship between β1 and β3 subtypes than between β1 and β2 subtypes, what can be important in receptor regulation (see below).
In addition to β-adrenoceptors, α1-adrenoceptors and α2-adrenoceptors are also present in cardiac tissue. The α1-adrenoceptor and β-adrenoceptor subtypes are effector receptors, i.e. when activated lead to functional changes in the functioning of the myocardium/cardiomyocytes (Brodde and Michel Citation1999). In contrast, α2-adrenoceptors modulate catecholamine release from the nerve terminals, i.e. they are located pre-junctionally (Brodde and Michel Citation1999). Also, these receptors are as much as 30-times less abundant (at the mRNA level) than α1-adrenoceptors (α2C vs. α1A; Berkowitz et al. Citation1994). Moreover, both α1-adrenoceptors and β1-adrenoceptors have specific low-affinity binding sites (α1L, so called “β4”, respectively) that can affect cardiac physiology in other ways than via conventional binding sites (Ford et al. Citation1997; Takahashi et al. Citation2000; Kaumann et al. Citation2001; Santos et al. Citation2005). The questions arise, why are targets for catecholamine action in the heart so abundant, and why are there several adrenoceptor subtypes and what is their role in cardiac function?
Signaling pathways of adrenergic receptors
Firstly, it is necessary to mention the function/coupling/signalling pathways of the individual adrenoceptor subtypes. Although α1-adrenoreceptors in humans are less highly expressed in the myocardium than β-adrenoceptors, in rodents (especially in rat) the amount of α1-adrenoceptor binding sites is greater than the amount of β-adrenoceptor binding sites. Despite the lower density of α1-adrenoreceptors in the human heart, their physiological relevance is undoubted. These receptors are responsible for enhanced inotropy under most conditions, but opposite effects are also possible. In addition, stimulation of α1-adrenoceptors increases the Ca2 + sensitivity of the myofilaments. Apart from these short-term effects, extended stimulation of cardiac α1-adrenoceptors may also cause the development of a hypertrophic cardiac phenotype in rats (Zimmer Citation1997). Nevertheless, a hypertrophic effect due to extended stimulation of cardiac α1-adrenoceptors was shown in rodents and it is difficult to extrapolate these results into human physiology. α1-adrenoceptors couple to Gq/11 proteins (). Subsequent steps in the intracellular signalling cascade include activation of phospholipase C (PLC) and increase in intracellular diacylgylcerol (DAG) and inositol 1,4,5-trisphosphate (IP3) concentration (). Cardiac α1-adrenoceptors couple not only to PLC; phospholipase D can be also activated and coupling to various ion currents, i.e. L-type Ca2 + channels, the transient outward current Ito, the delayed rectifier K+ current, and the acetylcholine-activated K+ channel has been demonstrated (Endoh et al. Citation1991). Moreover, the Na+/H+ exchanger and Na+/K+-ATPase can be activated (Endoh et al. Citation1991).
Table I. Pharmacological characteristics of individual adrenoreceptor subtypes and coupling to G proteins with subsequent activation of second messengers.
Figure 1 Signaling cascades of individual types of adrenergic receptors. AC, adenylate cyclase; DAG, diacylglycerol; DHPR, dihydropyridine receptor, Gs; Gi, Gq/11, G proteins type s, i and q; IP3, inositol 1,4,5-trisphosphate; IP3R, IP3 receptor; NCX, sodium calcium exchanger; PKA, protein kinase A; PKB, protein kinase B; PKC, protein kinase C; PLC, phospholipase C; PIP2, phosphatidylinositol 4,5-diphosphate; RyR, ryanodine receptor; PLB, phospholamban; SERCA, sarcoplasmic reticulum calcium ATPase; CaMKII, calmodulin-dependent kinase II; PI3K, phosphatidyl inositol 3-kinase.
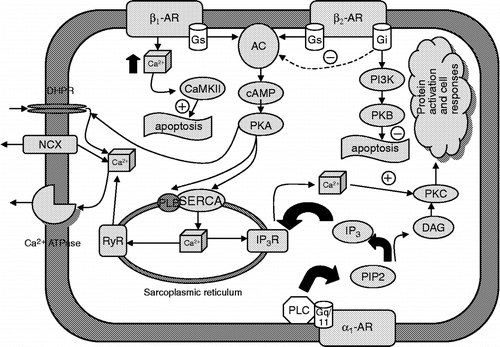
As mentioned above, the role of α2-adrenoceptors is modulatory rather than affecting cardiac function directly. α2-adrenoceptors mediate prejunctional inhibition of norepinephrine release. All α2-adrenoceptor subtypes inhibit adenylyl cyclase (AC), which results in decreased intracellular cAMP content ().
Important differences occur between the two “classical” heart β-adrenoceptors and the β3 subtype. The human β3-adrenoceptor gene contains introns, whereas the β1- and β2-adrenoceptor genes do not (Brodde and Michel Citation1999). Moreover, the β3-adrenoceptors have no phosphorylation sites for protein kinase A (PKA) and therefore they are considered as relatively insensitive to receptor desensitization in vitro and in vivo following activation with agonists (Gauthier et al. Citation2000).
The β1-adrenoceptors are considered as the main heart adrenoceptor subtype. Their role in cardiac physiology is stimulatory (the effects are positively inotropic, chronotropic, dromotropic and bathmotropic). Thus, stimulation of these receptors increases the strength of heart muscle contraction, the heart rate, increases the atrio–ventricular conduction velocity and increases the heart muscle excitability (the last two effects are sometimes considered as secondary and only inotropic and chronotropic effects are described). This receptor subtype couples to Gs proteins (), stimulates AC, which subsequently leads to increase in cAMP intracellular levels (), activation of PKA, and further to phosphorylation of several sarcolemmal proteins, including L-type Ca2 + channels and phospholamban, which regulates reticular Ca2 + -ATPase (Kaumann and Molenaar Citation1997). Phosphorylation of L-type Ca2 + channels promotes calcium influx and thus enhances contraction; phosphorylation of phospholamban may be involved in enhanced diastolic relaxation by increasing calcium uptake into the sarcoplasmic reticulum. Gs can also directly activate L-type calcium channels (it is not clear yet, whether this applies also for human myocardium, see Brodde and Michel Citation1999 for discussion).
From studies with cloned β2-adrenoceptors, it is known that the functions, coupling and signalling pathways resemble those of the β1 subtype (). Differences from β1-adrenoceptors that should be mentioned when considering the importance of β2-adrenoceptors in the heart include: β2-adrenoceptor stimulation (in contrast to β1-adrenoceptor stimulation) does not correlate well with increases in intracellular cAMP and does not lead to phosphorylation of phospholamban in these cells. Moreover, there are kinetic differences between β1- and β2-adrenoceptor-mediated activation of L-type calcium channels. As another difference, more effective coupling of β2-adrenoceptors to AC was observed, which might explain why adrenaline causes nearly identical increases in force of contraction via β1- or β2-adrenoceptor stimulation (Brodde and Michel Citation1999). Moreover, chronic stimulation of β1-adrenoceptors and β2-adrenoceptors elicits opposing effects on the fate of cardiomyocytes: β1-adrenoceptors induce hypertrophy and apoptosis; but β2-adrenoceptors promote cell survival (Zheng et al. Citation2004). The other difference between β1-adrenoceptors and β2-adrenoceptors lies in their differential role in regulating autonomic signalling: it has been demonstrated that heart rate variability in β1- and β2- KO mice shows significant differences (Ecker et al. Citation2006). In other words, this study demonstrated the specific roles of individual adrenoceptor subtypes in regulating both heart rate and heart rate variability. Finally, there is evidence that β2-adrenoceptors couple, in addition to the “classical” cascade, also to Gi proteins (Kilts et al. Citation2000). Thus, in addition to cAMP and calcium signalling pathways, β2-adrenoceptors activate guanylyl cyclase via Gi/G0 family proteins (Li et al. Citation2004). Stimulation of guanylyl cyclase causes an increase in cGMP levels, and subsequent activation of protein kinase G.
One of the intriguing questions in cardiac physiology is about the presence of another β-adrenoceptor subtype(s). The presence of β3-adrenoceptor in the heart is supported by these findings: β3-adrenoceptor mRNA has been found repeatedly in the heart (Gauthier et al. Citation2000; Myslivecek et al. Citation2006), the β3-adrenoceptor has been also found using immunohistochemical detection (De Matteis et al. Citation2002), and negative inotropic effects have also been repeatedly recorded when using β3-adrenoceptor agonists (Gauthier et al. Citation1996, Citation1998, Citation1999). Moreover, the importance of β3-adrenoceptors was demonstrated using mice over-expressing this receptor (Tavernier et al. Citation2003). On the other hand, Heubach et al. (Citation2002) did not find negative inotropic effects of adrenaline as mediated via β3-adrenoceptors. These receptors would couple to Gi proteins in the heart (), which have inhibitory effects on AC and decreases the amount of intracellular cAMP, while in fat tissue β3-adrenoceptors are coupled to Gs. It is supposed that effects of β3-adrenoceptors on heart rate are mediated via increased nitric oxide synthase activity (Rozec and Gauthier Citation2006). Nevertheless, physiological relevance of these receptors in the heart remains to be elucidated.
During the 1990s it was believed that another, fourth, putative β4-adrenoceptor is present in the heart of several species (rat, mouse, ferret, human–Molenaar et al. Citation1997). Nevertheless, at the beginning of the new millennium it has been recognized that these binding sites are a rather low affinity state of β1-adrenoceptors rather than another β-adrenoceptor subtype (Granneman Citation2001). On the other hand, some results (Santos and Spadari-Bratfisch Citation2001; Santos et al. Citation2005) and those from our laboratory (Myslivecek et al. Citation2003) indicated that the situation with regard to low-affinity binding sites could be more complicated as in some conditions (stress), these sites show behavior distinct from β1-adrenoceptors.
Catecholamine action through receptors depends on several factors, according to general receptor occupational theory (Kenakin Citation2004). Specifically, action depends on (1) relative receptor density in the target tissue; (2) intrinsic efficacy (the ability to activate the intracellular signalling pathways); (3) receptor affinity, expressed as equilibrium dissociation constant of the drug (catecholamine)-receptor complex. Thus, when intrinsic activity and receptor density is higher and equilibrium dissociation constant is lower, drug (catecholamine) action is stronger. All these factors are considered when analyzing effects of receptor activation in target tissue, but the above is a simplification, which may lead to misinterpretation of results (Kenakin Citation2004). Considering the effects of catecholamines on heart adrenoceptors:
The relative amount of receptor in the tissue, and region-specific differences in receptor densities. β1-adrenoceptors are more abundant than β2-adrenoceptors and much more abundant than α1-adrenoceptors and β3-adrenoceptors, but there are some regions in which the relative density of β2-adrenoceptors is higher than that of β1-adrenoceptors (Brodde and Michel Citation1999; Myslivecek et al. Citation2006).
Receptor affinity. As can be deduced from , for some receptor subtypes expressed in the heart, there are substantial differences among receptor subtypes affinities to epinephrine or norepinephrine. Moreover, differences can be observed also in affinity to catecholamines for specific subtype (for example, β2-adrenoceptors have higher affinity to epinephrine than to norepinephrine).
Receptor function, or post-receptor coupling. Different adrenoceptors have different functions, e.g. some are cardiostimulatory (β1-adrenoceptor) and others are cardioinhibitory (β3-adrenoceptors). The subtype with complex function (α1-adrenoceptors) is also expressed in the heart. It has been mentioned above that adrenoceptors couple to specific G proteins and signalling systems. Despite that, there is evidence that β2-adrenoceptor couple in addition to the “classical” cascade also to Gi proteins (Kilts et al. Citation2000). This finding can extend our understanding of peculiarities of cardiac signaling, since disruption of coupling of these receptors to Gi enhances their ability to stimulate AC. Also, it should be mentioned that coupling of β3-adrenoceptors is “atypical” as they normally couple to Gs and not to Gi.
Adrenoceptors, similarly to other GPCRs undergo changes when stimulated more or less. Typically, with increased receptor stimulation receptors undergo desensitization-internalization and down-regulation (Morris and Malbon Citation1999; Fukunaga et al. Citation2006). However, there are some differences in the receptor regulation processes among adrenoceptor subtypes in the heart. As mentioned above, β3-adrenoceptors are relatively insensitive to receptor desensitization (Gauthier et al. Citation2000). There is also a difference in the regulatory processes between β1-adrenoceptors and β2-adrenoceptors. Although with stimulation β1-adrenoceptors are internalized like β2-adrenoceptors, there is no reduction in receptor number, but β1-adrenoceptors accumulate inside the cells. Thus, while β2-adrenoceptors undergo lysosomal degradation, the β1-adrenoceptors do not (Liang et al. Citation2003).
Besides other systems, stress affects also cardiac adrenoceptors, and alter signalling (Santos and Spadari-Bratfisch Citation2006). Moreover, balance between the sympathetic and parasympathetic systems can also be disturbed as a consequence of stress (Farah et al. Citation2006). Finally, stress changes receptor densities (Myslivecek et al. Citation2004).
An intriguing finding is that just two catecholamines (epinephrine, norepinephrine) have many “target” -receptors that differ greatly in the functional consequences of receptor activation: while β1- and β2-adrenoceptors cause cardiostimulation (Kaumann and Molenaar Citation1997), β3-adrenoceptors are responsible for cardioinhibition (Gauthier et al. Citation2000) and α1-adrenoceptors contribute to enhanced inotropy (Xiao et al. Citation2006), while the role of α2-adrenoceptors is in the regulation of catecholamine release (Docherty Citation2002). Moreover, the heart is equipped with a very efficient system of receptors that binds transmitters and activates intracellular signalling not in parallel, but as a consequence of differences in affinity, coupling, signalling and regulatory events. Conceivably, catecholamines first activate β1-adrenoceptors, than β2-adrenoceptors and α1-adrenoceptors (which are less expressed) and if the presence of catecholamines persists, a mechanism of cardioinhibition through β3-adrenoceptors may then be activated. It could be hypothesized that β3-adrenoceptors are “back-up” receptors activated during extreme or stressful conditions (Pelat et al. Citation2003). This hypothesis awaits further investigation.
Catecholamines and calcium release through the inositol 1,4,5-trisphosphate receptors
The catecholamines norepinephrine and epinephrine can modify calcium homeostasis, either by increasing cytoplasmic calcium by stimulating entry from the extracellular space through L-type calcium channels, or from intracellular calcium stores (sarcoplasmic reticulum) through inositol 1,4,5-trisphosphate (IP3) receptors (IP3R; ). Calcium is a key molecule controlling several cellular processes, from fertilization to cell death, and in all cell types. In excitable and contracting cells, such as cardiomyocytes, Ca2 + controls muscle contractility. The spatial and temporal segregation of Ca2 + concentrations are central to maintain its concentration gradients across the cells and the cellular compartments for normal function.
Together with ryanodine receptors, IP3 receptors are able to release calcium from the intracellular stores. While ryanodine receptors are high capacity channels, which are able to release huge amount of calcium and participate directly in the process of excitation–contraction coupling, IP3 receptors can release only a moderate amount of calcium and the physiological relevance of calcium release through this mechanism is not yet clear. Within cardiomyocytes, IP3 receptor mRNA levels are approximately 50-fold lower than levels of the cardiac ryanodine receptor mRNA (Moschella and Marks Citation1993). In the heart, all three types of IP3 receptor are found, although with different predominance. We reported that there is an abundant expression of the type 1 IP3 receptors in the cardiac atria in contrast to low expression in the ventricles (Lencesova et al. Citation2002). The type 1 IP3 receptor is most abundant in the cardiac ganglia and neuronal cells (Slavikova et al. Citation2006), while the type 2 IP3 receptor is most prevalent in cardiomyocytes (Perez et al. Citation1997).
The functional role of IP3 in the heart is still unclear. Several hormones can activate IP3 production in cardiomyocytes and some of their inotropic, chronotropic and arrhythmogenic effects may be due to the calcium release mediated by IP3 receptors (Lipp et al. Citation2000). Compared to ryanodine receptors, responses to IP3 are slow and weak, and the calcium so released does not contribute to calcium induced-calcium release. Thus, IP3 is less likely to be important in regulating beat-to-beat changes in intracellular calcium under physiological conditions. Chronic mechanical overload of the atrial myocardium increased IP3 receptor expression especially in patients with chronic atrial fibrillation (Yamada et al. Citation2002). Apart from the localization of IP3 receptors in normal atrial tissue, several reports pointed out a characteristic up-regulation of IP3 receptor mRNAs in hearts from hypertrophy and end-stage heart failure patients (Gutstein and Marks Citation1997), while mRNA for ryanodine receptors was down-regulated in the same tissue (Marks Citation2000). The IP3 signaling cassette might be also a promising new target for understanding and managing atrial arrhythmia (Mackenzie et al. Citation2002). Thus, up-regulation of IP3 receptors may be important in modulating intracellular calcium homeostasis and initiating and perpetuating atrial fibrillation. Furthermore, Xu et al. (Citation2000) proposed that decreased levels of the IP3 receptors might play a role in cardioprotection.
Inositol 1,4,5-trisphosphate receptors and stress
Stress is considered to be a major contributor to the development of cardiovascular disease and psychiatric illness. Therefore, it is crucial to clarify mechanisms involved in the conversion of brief beneficial responses to stressors into prolonged detrimental consequences. We have already shown different effects of single and repeated immobilization stress on gene expression of the IP3 receptors. After a single immobilization stress, gene expression of the type 1 IP3 receptor was increased (Lencesova et al. Citation2002; Krizanova et al. Citation2005), while after repeated immobilization stress (seven times), both gene expression and protein of the type 1 IP3 receptor was decreased significantly in the left atrium of rats (Krepsova et al. Citation2004). Exposure to a relatively weak stressor, cold for 24 h, was not able to increase gene expression of IP3 receptors (Krizanova et al. Citation2005). However, when rats were exposed to cold (4°C) for seven days, gene expression of the type 1 IP3 receptor was significantly upregulated. Animals exposed to cold for 28 days became cold-adapted and did not show any difference in the type 1 IP3 receptor mRNA level compared to non-stressed controls (Krizanova et al. Citation2005).
Several questions arise from these observations. Firstly, what elements are involved in the stress-induced increase in expression of IP3R1 and also IP3R2? Since the promoter region of the type 1 IP3R contains a glucocorticoid responsive element, we proposed involvement of glucocorticoids in this process. We have shown that adrenalectomy completely abolished the immobilization-induced increase in both IP3R1 and IP3R2 mRNAs, but did not affect basal gene expression of the respective proteins in control conditions (Lencesova et al. Citation2002). We also tested the effect of catecholamines using the neurotoxin 6-hydroxydopamine. When 6-hydroxydopamine was given to animals, norepinephrine and subsequently epinephrine levels were markedly reduced. The partial depletion of catecholamines decreased significantly mRNA and protein levels of the type 1 IP3 receptors in the heart, in both control conditions and after exposure to immobilization stress for 2 h (Jurkovicova et al. Citation2006). A similar effect was observed on gene expression for the type 2 and 3 IP3 receptors. These findings provide a molecular basis for the hypothesis that the mechanism of adrenergic modulation in the heart involves IP3 receptors. It was previously reported that changes in expression profiles for other genes in cardiomyocytes were observed after norepinephrine infusion (Ponicke et al. Citation2001; Li et al. Citation2003). By analogy to dihydropyridine receptors, for which norepinephrine treatment alters mRNA levels through the AP1 transcription factor (Deelman et al. Citation1998), it might be speculated that the effect of depleted norepinephrine is effected through AP1. The AP1 transcription factor is modulated by several proteins, products of immediate-early genes. We have shown that mice with knockout of the c-fos gene express significantly lower levels of the IP3R1 mRNA compared to their heterozygous littermates (). Interestingly, this decrease was more profound in male atria than in female atria. Nevertheless, a single immobilization stress elicited a large increase in the IP3R1 mRNA in male left atria. This increase was almost twice that induced by immobilization in control mice (). These results suggest that c-fos, and Fos protein, participates in regulating gene expression of the type 1 IP3 receptor in physiological conditions, but it also might affect the increased expression of these receptors induced by immobilization stress.
Physiological relevance of inositol 1,4,5-trisphosphate receptors in the heart
The second issue to be addressed is the physiological relevance of altered expression of the IP3 receptor genes in the heart. It is generally accepted that IP3 receptors do not play a major role in cardiac excitation–contraction coupling, although they could be involved in physiological modulation of cardiac contractility in response to pharmacological agents and hormones (Marks Citation1997). As mentioned above, IP3 receptors are up-regulated in several cardiovascular diseases, and also during single immobilization stress exposure and seven-day cold exposure. In contrast, decreased levels of IP3 receptors were observed after repeated immobilization stress (two hours daily for 7 days) in the heart (Krepsova et al. Citation2004), stellate ganglia (Micutkova et al. Citation2003) and kidney (Zacikova et al. Citation2000). Increased and decreased levels of IP3 receptors have different physiological consequences.
Lack of type 1 IP3 receptors was studied in IP3R1 gene knockout mice (Matsumoto and Nagata Citation1999). These mice were rarely born alive, indicating that IP3R1 has some important functions during embryonic development. Liveborn exhibited severe neurological symptoms, ataxia and epilepsy, and were shown to be deficient in cerebellar long-term depression. These results suggest that IP3 receptors are crucial in neonates, while in adulthood they have regulatory role mainly in neuronal tissue.
Apoptosis is a “suicide” program highly conserved throughout evolution, and present in all metazoan cells (Evan et al. Citation1995). Apoptosis plays an important role in normal development and in pathological processes, such as heart failure and atherosclerosis. It has been proposed that elevation of cytoplasmic calcium concentration is a regulatory signal involved in apoptosis (Cohen and Duke Citation1984; Jones et al. Citation1989). IP3 has been reported to be required for signaling pathways leading to apoptotic cell death in lymphoid cells, when apoptosis is induced by a number of different agents, including glucocorticoids (Jayaraman and Marks Citation1997). Recent studies have demonstrated that IP3 receptors can also be activated during apoptosis independently of the production of IP3. In the case of type 1 IP3 receptors, this may occur following proteolysis by activated caspase 3.The type 1 IP3 receptor has a single highly conserved DEVD cleavage site, which is caspase 3 specific. Truncation of IP3 receptors by caspase 3 at this position removes a large portion of the protein, including the amino-terminal IP3-binding region and other regulatory domains (Hanson et al. Citation2004). The part that remains in the endoplasmic reticulum membrane is a constitutively active channel that continuously leaks calcium (Nakayama et al. Citation2004).
In an alternative model for IP3 receptor activation during apoptosis that is not reliant on caspase cleavage, cytochrome C released from mitochondria is suggested to control calcium movement through IP3 receptors. Cytochrome C was shown to bind IP3 receptors and reduce the calcium-dependent inhibition of channel opening (Boehning et al. Citation2003). These studies all point to a crucial role of IP3 receptors in the generation of calcium signals that drive cells into apoptosis.
Conclusion
In summary, the catecholamine synthesizing pathway together with calcium is able to secure a wide variety of processes in the heart. Stress can affect not only production of catecholamines, but also adrenoceptors and IP3 receptors. In this review we described in particular communication by the adrenergic receptor pathway through the release of calcium from intracellular stores, mainly through IP3 receptors. Recently we have found that the amount of the type 1 IP3 receptors is decreased when catecholamines are partially depleted. This finding provides a molecular basis for the hypothesis that a mechanism of adrenergic modulation in the heart involves the type 1 IP3 receptors. Nevertheless, physiological relevance of this signaling remains to be elucidated. Since decreased expression and activity of IP3 receptors inhibits apoptosis, this allows the speculation that an increase in IP3 receptors due to increased and prolonged adrenergic modulation might be one of the stimuli involved in the process of apoptosis. Our further research will be oriented to clarify this issue.
Acknowledgements
This work was supported by grants Genomika SP 51/028 08 00/0280802, VEGA 2/6078, 2/5125 and 2/7123, GAUK11/06 and APVV 51-027-404.
References
- Axelrod J. Factors affecting the metabolism of epinephrine and other amines. Res Publ Assoc Res Nerv Ment Dis 1962; 40: 280–287
- Beckers F, Ramaekers D, Speijer G, Ector H, Vanhaecke J, Verheyden B, Van Cleemput J, Droogne W, Van de Werf F, Aubert AE. Different evolutions in heart rate variability after heart transplantation: 10-year follow-up. Transplantation 2004; 78: 1523–1531
- Berkowitz DE, Price DT, Bello EA, Page SO, Schwinn DA. Evidence for species heterogeneity and implications for human pharmacology. Anesthesiology 1994; 81: 1235–1244
- Boehning D, Patterson RL, Sedaghat L, Glebova NO, Kurosaki T, Snyder SH. Cytochrome c binds to inositol (1,4,5) trisphosphate receptors, amplifying calcium-dependent apoptosis. Nat Cell Biol 2003; 5: 1051–1061
- Bohn MC, Goldstein M, Black IB. Role of glucocorticoids in expression of the adrenergic phenotype in rat embryonic adrenal gland. Dev Biol 1981; 82: 1–10
- Brodde OE, Michel MC. Adrenergic and muscarinic receptors in the human heart. Pharmacol Rev 1999; 51: 651–690
- Bruhn TO, Sutton RE, Rivier CL, Vale WW. Corticotropin-releasing factor regulates proopiomelanocortin messenger ribonucleic acid levels in vivo. Neuroendocrinology 1984; 39: 170–175
- Bylund DB, Eikenberg DC, Hieble JP, Langer SZ, Lefkowitz RJ, Minneman KP, Molinoff PB, Ruffolo RR, Jr., Trendelenburg U. International Union of Pharmacology nomenclature of adrenoceptors. Pharmacol Rev 1994; 46: 121–136
- Chen XH, Harden KT, Nicholas RA. Molecular cloning and characterization of a novel β-adrenergic receptor. J Biol Chem 1994; 269: 24810–24819
- Cohen JJ, Duke RC. Glucocorticoid activation of a calcium-dependent endonuclease in thymocyte nuclei leads to cell death. J Immunol 1984; 132: 38–42
- Culman J, Torda T, Weise VK. A radiometric assay for phenylethanolamine N-methyltransferase and catechol O-methyltransferase in a single tissue sample: Application to rat hypothalamic nuclei, pineal gland, and heart. Anal Biochem 1987; 164: 345–354
- Deelman LE, Jonk LJ, Henning RH. The isolation and characterization of the promoter of the human type 1 inositol 1,4,5-trisphosphate receptor. Gene 1998; 207: 219–225
- De Matteis R, Arch JR, Petroni ML, Ferrari D, Cinti S, Stock MJ. Immunohistochemical identification of the beta(3)-adrenoceptor in intact human adipocytes and ventricular myocardium: Effect of obesity and treatment with ephedrine and caffeine. Int J Obes Relat Metab Disord 2002; 26: 1442–1450
- Docherty JR. Age-related changes in adrenergic neuroeffector transmission. Auton Neurosci 2002; 96: 8–12
- Ebert SN, Thompson RP. Embryonic epinephrine synthesis in the rat heart before innervation: Association with pacemaking and conduction tissue development. Circ Res 2001; 88: 117–124
- Ebert SN, Baden JM, Mathers LH, Siddall BJ, Wong DL. Expression of phenylethanolamine n-methyltransferase in the embryonic rat heart. J Mol Cell Cardiol 1996; 28: 1653–1658
- Ecker PM, Lin CC, Powers J, Kobilka BK, Dubin AM, Bernstein D. Effect of targeted deletions of beta1- and beta2-adrenergic-receptor subtypes on heart rate variability. Am J Physiol Heart Circ Physiol 2006; 290: H192–H199
- Elayan HH, Kennedy B, Ziegler MG. Epinephrine synthesis by an N-methyltransferase in rat liver. Gastroenterology 1990a; 98: 152–155
- Elayan HH, Kennedy BP, Ziegler MG. Cardiac atria and ventricles contain different inducible adrenaline synthesising enzymes. Cardiovasc Res 1990b; 24: 53–56
- Endoh M, Hiramoto T, Ishihata A, Takanashi M, Inui J. Myocardial alpha 1-adrenoceptors mediate positive inotropic effect and changes in phosphatidylinositol metabolism. Species differences in receptor distribution and the intracellular coupling process in mammalian ventricular myocardium. Circ Res 1991; 68: 1179–1190
- Esler M, Eisenhofer G, Dart A, Chin J, Cox H, Lambert G, Jennings G. Adrenaline release by the human heart. Clin Exp Pharmacol Physiol 1991; 18: 67–70
- Esler M, Jennings G, Lambert G, Meredith I, Horne M, Eisenhofer G. Overflow of catecholamine neurotransmitters to the circulation: Source, fate, and functions. Physiol Rev 1990; 70: 963–985
- Evan GI, Brown L, Whyte M, Harrington E. Apoptosis and the cell cycle. Curr Opin Cell Biol 1995; 7: 825–834
- Farah VM, Joaquim LF, Morris M. Stress cardiovascular/autonomic interactions in mice. Physiol Behav 2006, (in press)
- Ford AP, Daniels DV, Chang DJ, Gever JR, Jasper JR, Lesnick JD, Clarke DE. Pharmacological pleiotropism of the human recombinant alpha1A-adrenoceptor: Implications for alpha1-adrenoceptor classification. Br J Pharmacol 1997; 121: 1127–1135
- Fukunaga S, Setoguchi S, Hirasawa A, Tsujimoto G. Monitoring ligand-mediated internalization of G protein-coupled receptor as a novel pharmacological approach. Life Sci 2006, (in press)
- Gauthier C, Langin D, Balligand JL. Beta3-adrenoceptors in the cardiovascular system. Trends Pharmacol Sci 2000; 21: 426–431
- Gauthier C, Leblais V, Kobzik L, Trochu JN, Khandoudi N, Bril A, Balligand JL, Le Marec H. The negative inotropic effect of beta3-adrenoceptor stimulation is mediated by activation of a nitric oxide synthase pathway in human ventricle. J Clin Invest 1998; 102: 1377–1384
- Gauthier C, Tavernier G, Charpentier F, Langin D, Le Marec H. Functional beta3-adrenoceptor in the human heart. J Clin Invest 1996; 98: 556–562
- Gauthier C, Tavernier G, Trochu JN, Leblais V, Laurent K, Langin D, Escande D, Le Marec H. Interspecies differences in the cardiac negative inotropic effects of beta(3)-adrenoceptor agonists. J Pharmacol Exp Ther 1999; 290: 687–693
- Goncalvesova E, Micutkova L, Mravec B, Ksinantova L, Krizanova O, Fabian J, Kvetnansky R. Changes in gene expression of phenylethanolamine N-methyltransferase in the transplanted human heart. Ann N Y Acad Sci 2004; 1018: 430–436
- Granneman JG. The putative beta4-adrenergic receptor is a novel state of the beta1-adrenergic receptor. Am J Physiol Endocrinol Metab 2001; 280: E199–E202
- Gutstein DE, Marks AR. Role of inositol 1,4,5-trisphosphate receptors in regulating apoptotic signaling and heart failure. Heart Vessels 1997, Suppl 12: 53–57
- Hanson CJ, Bootman MD, Roderick HL. Cell signalling: IP3 receptors channel calcium into cell death. Curr Biol 2004; 14: R933–R935
- Heubach JF, Rau T, Eschenhagen T, Ravens U, Kaumann AJ. Physiological antagonism between ventricular beta 1-adrenoceptors and alpha 1-adrenoceptors but no evidence for beta 2- and beta 3-adrenoceptor function in murine heart. Br J Pharmacol 2002; 136: 217–229
- Huang MH, Bahl JJ, Wu Y, Hu F, Larson DF, Roeske WR, Ewy GA. Neuroendocrine properties of intrinsic cardiac adrenergic cells in fetal rat heart. Am J Physiol Heart Circ Physiol 2005; 288: H497–H503
- Huang MH, Friend DS, Sunday ME, Singh K, Haley K, Austen KF, Kelly RA, Smith TW. An intrinsic adrenergic system in mammalian heart. J Clin Invest 1996; 98: 1298–1303
- Jayaraman T, Marks AR. T cells deficient in inositol 1,4,5-trisphosphate receptor are resistant to apoptosis. Mol Cell Biol 1997; 17: 3005–3012
- Jeong J, Choi S, Gu C, Lee H, Park S. Genomic structure and promoter analysis of the mouse EphA8 receptor tyrosine kinase gene. DNA Cell Biol 2000; 19: 291–300
- Jones OT, Kune DL, Angelides KJ. Localization and mobility of omega-conotoxin-sensitive Ca2+ channels in hippocampl CA1 neurons. Science 1989; 244: 1189–1193
- Jurkovicova D, Kubovcakova L, Hudecova S, Kvetnansky R, Krizanova O. Adrenergic modulation of the type 1 IP3 receptors in the rat heart. Biochim Biophys Acta 2006; 1763: 18–24
- Kaumann AJ, Molenaar P. Modulation of human cardiac function through 4 beta-adrenoceptor populations. Naunyn Schmiedebergs Arch Pharmacol 1997; 355: 667–681
- Kaumann AJ, Engelhardt S, Hein L, Molenaar P, Lohse M. Abolition of ( − )-CGP 12177-evoked cardiostimulation in double beta1/beta2-adrenoceptor knockout mice. Obligatory role of beta1-adrenoceptors for putative beta4-adrenoceptor pharmacology. Naunyn Schmiedebergs Arch Pharmacol 2001; 363: 87–93
- Kenakin T. Principles: Receptor theory in pharmacology. Trends Pharmacol Sci 2004; 25: 186–192
- Kennedy B, Ziegler MG. Cardiac epinephrine synthesis. Regulation by a glucocorticoid. Circulation 1991; 84: 891–895
- Kennedy B, Ziegler MG. Ontogeny of epinephrine metabolic pathways in the rat: Role of glucocorticoids. Int J Dev Neurosci 2000; 18: 53–59
- Kennedy B, Bigby TD, Ziegler MG. Nonadrenal epinephrine-forming enzymes in humans. Characteristics, distribution, regulation, and relationship to epinephrine levels. J Clin Invest 1995; 95: 2896–2902
- Kilts JD, Gerhardt MA, Richardson MD, Sreeram G, Mackensen GB, Grocott HP, White WD, Davis RD, Newman MF, Reves JG, Schwinn DA, Kwatra MM. Beta(2)-adrenergic and several other G protein-coupled receptors in human atrial membranes activate both G(s) and G(i). Circ Res 2000; 87: 705–709
- Krepsova K, Micutkova L, Novotova M, Kubovcakova L, Kvetnansky R, Krizanova O. Repeated immobilization stress decreases mRNA and protein levels of the type 1 IP3 receptor in rat heart. Ann N Y Acad Sci 2004; 1018: 339–344
- Krizanova O, Kvetnansky R, Jurkovicova D. Effect of two distinct stressors on gene expression of the type 1 IP3 receptors. Gen Physiol Biophys 2005; 24: 237–246
- Krizanova O, Micutkova L, Jelokova J, Filipenko M, Sabban E, Kvetnansky R. Existence of cardiac PNMT mRNA in adult rats: Elevation by stress in a glucocorticoid-dependent manner. Am J Physiol Heart Circ Physiol 2001; 281: H1372–H1379
- Kvetnansky R, Kubovcakova L, Tillinger A, Micutkova L, Krizanova O, Sabban EL. Gene expression of phenylethanolamine n-methyltransferase in corticotropin-releasing hormone knockout mice during stress exposure. Cell Mol Neurobiol 2006, (in press)
- Kvetnansky R, Micutkova L, Kubovcakova L, Sabban EL, Palkovits M, Krizanova O. Localization and regulation of phenylethanolamine N-methyltransferase gene expression in the heart of rats and mice during stress. Ann N Y Acad Sci 2004; 1018: 405–417
- Kvetnansky R, Weise VK, Thoa NB, Kopin IJ. Effects of chronic guanethidine treatment and adrenal medullectomy on plasma levels of catecholamines and corticosterone in forcibly immobilized rats. J Pharmacol Exp Ther 1979; 209: 287–291
- Lencesova L, Ondrias K, Micutkova L, Filipenko M, Kvetnansky R, Krizanova O. Immobilization stress elevates IP(3) receptor mRNA in adult rat hearts in a glucocorticoid-dependent manner. FEBS Lett 2002; 531: 432–436
- Liang W, Austin S, Hoang Q, Fishman PH. Resistance of the human beta 1-adrenergic receptor to agonist-mediated down-regulation. Role of the C terminus in determining beta-subtype degradation. J Biol Chem 2003; 278: 39773–39781
- Li P, Li J, Feng X, Hou R, Han C, Zhang Y. Gene expression profile of cardiomyocytes in hypertrophic heart induced by continuous norepinephrine infusion in the rats. Cell Mol Life Sci. 2003; 60: 2200–9
- Li F, De Godoy M, Rattan S. Role of adenylate and guanylate cyclases in beta1-, beta2-, and beta3-adrenoceptor-mediated relaxation of internal anal sphincter smooth muscle. J Pharmacol Exp Ther 2004; 308: 1111–1120
- Lipp P, Laine M, Tovey SC, Burrell KM, Berridge MJ, Li W, Bootman MD. Functional InsP3 receptors that may modulate excitation–contraction coupling in the heart. Curr Biol 2000; 10: 939–942
- Mackenzie L, Bootman MD, Laine M, Berridge MJ, Thuring J, Holmes A, Li WH, Lipp P. The role of inositol 1,4,5-trisphosphate receptors in Ca(2+) signalling and the generation of arrhythmias in rat atrial myocytes. J Physiol 2002; 541: 395–409
- Marks AR. Intracellular calcium-release channels: Regulators of cell life and death. Am J Physiol 1997; 272: H597–H605
- Marks AR. Cardiac intracellular calcium release channels: Role in heart failure. Circ Res 2000; 87: 8–11
- Matsumoto M, Nagata E. Type 1 inositol 1,4,5-trisphosphate receptor knock-out mice: Their phenotypes and their meaning in neuroscience and clinical practice. J Mol Med 1999; 77: 406–411
- Micutkova L, Krepsova K, Sabban E, Krizanova O, Kvetnansky R. Modulation of catecholamine-synthesizing enzymes in the rat heart by repeated immobilization stress. Ann N Y Acad Sci 2004; 1018: 424–429
- Micutkova L, Kvetnansky R, Krizanova O. Repeated immobilization stress reduces the gene expression of the type 1 and 2 IP3 receptors in stellate ganglia. Neurochem Int 2003; 43: 557–561
- Molenaar P, Sarsero D, Kaumann AJ. Proposal for the interaction of non-conventional partial agonists and catecholamines with the “putative beta 4-adrenoceptor” in mammalian heart. Clin Exp Pharmacol Physiol 1997; 24: 647–656
- Morris AJ, Malbon CC. Physiological regulation of G protein-linked signaling. Physiol Rev 1999; 79: 1373–1430
- Moschella MC, Marks AR. Inositol 1,4,5-trisphosphate receptor expression in cardiac myocytes. J Cell Biol 1993; 120: 1137–1146
- Muglia LJ, Jacobson L, Weninger SC, Karalis KP, Jeong K, Majzoub JA. The physiology of corticotropin-releasing hormone deficiency in mice. Peptides 2001; 22: 725–731
- Myslivecek J, Novakova M, Palkovits M, Krizanova O, Kvetnansky R. Distribution of mRNA and binding sites of adrenoceptors and muscarinic receptors in the rat heart. Life Sci 2006; 79: 112–120
- Myslivecek J, Ricny J, Kolar F, Tucek S. The effects of hydrocortisone on rat heart muscarinic and adrenergic alpha 1, beta 1 and beta 2 receptors, propranolol-resistant binding sites and on some subsequent steps in intracellular signalling. Naunyn Schmiedebergs Arch Pharmacol 2003; 368: 366–376
- Myslivecek J, Ricny J, Palkovits M, Kvetnansky R. The effects of short-term immobilization stress on muscarinic receptors, beta-adrenoceptors, and adenylyl cyclase in different heart regions. Ann N Y Acad Sci 2004; 1018: 315–322
- Nakayama T, Hattori M, Uchida K, Nakamura T, Tateishi Y, Bannai H, Iwai M, Michikawa T, Inoue T, Mikoshiba K. The regulatory domain of the inositol 1,4,5-trisphosphate receptor is necessary to keep the channel domain closed: Possible physiological significance of specific cleavage by caspase 3. Biochem J 2004; 377: 299–307
- Pelat M, Verwaerde P, Galitzky J, Lafontan M, Berlan M, Senard JM, Montastruc JL. High isoproterenol doses are required to activate beta3-adrenoceptor-mediated functions in dogs. J Pharmacol Exp Ther 2003; 304: 246–253
- Perez PJ, Ramos-Franco J, Fill M, Mignery GA. Identification and functional reconstitution of the type 2 inositol 1,4,5-trisphosphate receptor from ventricular cardiac myocytes. J Biol Chem 1997; 272: 23961–23969
- Ponicke K, Schluter KD, Heinroth-Hoffmann I, Seyfarth T, Goldberg M, Osten B, Piper HM, Brodde OE. Noradrenaline-induced increase in protein synthesis in adult rat cardiomyocytes: Involvement of only alpha1A-adrenoceptors. Naunyn Schmiedebergs Arch Pharmacol 2001; 364: 444–453
- Rowan RA, Billingham ME. Myocardial innervation in long-term heart transplant survivors: A quantitative ultrastructural survey. J Heart Transplant 1988; 7: 448–452
- Rozec B, Gauthier C. beta3-adrenoceptors in the cardiovascular system: Putative roles in human pathologies. Pharmacol Ther 2006; 111: 652–673
- Sabban EL, Hiremagalur B, Nankova B, Kvetnansky R. Molecular biology of stress-elicited induction of catecholamine biosynthetic enzymes. Ann N Y Acad Sci 1995; 771: 327–338
- Sabban EL, Nankova BB, Serova LI, Hiremagalur B, Rusnak M, Saez E, Spiegelman B, Kvetnansky R. Regulation of gene expression of catecholamine biosynthetic enzymes by stress. Adv Pharmacol 1998; 42: 564–567
- Santos IN, Spadari-Bratfisch RC. Chronotropic response to (+/ − )-CGP12177 in right atria of stressed rats. Can J Physiol Pharmacol 2001; 79: 393–399
- Santos IN, Spadari-Bratfisch RC. Stress and cardiac beta adrenoceptors. Stress 2006; 9: 69–84
- Santos IN, Sumitame M, Caceres VM, Moreira MF, Krieger MH, Spadari-Bratfisch RC. Evidence for two atypical conformations of beta-adrenoceptors and their interaction with Gi proteins. Eur J Pharmacol 2005; 513: 109–118
- Schmid W, Cole TJ, Blendy JA, Schutz G. Molecular genetic analysis of glucocorticoid signalling in development. J Steroid Biochem Mol Biol 1995; 53: 33–35
- Shah SD, Tse TF, Clutter WE, Cryer PE. The human sympathochromaffin system. Am J Physiol 1984; 247: E380–E384
- Skeberdis VA. Structure and function of beta3-adrenergic receptors. Medicina (Kaunas) 2004; 40: 407–413
- Slavikova J, Dvorakova M, Reischig J, Palkovits M, Ondrias K, Tarabova B, Lacinova L, Kvetnansky R, Marks A, Krizanova O. IP3 type 1 receptors in the heart: Their predominance in atrial walls with ganglion cells. Life Sci 2006; 78: 1598–1602
- Slavikova J, Kuncova J, Reischig J, Dvorakova M. Catecholaminergic neurons in the rat intrinsic cardiac nervous system. Neurochem Res 2003; 28: 593–598
- Tai TC, Claycomb R, Her S, Bloom AK, Wong DL. Glucocorticoid responsiveness of the rat phenylethanolamine N-methyltransferase gene. Mol Pharmacol 2002; 61: 1385–1392
- Takahashi M, Taniguchi T, Kanamaru H, Okada K, Muramatsu I. Pharmacological characterization of [3H]-JTH-601, a novel alpha1-adrenoceptor antagonist: Binding to recombinant human alpha1-adrenoceptors and human prostates. Life Sci 2000; 67: 2443–2451
- Tavernier G, Toumaniantz G, Erfanian M, Heymann MF, Laurent K, Langin D, Gauthier C. beta3-Adrenergic stimulation produces a decrease of cardiac contractility ex vivo in mice overexpressing the human beta3-adrenergic receptor. Cardiovasc Res 2003; 59: 288–296
- Teitelman G, Joh TH, Park D, Brodsky M, New M, Reis DJ. Expression of the adrenergic phenotype in cultured fetal adrenal medullary cells: Role of intrinsic and extrinsic factors. Dev Biol 1982; 89: 450–459
- Thomas SA, Matsumoto AM, Palmiter RD. Noradrenaline is essential for mouse fetal development. Nature 1995; 374: 643–646
- Tillinger A, Bruderova V, Kubovcakova L, Zeman M, Kopacek J, Novakova M, Kvetnansky R, Krizanova O. Gene expression of the phenylethanolamine N-methyltransferase is differently modulated in cardiac atria and ventricles. Gen Physiol Biophys 2006a; 25: 355–364
- Tillinger A, Novakova M, Pavlovicova M, Lacinova L, Zatovicova M, Pastorekova S, Krizanova O, Kvetnansky R. Modulation of gene expression of the PNMT in the heart during immobilization stress by 6-hydroxydopamine. Stress 2006b; 9: 207–213
- Torda T, Culman J, Petrikova M. Distribution of phenylethanolamine-N-methyltransferase in the rat heart: Effect of 6-hydroxydopamine. Eur J Pharmacol 1987; 141: 305–308
- Venihaki M, Majzoub J. Lessons from CRH knockout mice. Neuropeptides 2002; 36: 96–102
- Wong DL, Anderson LJ, Tai TC. Cholinergic and peptidergic regulation of phenylethanolamine N-methyltransferase gene expression. Ann N Y Acad Sci 2002; 971: 19–26
- Wong DL, Siddall BJ, Ebert SN, Bell RA, Her S. Phenylethanolamine N-methyltransferase gene expression: Synergistic activation by Egr-1, AP-2 and the glucocorticoid receptor. Brain Res Mol Brain Res 1998; 61: 154–161
- Xiao RP, Zhu W, Zheng M, Cao C, Zhang Y, Lakatta EG, Han Q. Subtype-specific alpha1- and beta-adrenoceptor signaling in the heart. Trends Pharmacol Sci 2006; 27: 330–337
- Xu Y, Menon V, Jugdutt BI. Cardioprotection after angiotensin II type 1 blockade involves angiotensin II type 2 receptor expression and activation of protein kinase C-epsilon in acutely reperfused myocardial infarction in the dog. Effect of UP269-6 and losartan on AT1 and AT2-receptor expression and IP3 receptor and PKCepsilon proteins. J Renin Angiotensin Aldosterone Syst 2000; 1: 184–195
- Yamada J, Ohkusa T, Nao T, Ueyama T, Yano M, Kobayashi S, Hamano K, Esato K, Matsuzaki M. Up-regulation of inositol 1,4, 5-trisphosphate receptor expression in atrial tissue in patients with chronic atrial fibrillation. J Cardiol 2002; 39: 57–58
- Zacikova L, Ondrias K, Kvetnansky R, Krizanova O. Identification of type 1 IP(3) receptors in the rat kidney and their modulation by immobilization stress. Biochim Biophys Acta 2000; 1466: 16–22
- Zheng M, Han QD, Xiao RP. Distinct beta-adrenergic receptor subtype signaling in the heart and their pathophysiological relevance. Sheng Li Xue Bao 2004; 56: 1–15
- Zhou QY, Quaife CJ, Palmiter RD. Targeted disruption of the tyrosine hydroxylase gene reveals that catecholamines are required for mouse fetal development. Nature 1995; 374: 640–643
- Zimmer HG. Catecholamine-induced cardiac hypertrophy: Significance of proto-oncogene expression. J Mol Med 1997; 75: 849–859