Abstract
Perinatal asphyxia is a significant contributor to neonatal brain injury. However, there is significant variability in neurological outcome in neonates after global hypoxia–ischemia. The aims of this study were to identify which physiological response/s during global hypoxia–ischemia influence the severity of brain injury and to assess their relative importance.
Hypoxia/hypercapnia was induced in 20 anaesthetized piglets by reducing the inspired oxygen fraction to 10% and the ventilation rate from 30 to 10 breaths per minute for 45 min. Neurological outcome was assessed using functional markers including cerebral function amplitude (via electroencephalography) and cerebral impedance, and the structural marker microtubule associated protein-2 by immunohistochemistry at 6 h post hypoxia.
Significant variability in neurological outcome was observed following the constant hypoxia/hypercapnia insult. There was a high degree of variability in cardiovascular function (mean arterial blood pressure and heart rate) and serum cortisol concentrations in response to hypoxia. More effective maintenance of cardiovascular function and higher serum cortisol concentrations were associated with a better outcome. These two variables were strongly associated with neurological outcome, and together explained 68% of the variation in the severity of neurological outcome. The variability in the cardiovascular and cortisol responses to hypoxia may be a more important determinant of neurological outcome then previously recognized.
Abbreviations | ||
ANS | = | autonomic nervous system |
BPM | = | beats per minute |
CBF | = | cerebral blood flow |
CFM | = | cerebral function monitor |
HR | = | heart rate |
FiO2 | = | Fraction of inspired oxygen |
LDF | = | Laser Doppler flowmetry |
MAP | = | Mean arterial pressure |
MAP2 | = | Microtubule associated protein-2 |
PBS | = | Phosphate buffered saline |
SBF | = | Skin blood flow |
Introduction
Perinatal asphyxia occurs in four in every thousand live births (Vannucci and Perlman Citation1997) and carries significant risk of poor neurodevelopmental outcome (Shankaran et al. Citation1991). However there is substantial variation in the severity of brain injury, even amongst babies with similar clinical histories (Martinez et al. Citation1990). This variation is usually attributed to the uncertainties in relation to the duration and intensity of hypoxia (Williams et al. Citation1992; Citation1993), but there is mounting evidence that even following a uniform hypoxic stimulus the severity of brain injury is highly variable (Lavrijsen et al. Citation2005; Low Citation2004).
Variation in the systemic physiological response to hypoxia may be one potential mechanism to explain the observed variability in neurological outcome following an identical insult (Gunn et al. Citation1992; Mallard et al. Citation1992; Thoresen et al. Citation1996). For example, Mallard et al. (1995) demonstrated that following a standard insult, arterial blood pressure was highly variable with a standard deviation approaching 50% of the mean. Gunn et al. (Citation1992) also demonstrated that fetal sheep had variable responses to a uniform uterine artery occlusion, with some animals exhibiting sustained hypotension whilst others had no hypotension. Similarly, when the hypoxic insult is titrated to a defined physiological endpoint, there are broad differences in the time taken to reach this endpoint (Borke et al. Citation2004; Feet et al. Citation1998; Gunn et al. Citation1992; Ley et al. Citation2004), again suggesting that there is large variation in the individual global physiological responses to hypoxia. However, the extent to which the variability in physiological responses to a constant insult is associated with the observed variation in neurological outcome is currently unclear.
There is evidence that the ability to maintain cardiovascular function during hypoxia is an important determinant of neurological outcome (Bender et al. Citation2003; de Courten-Myers et al. Citation1989; Low Citation2004). A number of factors may contribute to the ability to maintain cardiovascular function during hypoxia. Increased activity of the sympathetic nervous system, including increased circulating catecholamine concentration, is essential for the maintenance of cardiac responses to hypoxia in the fetus and newborn (Seidler and Slotkin Citation1985; Downing et al. Citation1969; Jones et al. Citation1988; Jensen Citation1991). Cortisol administration has been shown to be neuroprotective in the fetus or newborn subjected to hypoxia (Liu et al. Citation1995; Barks et al. Citation1991), possibly via sustaining cardiovascular function (Kolanowski and Lammerant Citation1973; McCarl et al. Citation1965). The ability to maintain peripheral vasoconstriction has also been correlated with survival during hypoxia in the fetal sheep (Jensen et al. Citation1987).
Whilst some of these factors have been independently investigated in other studies, the relative importance of the factors that may contribute to variability in neurological outcome following global hypoxia in the neonate has not been defined. The aim of this study was to simultaneously monitor a broad range of physiological responses to acute global hypoxia in the neonatal pig in order to assess the variability of responses and to identify the relative influence of each on neurological outcome. Understanding the mechanisms responsible for deleterious responses to hypoxia is a key step in determining and potentially reducing the risk of neural injury.
Materials and methods
Animals
Twenty one-day-old piglets (female n = 9, male n = 11) (Landrace × Large White) from 17 litters were subjected to hypoxia. Control piglets (n = 5) from five separate litters (majority from same litters as the hypoxic piglets) underwent the same procedures as described below with the exception of hypoxia. This study was approved by the Animal Ethics Committee of The University of Queensland and complies with the NHMRC Australian Code of Practice for the Care and Use of Animals for Scientific Purposes.
Surgical preparation
Piglets were initially anaesthetised with 1–2% halothane (Rhône Mérieux, Melbourne) in air. An IV cannula was inserted. To avoid cardiac instability as a result of halothane administration, anaesthesia was maintained with propofol (10 mg/kg/h) (Diprivan, Zeneca, Macclesfield) and alfentanil (55 g/kg/h) (Rapifen, ICI Pharmaceuticals, Melbourne). Piglets were intubated using a cuffed endotracheal tube (size, 3.0 cm) (Mallinchrodt Critical Care, NY) and ventilated using a neonatal ventilator (SLE Newborn 250, UK). Ventilation was commenced at 30 breaths per minute (BPM) and peak pressure was adjusted to maintain paCO2 at 35–45 mmHg. The fraction of inspired oxygen (FiO2) was adjusted to maintain SaO2 at 95–98%. Piglets were warmed using a radiant heater to maintain rectal temperature at 38.5–39.5°C.
An umbilical artery catheter (3.5F) was inserted 10–12 cm to monitor mean arterial pressure (MAP), and heart rate (HR) and for blood sampling. Glucose (10%) was delivered via a mammary vein at 3 ml/kg/h.
Physiological monitoring
Skin blood flow (SBF) and cerebral blood flow (CBF) were measured continuously using laser Doppler flowmetry (LDF) (ADInstruments, PowerLab, Australia). The flat skin flow probe was positioned just above the thigh, after shaving and cleaning the skin. The cerebral probe (1 mm diameter needle) was inserted 0.1 cm into the parasaggittal cortex through a 3 mm burr hole in the skull. Two channels of EEG (Brainz Instruments Limited, New Zealand) were recorded, with one electrode positioned above the eye in the frontal region on each side and the other electrode approximately 3 cm behind this in the occipital region. Cerebral function monitoring (CFM) was obtained from software within the instrument by converting these two channels of EEG to a single channel CFM output.
Blood sampling
Arterial blood gases (Radiometer Pacific, ABL520, Copenhagen, Denmark) and glucose were assessed intermittently before, during and after hypoxia. The serum concentration of cortisol was determined before and at the end of hypoxia using a competitive binding immunoenzymatic assay (ADVIA Centaur, Ireland). The inter-assay variability (CV) for cortisol was 6% at 125 nmol/l, 4.6% at 564 nmol/l and 4.8% at 880 nmol/l; the intra-assay variability was 4.7% at 137 nmol/l, 4.9% at 558 nmol/l and 5.5% at 884 nmol/l. The limit of detection for cortisol was 11 nmol/l. The plasma concentration of adrenaline and noradrenaline was measured using High Performance Liquid Chromatography. The inter-assay variability (CV) for adrenaline was 6% at 1 nmol/l, 4.7% at 10 nmol/l and 5% at 52 nmol/l. The inter-assay variability (CV) for noradrenaline was 9% at 1 nmol/l, 5% at 4 nmol/l and 5% at 20 nmol/l. The assay is linear to 500 nmol/l for adrenaline and noradrenaline, with a limit of detection of 0.1 nmol/l. Serum troponin concentrations were measured before and 3 and 6 h after hypoxia using a chemiluminescence immunoassay (ADVIA Centaur, Ireland). Serum cortisol and troponin concentrations were analysed immediately. Catecholamine samples were stored at − 80°C until analysed.
Hypoxia
Pancuronium (Astra Zeneca, Australia) (0.2 mg/kg) was administered five minutes prior to hypoxia to prevent spontaneous respiration. Hypoxia was induced by reducing the FiO2 to 0.1 and decreasing the ventilation rate from 30 to 10 BPM for 45 min. Hypoxia was terminated by rapidly increasing the FiO2 to 1.0 and returning the ventilation rate to 30 BPM. This resulted in a rapid increase in blood pressure and heart rate. FiO2 was decreased within 15 min to the lowest value consistent with SaO2>94%. If MAP subsequently fell below 30 mmHg dopamine was administered at a rate of 12μg/kg/min to maintain MAP in the normal range and avoid brain injury occurring as a result of post hypoxic hypotension. Six hours after hypoxia piglets were euthanased using Lethobarb (3 ml sodium pentobarbitone 325 mgml− 1 IV) and the brain was removed for immunohistochemical analysis.
Determination of neurological outcome
In order to assess how the physiological responses during hypoxia independently impact on the subsequent neurological outcome a constant insult is required. However, our data (Bjorkman et al. Citation2006) indicate that with an insult of constant degree and duration as few as 20% of animals will provide useful data (i.e. survive and show brain damage) if outcome is assessed at 72 h. While assessment of outcome at 6 h will not allow determination of long term behavioural outcome, it will allow sufficient time for damage to be detectable by the methods described below, without the risk of loss of the most severely affected animals and thus skewing of the data. However assessment at this early time point remains challenging and thus a range of early markers was used in order to provide a broader picture of neurological changes and to increase the reliability of the outcome score.
Cerebral impedance is a functional marker used to monitor the level of cerebral edema. We have shown that cerebral impedance measurements at 4–6 h after hypoxia are strongly correlated with histological outcome assessed at 72 h in the piglet (Lingwood et al. Citation2003). Maximum EEG amplitude was used to monitor the recovery of cerebral activity (Williams et al. Citation1992). We have also shown that early CFM amplitude is well correlated with histological outcome at 72 h in this piglet model (Lingwood et al. Citation2003). Microtubule associated protein-2 (MAP2) immunoreactivity is a structural marker used to assess the integrity of the neuronal cytoskeleton. This is a reliable early marker of neurological outcome in the piglet (Lingwood et al. Citation2008). Although this combined approach will not provide a measure of the exact degree of injury it does provide a measure which is proportional to the final outcome and allows comparison between animals as required for this study.
Cerebral impedance
Cerebral impedance was measured using an SFB2 multifrequency bioelectrical impedance analyzer (SEAC, Brisbane, Australia) via gel electrodes (Fastrace 4, Conmed, NY, USA) placed on the scalp of the piglet (Lingwood et al. Citation2002). Impedance was monitored for 20–30 min at 5 min intervals prior to hypoxia, and at 1 h intervals for 6 h after hypoxia. Impedance at zero frequency (R0) was calculated using software supplied with the instrument. Baseline impedance data were averaged and all subsequent readings were expressed as a percentage of baseline. The range of values seen at 6 h after hypoxia was 70–130%, where 70–90% represents normal function in control piglets and 100–130% represents significant cerebral edema (Lingwood et al. Citation2002). These values were converted to a scale ranging from 0 to 10 (with 10 being the best outcome) using the following formula:
Impedance score = 10 − [(Impedance percent of baseline at 6 h after hypoxia-70)/6]
EEG amplitude
Maximum aEEG amplitude at 6 h was obtained from the CFM record. An EEG amplitude score was calculated out of 10 using the following formula:where EEG hypoxia = CFM maximum amplitude 6h after hypoxia, and EEG control = mean CFM maximum amplitude in the control animals 6 h after the mock insult.
MAP2 immunohistochemistry
The brain was sliced (5 mm thick coronal slices) and placed in fixative (4% paraformaldehyde) within seven minutes of death. After 12 h, paraformaldehyde was replaced with 10% picric acid in phosphate buffered saline (PBS) for 24 h. Tissue was stored in PBS with sodium azide at 4°C. Immunohistochemistry for MAP2 was performed on 7 μm thick paraffin-embedded brain sections. Tissue was de-waxed and rehydrated using a graded ethanol series. Normal goat serum (1%) was used to prevent non-specific labelling. Monoclonal anti MAP2 (ExBio) was applied (dilution 1:10,000) to brain sections and incubated at 4°C overnight. The antigen–antibody complex was visualised using Dako En Vision anti-mouse with 3,3′-diaminobenzidine. On completion of MAP2 staining sections were scanned and analysed using NIH ImageJ. Every pixel in each image was converted to either black or white based on the same threshold, in order to calculate the percent area stained for MAP2. Percent staining from three regions (hippocampus, basal ganglia, and cerebral cortex) were summed to produce the total MAP2 score with a potential maximum of 300. These values were converted to a scale ranging from 0 to 10 using the following formula where zero represents the poorest outcome and 10 represents normal structure.control = mean MAP2 score observed in control piglets.
The derivation of individual scores also took into account that numerical ranges of raw outcome measurements were different (e.g. 0–50 CFM, MAP2 150–350). Conversion of each measure to a score out of 10 prevents the measures with high values influencing the total score more than those with lower values. The derivations also take into account that the direction of change associated with poor outcome is not the same for every measure.
The multiple linear regression analysis used in this study requires a single measure of neurological outcome. Therefore the three measures described above were combined into a single score, which gave equal weighting to structure and function and had a maximum value of 20. This score was normally distributed.
Statistical analysis
Data are expressed as mean ± SD. Mann–Whitney U-test was used to compare changes in physiological function in control and hypoxic piglets. The Wilcoxon matched pair test was used to compare physiological variables at baseline and at the end of hypoxia. Linear regression analysis was used to measure the associations between physiological response/s during hypoxia and the severity of neurological damage and to assess the relative importance of responses. Sensitivity analysis was performed to determine the influence of any outliers on the correlation and thus confirm the reliability of the association. Those factors most strongly associated with outcome were added in a stepwise fashion into a multiple linear regression model. Interactions between independent variables of interest were taken into account after the addition of each covariant into the regression model. Model diagnostics (Studentized residuals) were performed to determine if the model was appropriate for the data. All statistical analyses were performed using Stata for windows (Version 8, Stata Inc, USA) with significance accepted at α = 0.05.
Results
The mean ( ± SD) weight of the 20 piglets that underwent hypoxia was 1.46 ± 0.17 kg. There were 11 males and nine females from a mean litter size of 11.3 ± 3.2 piglets. The baseline values of the physiological variables, along with values during hypoxia are reported in . There were no differences between control and hypoxic groups at baseline with the exception of MAP where the control group was influenced by two piglets that had an unusually low MAP. Seven piglets received dopamine following the hypoxic protocol. There was no difference in the mean neurological outcome scores between those piglets that received dopamine and those that did not receive dopamine (P = 0.97).
Table I. Physiological variables in control (n=5) and hypoxic (n=20) piglets at 10, 30 and 45 min after onset of hypoxia.
There was wide variability in the physiological response to hypoxia. Blood pressure and heart rate increased immediately after the onset of hypoxia in 19 of 20 piglets. Subsequently both variables decreased but the rate of decrease showed substantial variability (). The serum cortisol, adrenaline and noradrenaline concentrations were all significantly greater in the hypoxic group than in the control group (). The magnitude of the increases in these hormone concentrations was highly variable as indicated by the large standard deviation for values measured at the end of hypoxia. Serum troponin concentration was undetectable in the control piglets and at baseline in the hypoxic piglets. Values were 2.6 ± 2.2 μg/l at 3 h and 1.9 ± μg/l at 6 h post hypoxia. These physiological changes were not correlated with weight, age, gender, litter size, baseline physiology, duration or dose rate of anaesthetic, depth of anaesthesia as indicated by CFM amplitude or blood gas parameters before or during hypoxia. For all physiological variables the value at the end of hypoxia was independent of the baseline value.
Figure 1 Representative physiological responses during hypoxia and their subsequent neurological outcome score in three individual piglets. The upper panel shows the response of a piglet that maintained cardiovascular parameters above baseline for most or all of the hypoxic period. The middle panel shows the response of a piglet that did not maintain cardiovascular parameters above baseline as long as the first piglet. The lower panel shows the response of a piglet where cardiovascular parameters fell below baseline soon after the onset of hypoxia. Note that cerebral perfusion closely follows the changes in cardiovascular function in all piglets. Vertical dashed line indicates end of hypoxic period. Solid line is mean arterial pressure (MAP); dashed line is heart rate (HR); gray line is cerebral blood flow (CBF); dotted line is skin blood flow (SBF). All values are plotted as % baseline values. Outcome score is derived from cerebral impedance, EEG (CFM) and microtubule associated protein-2 (MAP2) measurements.
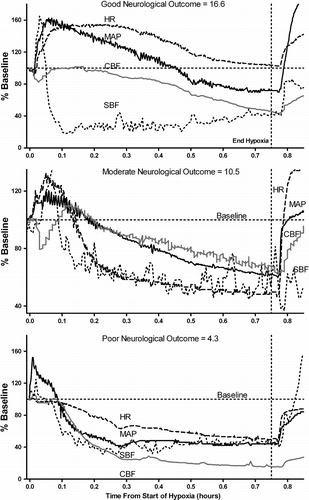
Both the individual components of the neurological outcome score () and the combined neurological outcome in the hypoxic group showed substantial variability with the combined outcome scores ranging from 3.8 to 17.8, compared to 16.9–18.4 for the control group (). This variability was not associated with age, weight, gender, litter size, administration of dopamine, duration or dose rate of anaesthesia prior to hypoxia, or depth of anaesthesia as indicated by CFM amplitude prior to hypoxia. No piglets died during the hypoxic insult.
Figure 2 Representative changes in individual components of the neurological outcome score. Example of the changes in cerebral impedance, CFM and total MAP2 score for individual piglets representing control experiment (no hypoxia), good, moderate and poor neurological outcome at 6 h. Solid line is CFM amplitude; broken line is cerebral impedance.
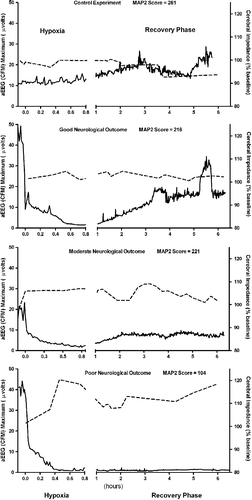
Figure 3 Neurological outcome scores in hypoxic and control animals showing scores in individual animals with means for the hypoxic (n = 20) and control (n = 5) animals.
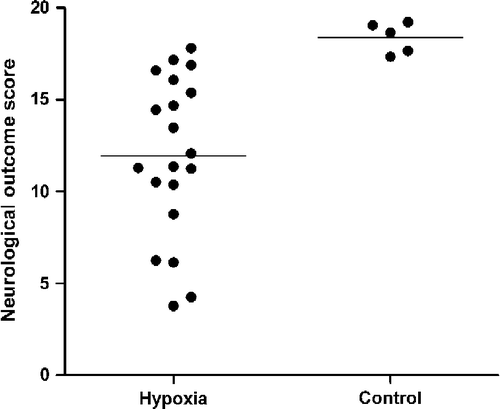
shows the results of the simple linear regression analysis for the physiological variables and neurological outcome. The duration of the time for which HR was below baseline, the serum cortisol concentrations at the end of hypoxia, and duration of hypotension (the time for which MAP was below 70% of baseline) were the most strongly correlated with neurological outcome. When these variables were entered stepwise into a multiple linear regression analysis (), only two factors (the time HR was below baseline and the cortisol concentrations) contributed significantly to the model. These two factors explain 68% of the variance in neurological outcome. The addition of further factors did not significantly strengthen the association.
Table II. Linear regression between physiological variables and neurological outcome (n=20).
Table III. Stepwise multiple regression for physiological variables that are significant determinants of neurological outcome.
Linear regression was also used to assess the relationship between physiological variables. shows the associations between the serum concentration of cortisol at the end of hypoxia with the time for which HR was below baseline and the duration of hypotension. A significant correlation was also observed between the time for which CBF was below baseline and the duration of hypotension (R2 = 0.32, P = 0.011).
Discussion
In this study we observed high variability in both neurological outcome and physiological responses to global hypoxia in the newborn piglet. This is the first study to simultaneously consider the relationships between a large number of physiological variables and neurological outcome following hypoxia and assesses the relative importance of these variables. The variables most strongly associated with outcome were the time for which HR was below baseline and the serum cortisol concentrations at the end of hypoxia: together these variables accounted for 68% of the variability in neurological outcome.
Previous studies have shown that neurological outcome is affected by cardiovascular function during hypoxia (de Courten-Myers et al. Citation1989; Gunn et al. Citation1992; Mallard et al. Citation1992; Thoresen et al. Citation1996; Bender et al. Citation2003). The current study supports these findings but shows that serum cortisol concentrations at the end of hypoxia are an almost equally important predictor of neurological outcome (r = − 0.77 for time HR is below baseline and 0.70 for serum cortisol concentrations). The inability of some piglets to maintain cardiovascular function during hypoxia may be due to low cardiac glycogen reserves, immaturity of the sympathetic nervous system or HPA axis. Further research is required to assess the importance of these factors in determining neurological outcome.
The cortisol concentrations reported in the current study were highly variable but within the same range as those reported before and during hypoxia in the near term anaesthetized fetal sheep (cortisol values ranging from 80–483 nmol/l) (Fletcher et al. Citation2006; Roelfsema et al. 2005). It is unclear why some animals are unable to produce as much cortisol during hypoxia as others and this area warrants further investigation. It is also unclear from the current study whether the beneficial association of cortisol with neurological outcome is a direct effect, or an indirect effect through cortisol actions on cardiovascular function. Cortisol has positive effects on the cardiovascular system such as enhancing the sensitivity of the myocardial endothelial cells to catecholamines (Giussani et al. Citation1994), prolonging the beating of heart cells in vitro (McCarl et al. Citation1965), and promoting vasoconstriction (Yang and Zhang Citation2004), while higher cortisol concentrations have been shown to be associated with better maintenance of cardiovascular function in the fetal sheep (Fletcher et al. Citation2006). Alternatively, better maintenance of cardiovascular function may be the result of sympathetic nervous system activity, which simultaneously increases cortisol secretion (Edwards and Jones Citation1987a, Citationb; Giussani et al. Citation1994). In contrast, a number of studies in adult rat stroke models have suggested cortisol may have detrimental effects on the brain during hypoxia–ischemia possibly by increasing glutamate excitotoxicity (Krugers et al. Citation1998; Payne et al. Citation2003; Smith-Swintosky et al. Citation1996). It is unclear what direct effects cortisol may have on the hypoxic neonatal brain and, if present, whether these may be outweighed by the beneficial effects on the cardiovascular system.
A number of physiological responses were not associated with neurological outcome. Despite the evidence that the sympathetic nervous system plays a critical role in cardiovascular function (Jones et al. Citation1988; Lagercrantz and Bistoletti Citation1973; Jensen Citation1991), the addition of serum catecholamine concentrations to the multiple regression model did not significantly improve the association with outcome. One possible explanation for the lack of significant associations observed may be because catecholamines have a very short half life in the circulation or are rapidly removed from the synaptic cleft (Esler et al. Citation1990), thus catecholamine concentrations in blood may not be a good measure of tissue exposure or activity of the sympathetic nervous system. Further research should focus on sympathetic nerve recordings and/or spillover studies in order to assess the importance of this system, its response to hypoxia and relationship to cardiovascular function and neurological outcome in the newborn piglet.
In the clinical environment where the duration of hypoxia is not constant, pH will be more variable and provide some indication of the severity of hypoxia. Our previous data demonstrated a significant association between pH at the end of hypoxia and neurological outcome when the severity of hypoxia is not constant (Lingwood et al. Citation2003). The current study found that arterial pH and base excess were not associated with neurological outcome. However, with the constant insult we applied in this study, there was minimal variability in pH at each time point. Despite the consistency of pH levels, we still observed considerable variability in neurological outcome. This supports the view that the physiological response to hypoxia is a more important determinant of neurological outcome than biochemical changes during hypoxia such as pH and base excess. This may account for the numerous studies which have reported that the severity of acidosis is a poor predictor of long term neurological outcome following asphyxia (Lavrijsen et al. Citation2005; Dennis et al. Citation1989).
Surprisingly, this study did not show a strong association between cortical cerebral blood flow and neurological outcome. It has been previously reported that brain injury is highly associated with reduced cerebral blood flow during hypoxia (Bender et al. Citation2003; de Courten-Myers et al. Citation1989). The observed relationship between cardiovascular function and outcome in this study is most likely due to the failure of autoregulation during hypoxia (Tweed et al. Citation1983), and thus the dependence of cerebral blood flow during hypoxia on cardiovascular function (Jensen Citation1996; Hohimer et al. Citation1991; Tweed et al. Citation1983; Lou et al. Citation1979). In our study the time for which CBF was below baseline was significantly correlated with the duration of hypotension. Laser Doppler flowmetry measures flow in only a small tissue volume ( < 1 mm3). Increases in cell volume due to cerebral edema and brain swelling may alter the amount of tissue being monitored and account for the lack of significant association between overall CBF and neurological outcome. Although it has been previously reported that there is no significant histopathological damage associated with implantation of the Doppler probe (Lan et al. Citation2000) the possibility that local damage has confounded results of the current study cannot be discounted.
A limitation of this study was the use of anaesthesia in this model of perinatal asphyxia. While anesthesia could potentially affect cerebral blood flow and neurological outcome, the anesthetic regime was the same for each piglet and thus these effects were consistent across all piglets. The duration, dose rate or depth of anaesthesia prior to hypoxia was not related to neurological outcome or physiological responses. Another potential limitation is the early assessment of neurological outcome. While cerebral impedance and CFM have been validated against histology at 72 h and reliably indicate the longer term outcome, it is not possible to determine the exact relationship between MAP2 immunoreactivity at 6 h and later histology. We have shown that there is a consistent relationship between MAP2 level at 6 h and other indicators of long term outcome (Lingwood et al. Citation2008). Thus, MAP2 immunoreactivity will allow comparison between animals, indicating the animals that will be most or least severely damaged, which is all that was required by this study. The findings of this study may not be applicable to different hypoxic insults (e.g. more rapid and severe insults). The major strength of this study was the measurement of multiple objective physiological responses during hypoxia and assessment of the relative importance of each of these responses in terms of neurological outcome. The quantification of how much of the variability in neurological outcome is associated with physiological response is of great importance in determining the factors that contribute to brain injury.
Conclusion
This study in piglets suggests that some individuals are more tolerant of neonatal hypoxia than others. There was significant variability in neurological outcome, which was largely explained by variability in both the cardiovascular responses and serum cortisol concentrations. The variability in response may be more important than previously recognised and may potentially explain why factors related to the severity of the insult, such as pH, are poor predictors of outcome. The identification by this study of major determinants of neurological outcome may make it possible to exploit naturally occurring mechanisms of tolerance to hypoxia in order to improve the physiological responses and subsequent neurological outcome. Our study indicates that the most effective strategies to improve the outcome may be based on modification of the cardiovascular and adrenocortical responses to hypoxia.
Declaration of interest: The authors report no conflicts of interest. The authors alone are responsible for the content and writing of the paper.
Notes
Research funded by University of Queensland Research and Development Fund.
References
- Barks JD, Post M, Tuor UI. Dexamethasone prevents hypoxic-ischemic brain damage in the neonatal rat. Pediatr Res 1991; 29: 558–563
- Bender TM, Johnston JA, Manepalli AN, Mink RB. Association between brain tissue pH and brain injury during asphyxia in piglets. Resuscitation 2003; 59: 243–254
- Bjorkman ST, Foster KA, O'Driscoll SM, Healy GN, Lingwood BE, Burke C, Colditz PB. Hypoxic/ischemic models in newborn piglet: comparison of constant FiO2 versus variable FiO2 delivery. Brain Res 2006; 1100: 110–117
- Borke WB, Munkeby BH, Morkrid L, Thaulow E, Saugstad OD. Resuscitation with 100% O(2) does not protect the myocardium in hypoxic newborn piglets. Arch Dis Child 2004; 89: F156–F160
- de Courten-Myers GM, Fogelson HM, Kleinholz M, Myers RE. Hypoxic brain and heart injury thresholds in piglets. Biomed Biochim Acta 1989; 48: S143–S148
- Dennis J, Johnson A, Mutch L, Yudkin P, Johnson P. Acid–base status at birth and neurodevelopmental outcome at four and one-half years. Am J Obstet Gynecol 1989; 161: 213–220
- Downing SE, Gardner TH, Rocamora JM. Adrenergic support of cardiac function during hypoxia in the newborn lamb. Am J Physiol 1969; 217: 728–735
- Edwards AV, Jones CT. The effect of splanchnic nerve section on the sensitivity of the adrenal cortex to adrenocorticotrophin in the calf. J Physiol 1987a; 390: 23–31
- Edwards AV, Jones CT. The effect of splanchnic nerve stimulation on adrenocortical activity in conscious calves. J Physiol 1987b; 382: 385–396
- Esler M, Jennings G, Lambert G, Meredith I, Horne M, Eisenhofer G. Overflow of catecholamine neurotransmitters to the circulation: source, fate, and functions. Physiol rev 1990; 70: 963–985
- Feet BA, Brun NC, Hellstrom-Westas L, Svenningsen NW, Greisen G, Saugstad OD. Early cerebral metabolic and electrophysiological recovery during controlled hypoxemic resuscitation in piglets. J Appl Physiol 1998; 84: 1208–1216
- Fletcher AJ, Gardner DS, Edwards CM, Fowden AL, Giussani DA. Development of the ovine fetal cardiovascular defense to hypoxemia towards full term. Am J Physiol Heart Circ Physiol 2006; 291: H3023–H3034
- Giussani DA, McGarrigle HH, Moore PJ, Bennet L, Spencer JA, Hanson MA, et al. Carotid sinus nerve section and the increase in plasma cortisol during acute hypoxia in fetal sheep. J Physiol 1994; 477(Pt 1)75–80
- Gunn AJ, Parer JT, Mallard EC, Williams CE, Gluckman PD. Cerebral histologic and electrocorticographic changes after asphyxia in fetal sheep. Pediatr Res 1992; 31: 486–491
- Hohimer AR, Chao CR, Bissonnette JM. The effect of combined hypoxemia and cephalic hypotension on fetal cerebral blood flow and metabolism. J Cereb Blood Flow Metab 1991; 11: 99–105
- Jensen A. The role of the sympathetic nervous system in preventing perinatal brain damage. In Oxygen, basis of the regulation of vital functions in the fetus, W Kunzel. Springer Verlag, New York, NY 1991; 77–107
- Jensen A. The brain of the asphyxiated fetus—basic research. Eur J Obstet, Gynecol Reprod Biolgy 1996; 65: 19–24
- Jensen A, Hohmann M, Kunzel W. Dynamic changes in organ blood flow and oxygen consumption during acute asphyxia in fetal sheep. J Dev Phys 1987; 9: 543–559
- Jones CT, Roebuck MM, Walker DW, Johnston BM. The role of the adrenal medulla and peripheral sympathetic nerves in the physiological responses of the fetal sheep to hypoxia. J Dev Physiol 1988; 10: 17–36
- Kolanowski J, Lammerant J. Rate of uptake of endogenous cortisol by the left ventricle of the anaesthetized dog during ventilation with ambient air and during hypoxia. J Physiol 1973; 233: 519–527
- Krugers HJ, Kemper RH, Korf J, Ter Horst GJ, Knollema S. Metyrapone reduces rat brain damage and seizures after hypoxia-ischemia: an effect independent of modulation of plasma corticosterone levels?. J Cereb Blood Flow Metab 1998; 18: 386–390
- Lagercrantz H, Bistoletti P. Catecholamine release in the newborn infant at birth. Pediatr Res 1973; 11: 889–893
- Lan J, Hunter CJ, Murata T, Power GG. Adaptation of laser-Doppler flowmetry to measure cerebral blood flow in the fetal sheep. J Appl Physiol 2000; 89: 1065–1071
- Lavrijsen SW, Uiterwaal CS, Stigter RH, de Vries LS, Visser GH, Groenendaal F. Severe umbilical cord acidemia and neurological outcome in preterm and full-term neonates. Biol Neonate 2005; 88: 27–34
- Ley D, Oskarsson G, Bellander M, et al. Different responses of myocardial and cerebral blood flow to cord occlusion in exteriorized fetal sheep. Pediatr Res 2004; 55: 568–575
- Lingwood BE, Dunster KR, Colditz PB, Ward LC. Noninvasive measurement of cerebral bioimpedance for detection of cerebral edema in the neonatal piglet. Brain Res 2002; 945: 97–105
- Lingwood BE, Dunster KR, Healy GN, Ward LC, Colditz PB. Cerebral impedance and neurological outcome following a mild or severe hypoxic/ischemic episode in neonatal piglets. Brain Res 2003; 969: 160–167
- Lingwood B, Healy G, Sullivan S, Pow D, Colditz P. MAP2 provides reliable early assessment of neural injury in the newborn piglet model of birth asphyxia. J Neurosci Methods 2008; 171: 141–146
- Liu Y, Wada H, Takada S, Uetani Y, Itoh H, Nakamura H. Preventive effects of dexamethasone on hypoxic-ischemic brain damage in the neonatal rat. Brain Dev 1995; 17: 186–192
- Lou HC, Lassen NA, Tweed WA, Johnson G, Jones M, Palahniuk RJ. Pressure passive cerebral blood flow and breakdown of the blood–brain barrier in experimental fetal asphyxia. Acta Paediatr Scand 1979; 68: 57–63
- Low JA. Determining the contribution of asphyxia to brain damage in the neonate. J Obstet Gynaecol Res 2004; 30: 276–286
- Mallard EC, Gunn AJ, Williams CE, Johnston BM, Gluckman PD. Transient umbilical cord occlusion causes hippocampal damage in the fetal sheep. Am J Obstet Gynecol 1992; 167: 1423–1430
- Martinez AM, Padbury JF, Humme JA, Evans CW, Shames L. Plasma catecholamines and their physiologic thresholds during the first ten days of life in sheep. J Dev Physiol 1990; 13: 141–146
- McCarl RL, Szuhaj BF, Houlihan RT. Steroid stimulation of beating of cultured rat-heart cells. Science 1965; 150: 1611–1613
- Payne RS, Tseng MT, Schurr A. The glucose paradox of cerebral ischemia: evidence for corticosterone involvement. Brain Res 2003; 971: 9–17
- Seidler FJ, Slotkin TA. Adrenomedullary function in the neonatal rat: responses to acute hypoxia. J Physiol 1985; 358: 1–16
- Shankaran S, Woldt E, Koepke T, Bedard MP, Nandyal R. Acute neonatal morbidity and long-term central nervous system sequelae of perinatal asphyxia in term infants. Early Hum Dev 1991; 25: 135–148
- Smith-Swintosky VL, Pettigrew LC, Sapolsky RM, Phares C, Craddock SD, Brooke SM, Mattson MP. Metyrapone, an inhibitor of glucocorticoid production, reduces brain injury induced by focal and global ischemia and seizures. J Cereb Blood Flow Metab 1996; 16: 585–598
- Thoresen M, Haaland K, Loberg EM, Whitelaw A, Apricena F, Hanko E, Steen PA. A piglet survival model of posthypoxic encephalopathy. Pediatr Res 1996; 40: 738–748
- Tweed WA, Cote J, Pash M, Lou H. Arterial oxygenation determines autoregulation of cerebral blood flow in the fetal lamb. Pediatr Res 1983; 17: 246–249
- Vannucci RC, Perlman JM. Interventions for perinatal hypoxic-ischemic encephalopathy. Pediatrics 1997; 100: 1004–1014
- Williams CE, Gunn AJ, Mallard C, Gluckman PD. Outcome after ischemia in the developing sheep brain: an electroencephalographic and histological study. Ann Neurol 1992; 31: 14–21
- Williams CE, Mallard C, Tan W, Gluckman PD. Pathophysiology of perinatal asphyxia. Clin Perinatol 1993; 20: 305–325
- Yang S, Zhang L. Glucocorticoids and vascular reactivity. Curr Vasc Pharmacol 2004; 2: 1–12