1. Introduction
Among all the unsteady phenomena present at three-dimensional flows, the Kramer effect has, to our knowledge, never been demonstrated in swimming. Yet this phenomenon plays an important role in the flow generated by the rotating movements of wings, whether in the air or in water. It is for example very present in the animal flapping flight (hummingbird for example) or for the Micro Air Vehicle (MAV). More specifically, this effect occurs during the rotations of the wings (pronation or supination phases) between the translation phases. During these rotating movements, the flow around the profile then tends to deviate from the Kutta condition by the presence of a stopping point upstream of the trailing edge. In order to restore this Kutta condition, additional circulation is generated around the profile, acting favorably (or adversely) on force generation according to the characteristics of the rotation (Bennett Citation1970; Dickinson et al. Citation1999). This effect was experimentally demonstrated by Kramer (Citation1932). In swimming, such rotational movements exist, especially in synchronized swimming (called “sculling” movement), but also in competitive swimming, between the different phases of insweep, upsweep or outsweep (times where the hand goes through the outermost points of the aquatic stroke; Samson et al. Citation2015). Thus, the purpose of this study is to analyse whether the angular rotations of the hands in swimming (pronation and/or supination), during the transition phases between insweep and upsweep, have an effect on the hydrodynamic forces.
2. Methods
The study was performed in numerical simulation. The flow was simulated using a URANS method (Samson et al. Citation2017). The k-ω SST model closed the flow equations and a moving mesh was used, in free surface condition. The finite volume method is applied on a hybrid mesh made of structured mesh (near-wall) and unstructured mesh. Kinematic parameters were imported into the solver via a data file, which the time step is equal to the physical time-step (2.5.10−3 s). The independence of the calculated physics based on the numerical conditions was tested with respect to the computational time-step, the number of iterations, and the domain and mesh size. Kinematic data were measured in the context of swimming from an expert swimmer. A Vicon® optoelectronic motion analysis system was used (Monnet et al. Citation2014). The movement consisted in an aquatic stroke of a hand-forearm segment in front crawl swimming, in sprint (Samson et al. Citation2015). During this movement, there are two pronation phases for this swimmer between each sweep phase (). In order to study the role of these phases of rotation of the hand, three simulations were carried out:
Figure 1. (a) norm of translational velocity of the hand in the global reference (blue curve) and rotational speed of the hand (red curve). (b) pronation, supination of the hand; (c) top view of three hand aquatic strokes: case 1: with DC-IN and IN-UP pronation; case 2, without DC-IN pronation, case 3: without IN-UP pronation.
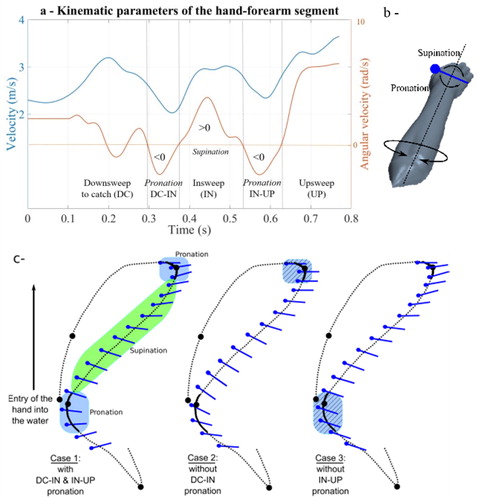
Case 1: path with both pronation DC-IN and IN-UP (real kinematic data, measured from an expert swimmer).
Case 2: modified path, without pronation of the hand during the DC-IN transition DC-IN.
Case 3: modified path, without pronation of the hand during the DC-IN transition IN-UP.
Comparison between these three simulations was made from the total forces produced by the forearm and the hand in the absolute reference (0, x, y, z).
3. Results and discussion
Case 1 (solid curve): shows, at t = 0.32 s and t = 0.35 s (Pronation DC-IN phase), two peaks of force to a value of about 100 N. During the pronation IN-UP phase, there is a peak force (55 N at t = 0.58 s).
Figure 2. Hydrodynamic forces acting on the hand and forearm during an aquatic stroke in front crawl swimming.
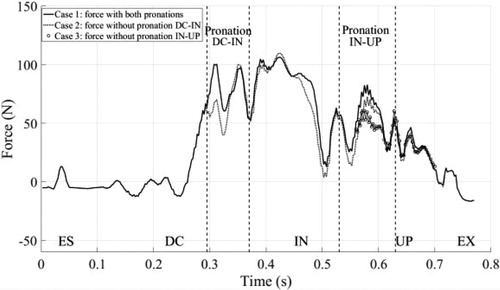
Case 2 (dashed curve): shows that the forces acting on the hand and forearm are less important when the hand is not pronating. The absence of pronation acts directly on the forces produced during this transition, and in particular the first peak of force from t = 0.28 s to t = 0.32 s. Beyond this, we find that the absence of pronation also acts on the propulsive forces on the following phases, where the hydrodynamic force is lower in case 2 than in case 1.
Case 3 (curve with circles): It can be seen in that the absence of pronation during the IN-UP transition (between t = 0.53 s and t = 0.63 s) decreases the hydrodynamic forces acting on the hand-forearm segment. This lack of pronation acts more on the hydrodynamic forces created during the next phase (upsweep). This decrease in strength can be further explained by the decrease in the angle of attack (due to the absence of pronation) during the insweep phase.
Thus, it appears from the results obtained in the three different cases that the DC-IN and IN-UP pronation phases generate more propulsive force than when there are no pronation. Based of Bennett (Citation1970) and Dickinson et al. (Citation1999), we can then hypothesize that these force peaks were generated from additional circulation created around the hand and forearm. Besides the additional generation of circulation, impulse incidence change tends to delay the “dynamic stall” effect when rotation increases the angle of attack (Bennett Citation1970). Here the pronation of the hand, besides the fact that it prepares the right orientation for the next phase, could then create an additional circulation which, if one relies on these studies, would explain the peaks of force created at t = 0.35 s and t = 0.58 s.
4. Conclusions
It has been shown that the hand pronations performed during the transitions between the translation phases contribute to increase the propulsive forces. This study has highlighted the role of the Kramer effect in the generation of unsteady swimming forces. In addition, this pronation promotes the orientation of the hand in the direction of advancement, but also prepares the orientation of the hand for the next phase by contributing to the maintenance of high angles of attack. These results then tend to value the technique in “S”, with external and internal sweep, rather than an “I” path in the axis. This hypothesis needs to be verified more by different path configurations, especially on sculling type paths (breaststroke, butterfly, synchronized swimming).
References
- Bennett L. 1970. Insect flight: lift and rate of change of incidence. Science. 167(3915):177–179.
- Dickinson MH, Lehmann FO, Sane SP. 1999. Wing rotation and the aerodynamic basis of insect flight. Science. 284(5422):1954–1960.
- Kramer M. 1932. Die zunahme des maximalauftriebes von tragflugeln bei plotzlicher anstellwinkelvergrosserung (boeneffekt). Zeit Flugtech Motorluftsch. 23:185–189.
- Monnet T, Samson M, Bernard A, David L, Lacouture P. 2014. Measurement of three dimensional hand kinematics during swimming with a motion capture system: a feasibility study. Sports Eng. 17(3):171–181.
- Samson M, Monnet T, Bernard A, Lacouture P, David L. 2015. Kinematic hand parameters in front crawl at different paces of swimming. J Biomech. 48(14):3743–3750.
- Samson M, Monnet T, Bernard A, Lacouture P, David L. 2017. Unsteady computational fluid dynamics in front crawl swimming. Comput Methods Biomech Biomed Engin. 20(7):783–793.