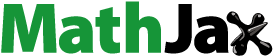
ABSTRACT
Waste tire rubber has been incorporated into asphalt modification for decades due to its various benefits. There are two main mechanisms during bitumen–rubber interaction: rubber swelling and chemical degradation. This study surveys these two processes from the viewpoint of polymer science. The kinetics of rubber dissolution and thermodynamics of rubber swelling are discussed to provide a fundamental understanding of the interaction process and to demonstrate how optimisation of material selection and processing procedures can lead to the desired binder properties. Factors including the interaction conditions and raw material characteristics are analysed based on the previous theories and compared with experimental results.
1. Introduction
With the developments in transportation and the associated increase in numbers of vehicles, approximately one billion end-of-life tires (ELTs) are produced every year worldwide (WBCSD Citation2010). Due to the greater awareness of environmental issues and potential economic benefits, engineers are attempting to develop a more sustainable framework to dispose the ELTs (Sienkiewicz et al. Citation2012). Locally, it was estimated that the European Union (EU) produced 3.6 million tonnes of ELTs in 2013. An estimated 2.7 million tonnes of them were recovered and recycled, which represents a treatment rate of 96% and confirms the world-leading role of the EU in the waste tire management (Etrma Citation2016). Material recycling is the most common means of managing ELTs in the EU and has been gaining more and more attention due to the lower processing costs and additional benefits (Sienkiewicz et al. Citation2012, Torretta et al. Citation2015). In the paving industry, waste tire rubber has been successfully utilised as a modifier to improve the properties of binders (Lo Presti Citation2013).
Crumb rubber is recycled rubber with a granular consistency produced from ELTs through removing the steel and tire cord (fluff) and mechanical grinding. Crumb rubber modifier (CRM), whose size is usually ranging from 0.075 to 4.75 mm, is the common name used to identify the rubber particles ground from ELTs for bitumen modification (State of California Department of Transportation Citation2003). There are two primary methods of incorporating CRM into hot mix asphalt (HMA), which are generally referred to as dry process and wet process. In the dry process, CRM is mixed directly with the aggregate prior to introducing the required binder during the HMA production. The CRM acts as a partial replacement of stone aggregates in asphalt mixtures. Through the wet process, CRM is blended with bitumen and a predetermined reaction time is required before mixing the modified binder with aggregates. According to the different wet processing technologies (State of California Department of Transportation Citation2003, Lo Presti Citation2013, Shu and Huang Citation2014), rubberised asphalt has various technical terminologies, such as Asphalt Rubber (AR), Terminal Blends (TB), Crumb Rubber Modified Binder (CRMB), etc. Specifically, AR is defined as a blend of bitumen, recycled tire rubber and certain additives in which the minimum rubber content is 15% by weight of the total blend. The blend is reacted at high temperatures until sufficient swelling of rubber particles is achieved. TB is actually a preparation technique where finely ground CRMs are blended with hot bitumen at the refinery or the binder storage and distribution terminal. CRMB is a general term to identify any bituminous binder modified by CRM. Rubberised asphalt concrete has been successfully applied in paving industries for decades thanks to the development of paving technologies (Lo Presti Citation2013). The incorporation of CRM into HMA improves the overall pavement performance and yield also intangible benefits (Wang et al. Citation2018a). Most of these improvements are attributed to the interaction of crumb rubber and bitumen which leads to changes in binder composition and microstructure (Gawel et al. Citation2006, Attia and Abdelrahman Citation2009, Ghavibazoo and Abdelrahman Citation2013).
Previous researchers have done extensive literature reviews (Lo Presti Citation2013, Shu and Huang Citation2014, Wang et al. Citation2017a, Citation2017b, Citation2018a) of rubberised asphalt regarding the production technology, mix design methodologies, performance characterisation and specifications, storage and transport stability, construction technologies, environmental and economic impact assessment, etc. However, most of the conclusions drawn in the previous studies were based on laboratory tests and lack of fundamental explanations, which may limit their further applications. This paper focuses on a less discussed but crucial topic, namely, the interaction mechanisms between bitumen and rubber at different conditions from the viewpoint of polymer science. It is an attempt to establish a theoretical framework for the rubber–bitumen interaction process as a tool to optimise the binder properties.
presents the framework of this review article. After the introduction, the chemistry of bitumen and rubber, which is most related to the interaction, is introduced. Then, the bitumen–rubber interaction is described from both phenomenal and theoretical viewpoints. The kinetic and thermodynamics aspects of the interaction will be discussed in the theoretical part. In the following section, critical factors in practice are analysed with reflections from the theories in an attempt to binder property optimisation. Conclusions and recommendations based on previous analyses are summarised in the last section.
2. Chemistry of raw materials: bitumen and crumb rubber
2.1. Composition of bitumen
Bitumen is not a polymeric material but a complex mixture consisting of hydrocarbons of different size, polarity and aromaticity. It may also contain small amounts of heteroatoms, such as sulphur (0–9 wt.%), nitrogen (0–2 wt.%), oxygen (0–2 wt.%) and traces of metals (e.g. vanadium and nickel). The number-average molecular weight of bitumen generally ranges from 600 to 1500 Da and therefore, bitumen molecules cannot be considered as macromolecules in the polymeric sense. Given the highly complex molecular structure of bitumen, it is almost impossible to conduct detailed chemical analysis. Even though bitumen consists of a continuum of similar molecules, to facilitate classification and the understanding of its properties based on dominant molecular group types, bitumen molecules are generally classified into different molecular groups depending on their size and solubility in polar, aromatic or non-polar solvents. The widely accepted SARA (saturates, aromatics, resins and asphaltenes) fractions, developed based on the selective adsorption–desorption (chromatographic) method (Lesueur Citation2009), are used to give the relative quantities of these components in bitumen. The physicochemical properties of bitumen and the SARA fractions are compiled in . Bitumen can be described as a colloidal dispersion of high-molecular-weight asphaltene micelles in the low-molecular-weight maltenes (saturates, aromatics, resins) (Lesueur Citation2009). The solubility model is also often used to describe the chemistry of bitumen where the asphaltenes are dissolved in the maltenes rather than dispersed (Redelius Citation2004). The key aspect of solubility model is that there is no gap in chemical properties between the fractions of bitumen which are a continuous range of molecules with respect to size, polarity and aromaticity (Redelius and Soenen Citation2015). The colloidal structure and solubility parameter of bitumen influences its rheology and interactions with different polymers.
Table 1. Physiochemical properties of bitumen and the SARA fractions (Daly Citation2017, Lesueur Citation2009).
2.2. Compositions of tire rubber
As mentioned before, the crumb rubber used for bitumen modification is recycled from waste tires. The composition of tires is very complex to fulfil their end-use properties. summarises the detailed tire ingredients used in different vehicles in the EU. Normally, during the handling and shredding processes, the reinforcing wires and fibres are removed to produce a clean and highly consistent rubber material for bitumen modification. In general, truck and off-the-road (OTR) tires contain higher proportions of natural rubber (NR) than passenger car tires. NR and synthetic rubber (SR) have different interactions with bitumen at the same conditions. This explains why the tire source can have considerable influence on the properties of CRMB binders (Frantzis Citation2004, Artamendi and Khalid Citation2006). NR is industrially obtained from the latex of the tree called Hevea brasiliensis and it is essentially a hydrocarbon polymer. The main constituent of NR is the polymer cis-1,4-polyisoprene with a molecular weight of 105–106 Da. As can be seen in (a), macromolecules of NR are long, regular, flexible and practically linear. In contrast, SR is any artificial rubber, synthesised from petroleum by-products through a polymerisation process. The most common SR used in tire manufacturing is styrene–butadiene rubber (SBR) shown in (b) derived from the copolymerisation of styrene (C8H8) and butadiene (C4H6). The properties of SBR are influenced not only by the micro- and macrostructure of polymer chains, but also the styrene/butadiene ratio. NR and SBR polymers used in tire manufacturing possess similar glass transition temperatures of approximately −70°C (Burfield and Lim Citation1983). During the polymer production, polymers are usually heated well above the glass transition temperature to allow casting, moulding and extrusion into desired forms. Considering the typical tire moulding temperature is ∼177°C in the manufacturing process (Mark et al. Citation2013), it is not uncommon to see the interaction temperature between rubber and bitumen range from 180 to 220°C. In this temperature range, the polymer chains of rubber change from rigid glassy regions to flowable melt regions. Therefore, rubber particles can be incorporated into the bitumen network establishing the inverse continuous phase due to the high chain mobility.
Figure 2. Chemical structure of (a) cis-polyisoprene, (b) copolymer SBR and (c) crosslinking after vulcanisation, adapted from Mark (Citation2009).
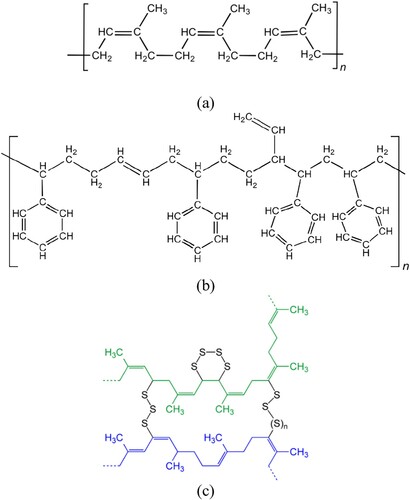
Table 2. Composition comparison of different tires in the EU, adapted from Etrma (Citation2016).
To improve the durability and mechanical properties of rubber, vulcanisation is applied to most rubber polymers by forming chemical crosslinks between individual polymer chains to create three-dimensional networks (Mark et al. Citation2013). The most commonly used curative during the vulcanisation process is sulphur. (c) shows the formation of crosslink between two strands (blue and green) of NR after vulcanisation with elemental sulphur (Mark Citation2009). Vulcanisation can effectively increase the retractive force and reduce the amount of permanent deformation remaining after removal of the force. During the tire rubber manufacturing process, various additives and ancillary substances necessary for vulcanisation are added to improve the overall physical properties. Carbon black and amorphous silica are added into rubber as reinforcing fillers, which can significantly improve the anti-abrasion property and the material strength and hardness. Other additives, including activator accelerator (zinc oxide), coagulants, anti-oxidants, colour pigments, surfactants, softeners (oils), etc., are added in the tire manufacturing process to improve the tire properties and workability. Thermogravimetric analysis (TGA) is a useful and reliable tool for material composition analysis (Cui et al. Citation1999). presents a typical TGA curve of a tire rubber sample. It provides quantitative and/or qualitative information about the main component and their concentrations in the sample based on the decomposition temperature and mass loss (Cui et al. Citation1999). As shown in , the main components of tire rubber include oily and volatile substances, NR and SR, inorganic fillers, carbon black and residual ash. This implies that TGA could be used for monitoring the change of rubber during the bitumen–rubber interaction process (Ghavibazoo and Abdelrahman Citation2013).
3. Bitumen–rubber system and interaction process
Typically, CRMB is produced by mixing bitumen and rubber at elevated temperature for a certain period. The bitumen–rubber interaction controls the property development of CRMB and has a great influence on the binder transport and storage. There are two main stages which occur during the interaction process: rubber swelling and degradation (Abdelrahman and Carpenter Citation1999, Wang et al. Citation2017a). Rubber swelling is a physical diffusion process while the degradation of rubber is a chemical process in which new substances are created. It is often seen in the literature that researchers use the terminology of rubber dissolution to describe the status of rubber particles in bitumen during the interaction. Rubber dissolution is measured by extracting the rubber particles from the binder matrix. The portion of rubber particles in CRMB that passes through a certain fine mesh (usually 75 µm), which is usually difficult recognised by eyes, is considered as the dissolved portion (Ghavibazoo et al. Citation2013b). From the definition, it can be seen that dissolution here is a vague engineering term which cannot distinguish if chemical degradation of rubber occurs or not. The dissolved portion may contain extremely fine rubber particles that are disassociated/split from coarse rubber particles. The percentage of rubber dissolution is often used to differentiate different types of CRMB, e.g. AR and TB. Swelling and complete dissolution of rubber are two opposite ends of the interaction of bitumen and rubber depending on the interaction conditions (Ghavibazoo et al. Citation2013b).
3.1. Rubber swelling
Swelling of polymers is a volume expansion process of the polymer network due to absorption of solvents. For uncrosslinked polymers ((a)), when exposed to suitable solvents, they can be easily swollen up and eventually dissolve into the solvent. This process is called polymer dissolution which will be discussed in the following section. The dissolution of an uncrosslinked polymer into a solvent involves two phenomena, i.e. solvent diffusion-induced swelling and chain disentanglement (Tu and Ouano Citation1977). It should be pointed out that the definition of polymer dissolution here is different from the term ‘dissolution’ used for rubber dissolution previously in an engineering context. For crosslinked polymer systems in which links between chains or segments are established ((b)), because of the constraints of the network structure, the network might be swollen by absorbing solvents but the dissolution will hardly occur. Limited swelling is a characteristic property of polymers with network structures (Flory and Rehner Citation1943). As clarified in the previous section, tire rubber contains a large portion of vulcanised/crosslinked polymer and a small portion uncrosslinked polymer. Therefore, swelling of tire rubber in organic solvents is a partial dissolution process (Stroup-Gardiner et al. Citation1993, Artamendi and Khalid Citation2006). Depending on the compatibility between rubber and solvents, the swelling extent and dissolution rate may vary. When mixing rubber with bitumen at high temperatures, the light fractions of bitumen will diffuse into the rubber network and cause its swelling. The volume change of rubber particles and the formation of the gel layer adjacent to the rubber–bitumen interface reduces the inter-particle distance and changes the component proportions of the remaining bitumen and, therefore, stiffens the composite material.
Figure 4. Schematic representation of the molecules of (a) the uncrosslinked polymer and (b) the crosslinked polymer (links are pictured as knots), adapted from Mark et al. (Citation2013).
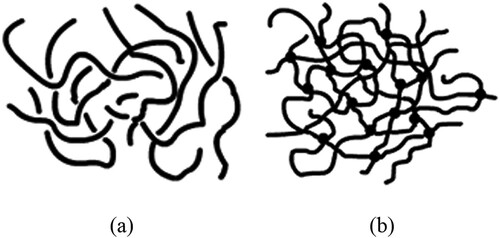
3.2. Chemical degradation
Under severe interaction conditions (excessively high mixing temperature with high shear and long mixing times), rubber network degradation occurs. Even though the network structure formed by crosslinking prevent the dissolution of rubber molecules, the high thermal energy and shearing energy induced in the mixing process will destroy the crosslinking and hence the rubber network. This process involves the scission of disulphide bond (S–S), carbon–sulphur bond (C–S) and carbon–carbon bond (C–C), which eventually results in the breakage of crosslinked bonds and backbone chain bonds reducing thus the average molecular weight of rubber (Zanzotto and Kennepohl Citation1996). This degradation process is referred to as devulcanisation and depolymerisation, respectively (Abdelrahman and Carpenter Citation1999). The average bond energies of different bonds are listed in (Chemistry LibreTexts Library Citation2017). Based on the relative bond energies of S–S, C–S and C–C bonds (with the order of C–S < S–S < C–C), the S–S bond or C–S bond is less stable than C–C bond in the presence of energy perturbations, such as chemical and thermal attacks. Therefore, the scission of S–S and S–C takes place earlier and destroys the network formed by crosslinking. That is why during the mixing process of the bitumen–rubber blend at high temperatures, the acrid smell from sulfoxide may be detected. The rubber network degradation removes the constraints for the polymer chains. The free rubber polymer chains will eventually dissolve into bitumen. The mixing energy imposed by the mixer during interaction can accelerate the swelling process of rubber particles and enhance the size-reduction effects. The chemical degradation of rubber networks is adverse to the mechanical property development of binders (Tang et al. Citation2016, Yao et al. Citation2016) but beneficial to improve the binder storage stability (Lo Presti et al. Citation2018).
Table 3. Average bond energies of chemical bonds typically in CRMB.
3.3 Other component exchange
The previous two sub-sections discussed the behaviour of rubber polymer when interacting with bitumen. However, in reality, tire rubber is a mixture of different components. During the bitumen–rubber interaction process, oily components, carbon black and some inorganic fillers bound to rubber are also released under high interaction temperatures and mixing forces. Considering the large proportion of these non-polymer components, the effects of their release into bitumen on the binder properties cannot be ignored. It was reported that these non-polymer components significantly influence the ageing and rheological properties of CRMB (Ghavibazoo et al. Citation2015, Wang et al. Citation2019c).
In summary, the interaction stages of bitumen–rubber can be divided into three steps as shown in (Wang et al. Citation2017a). Stage 0: initial configuration. Rubber particles are just immersed in the fluid bitumen. Stage 1: swelling phase. Rubber particles start swelling by absorbing the light fractions of bitumen and form a gel layer adjacent to the bitumen–rubber interface. Stage 2: post-swelling and beginning of degradation. The swelling of rubber particles continues happening. Meanwhile, chemical degradation takes place through the breakup of the crosslinked network and polymer chains. Swollen rubber particles are split into smaller ones due to the destruction of network structure. Stage 3: degradation and complete dissolution. The degradation of rubber particles continues progressing until they are completely dissolved into the bitumen matrix, which produces a homogenous binder. It is noteworthy that the different interaction stages may overlap. The degradation of the rubber network enhances the absorption of light components from bitumen to the remaining rubber for swelling. In turn, swelling also speeds up the degradation rate of rubber. In reality, there is a swelling gradient of rubber from the outer layer to the inner core.
Figure 5. Interaction stages of bitumen and rubber, adapted from Wang et al. (Citation2017a).
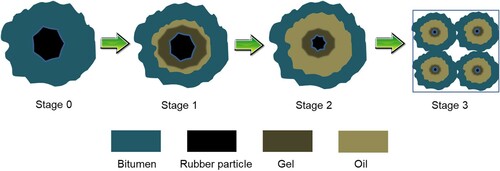
4. Theoretical considerations of bitumen–rubber interaction
4.1. Relationship between bitumen–rubber interaction and polymer dissolution in solvents
Looking back to the interaction between bitumen and rubber, it has similarities as the polymer dissolution process but is not equal to each other. In the following analysis, bitumen is generally considered as the low-molecular-weight solvent, while rubber is a polymer with high molecular weight. The complex compositions of the rubber system increase the complexity of the interaction mechanism with bitumen. The uncrosslinked and crosslinked parts in rubber have different behaviours when interacting with bitumen. Combining the experimental findings in bitumen–rubber interactions and the knowledge of polymer dissolution, summarises the interaction mechanisms of rubber with bitumen at different stages. The devulcanisation process will break the crosslinks and make chain disentanglement happen. It should be emphasised that each interaction step does not necessarily follow the exact sequence as shown in . In most instances, rubber swelling and degradation occur simultaneously because of the diversity of rubber particle size. Fine particles finish the swelling stage in no time and start the degradation stage. Similarly, chain disentanglement, devulcanisation and depolymerisation can also overlap. The theoretical aspects of rubber swelling and degradation stages will be described as follows.
As illustrated in , polymer dissolution in solvents is actually a two-step process, which consists of: (a) diffusion-induced swelling of the polymer network and (b) chain disentanglement of the swollen polymer. The dissolution of a polymer in a solvent is usually a very slow process depending on the microstructure and chemical composition of a given polymer as well as the surrounding environment. shows the dissolution process of an uncrosslinked polymer. Polymer molecules consist of long chains with large numbers of segments, forming tightly folded coils which are entangled to each other. When the polymer is just added into a thermodynamically miscible solvent, the coils still hold together as a solid due to the cohesive and attractive intra- and intermolecular forces, (a). Later on, due to polymer–solvent interactions, segments with polymer chains start to imbibe solvent molecules, increasing the volume of the polymeric network and forming a swollen polymeric gel, (b). The swollen gel exhibits elastic rather than plastic properties. Once the swelling reaches a critical point, which means all segments are solvated but remaining in contact due to strong polymer interactions, the polymer chains start disentangling from the bulk. The whole loosen/unfolded coils will slowly diffuse out of the swollen polymer gel and eventually disperse into the solution. When all the chain segments are dispersed into the solvent, the solvent molecules will fill the empty space between the loose segments, occupying a spherical or ellipsoidal volume, (c). This apparent volume occupied by the swollen polymer coil with the absorbed solvent is called hydrodynamic volume. Therefore, there are two transport mechanisms in this dissolution process as shown in , i.e. solvent diffusion and chain disentanglement. The rubbery–solvent interface moves towards the solvent, while the glassy–rubbery interface moves towards the glassy part of the polymer (Narasimhan Citation2001).
Figure 7. Schematic representation of the dissolution process for polymer molecules, blue lines represent polymer chains and yellow dots represent solvent molecules. (a) polymer molecules in solid state just after being added to a solvent; (b) a swollen polymeric gel; (c) solvated polymer molecules dispersed into a solution.
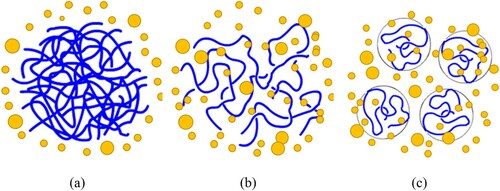
Figure 8. A schematic diagram of a one-dimensional solvent diffusion and polymer dissolution process, adapted from Narasimhan (Citation2001).
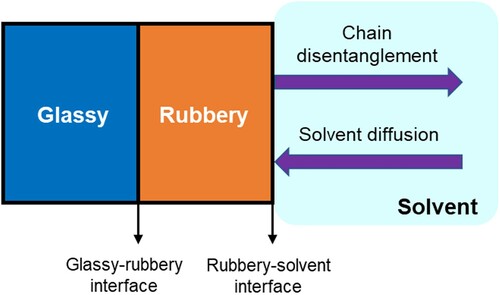
To have more insights into the microstructural changes of the polymer during dissolution, displays the evolution of polymer chains from a spatial viewpoint. It can be found polymer chains are distributed in different states at different positions, implying different microstructures of the polymer. Many researchers have proved the formation of a multilayer structure during the polymer dissolution process (Miller-Chou and Koenig Citation2003). The structure of the surface layer between the pure polymer and the pure solvent is summarised as follows: the infiltration layer, the solid swollen layer, the gel layer and the liquid layer (). This structure classification is based on the glassy polymers. However, some of the layers may not be formed during dissolution depending on the polymer and solvent properties as well as the interaction conditions. Adjacent to the core of pure polymer is the infiltration layer. The solid swollen layer is established for the polymer–solvent system in the glassy state. When swelling equilibrium achieves, the penetrated solvent molecules start to push the polymer molecules into the solvent. During this transport process of polymer substance, chain disentanglement may happen as shown in . In the gel layer, polymer chains start disentangling when the solvent concentration inside the polymer chain reaches the critical gel concentration (Peppas et al. Citation1994). At this moment, the two interfaces (polymer–gel and gel–solvent) proceed at the same velocity. As time passes, a more dilute upper layer called the liquid layer is formed to the direction of the pure solvent. As the glassy–rubbery interface continues to move inward (to the direction of polymer core) in , the glassy core gradually disappear. As a result, the rubbery–solvent interface keeps moving outward until the complete polymer dissolution is achieved (Vrentas and Vrentas Citation1998). Above is the schematic description of the polymer dissolution process. Several other models were also developed to explain the polymer dissolution behaviours: phenomenological models with diffusion equations (Tu and Ouano Citation1977), external mass transfer models (Lee and Peppas Citation1987), stress relaxation models with the reptation theory (Brochard and Degennes Citation1983), anomalous transport models and scaling laws (Peppas et al. Citation1994), continuum framework models (Narasimhan and Peppas Citation1996b).
Figure 10. Schematic representation of the surface layer structure, adapted from Miller-Chou and Koenig (Citation2003).

Therefore, an entire bitumen–rubber interaction involves polymer dissolution and many other reactions. The kinetics and thermodynamics framework of bitumen–rubber systems is discussed in the next sub-sections following the sequence of the aforementioned phenomena.
4.2. Diffusion of bitumen into rubber
When rubber networks are exposed to bitumen, certain fractions of the bitumen (mostly the low-molecular-weight maltenes) diffuse into and are imbibed by the polymer network, causing its swelling. This diffusion process continues until the solvent concentrations inside and outside the polymer are equal to each other. Previous studies have shown that the Fickian model is most appropriate for describing the swelling of rubbery polymers (Papanu et al. Citation1989). The kinetics of bitumen diffusion into rubber is usually described by Fick’s laws of diffusion (Artamendi and Khalid Citation2006, Wang et al. Citation2019a). Fick’s second law predicts the evolution of bitumen concentration with time due to the progress of diffusion into rubber as(1)
(1) where C is the concentration of diffusing substance;
is time; and D is the diffusion coefficient. For the Fickian diffusion, it is obvious that the concentration gradient is the driving force for the diffusion process. However, for non-ideal systems including CRMB, the diffusion is often driven by the gradient of chemical potential, which can be described by the Cahn–Hilliard equation
(2)
(2) where
is the local polymer fraction;
is the mobility coefficient; and
is the total free energy of the system. The chemical potential is defined as
. The Cahn–Hilliard equation has a similar form to Fick’s second law. In the system, the diffusion rate is positively correlated to the mobility coefficient. The Cahn–Hilliard equation is useful when considering the thermodynamics of swelling equilibrium.
There are many methods for measuring the diffusion of materials in polymers, such as optical method, gravimetric method, vapour sorption, employing radioactive trace materials and interferometer procedures (Buckley et al. Citation1962, Buckley and Berger Citation1962). However, because of the complexity and particularity of the combination of rubber and bitumen, the conventional gravimetric method is often used to monitor the diffusion process. Laboratory swelling tests with a rectangular rubber block have been done to obtain this parameter (Frantzis Citation2004, Artamendi and Khalid Citation2006, Feng et al. Citation2015). Following the initial linear region, it was found a clearly defined equilibrium plateau region occurred. For other geometrical rubber specimens, for instance, cylindrical specimens, the diffusion coefficient can be obtained through the analytical solutions of one-, two- and three-dimensional diffusion models (Luo et al. Citation2017, Luo and Huang Citation2018).
4.3. Equilibrium swelling of rubber in bitumen
4.3.1. The Flory–Rehner theory
The classical benchmark theory for describing the swelling process in polymer gels is the Flory–Rehner theory (Quesada-Pérez et al. Citation2011). Based on this theory, the thermodynamic force of mixing (osmotic force) and the retractive force of the polymer chains (elastic force) are the two opposing forces competing to determine the swelling behaviour in gels. On swelling, each polymer network strand is stretched, and the crosslink junctions move further apart. Against the osmotic force, there is an opposite elastic force, which balances the stretching of the network and opposes its deformation. When the osmotic pressure is balanced by the elastic restraint, swelling reaches its equilibrium state (Ganji et al. Citation2010). According to the Flory–Rehner theory, the thermodynamic equilibrium of a gel is reached when the chemical potential of the solvent (designated by ) is equal inside and outside the gel
(3)
(3) Using the concept of osmotic pressure (
), Equation (3) can be rewritten in the following form
(4)
(4)
is the molar volume of bitumen. The osmotic pressure, defined as the rate of change of the total free energy of the polymer–solvent system, can be calculated using the following equation:
(5)
(5) where
is Avogadro’s number;
is the number of bitumen molecules;
is the change of Helmholtz free energy since the rubber–bitumen system is theoretically incompressible. The free energy change during the swelling process in the case of incompressible system is
(6)
(6) where
is the change of total free energy in the gel,
is the change of free energy of mixing and
is the change of elastic free energy. Equilibrium swelling is achieved by minimisation of the Helmholtz free energy. In the Flory–Rehner theory, the mixing free energy is formulated with the Flory–Huggins equation
(7)
(7) where
is the Boltzmann constant; T is the temperature;
is the local volume fraction of solvent (bitumen);
is the local volume fraction of rubber in a swollen state, defined by Equation (8); χ is the interaction parameter between the bitumen and rubber;
is the number of bitumen molecules
(8)
(8) Here
is the volume of the rubber gel (including the absorbed solvent within it) at a given state and
is the volume of the dry rubber. The simplest model that captures the idea of rubber elasticity is the affine network model (Flory Citation1950, Citation1985), which assumes an affine deformation: the relative deformation of each network strand and the macroscopic relative deformation of the whole network are the same. The free energy required to deform a network mainly arises from the change of entropy associated with an isotropic deformation
(9)
(9) where
is the number of effective chains in the network;
is the linear swelling ratio, which can be computed with the following equation by involving the molar volume of the solvent
(10)
(10) The chemical potential (Rubinstein and Colby Citation2003) of the bitumen in the rubber gel is defined as
(11)
(11) Incorporating Equations (7), (9) and (10) into Equation (11) yields
(12)
(12) When reaching equilibrium, the chemical potential of the solvent inside and outside the swollen polymer will be equal to each other, which means the left side of Equation (12) will be cancelled. Rearranging Equation (12) gives
(13)
(13) The number of effective chains per unit volume can be linked with the specific volume of rubber
and the molecular weight between crosslinks
as follows:
(14)
(14) Recalling the definition of crosslink density
(Ganji et al. Citation2010), which is a measure of crosslinked points per unit volume (mol/cm3), gives the final expression through further rearrangement
(15)
(15) The term
in common equation for calculating the crosslink density is eliminated due to the fact that the molecular weight of the rubber is much higher than
. It should be noted that the previous equations are derived for the crosslinked polymer system. For the polymer system with only entanglements, the number of moles of crosslinks can be replaced by the number of moles of entanglements (Papanu et al. Citation1989, Narasimhan and Peppas Citation1996a). Then, similar calculations can be performed. Ideally, through laboratory experiments, the swelling ratio of rubber (
) in certain solvents can be measured by different methods. In addition, with known values of the Flory interaction parameter, both crosslink density and a molecular weight between crosslinks can be further derived through Equation (17) when the solvent is determined. On the other hand, if the crosslink density of rubber and the Flory interaction parameter between rubber and bitumen are known in advance, the equilibrium swelling ratio of rubber in bitumen can be predicted.
4.3.2. The Flory–Huggins parameter
The Flory interaction parameter in the Flory–Huggins equation for polymer solutions is of prime importance since it describes the thermodynamic state of polymer–solvent interaction in a given mixture. This parameter is defined to characterise the difference of interaction energies in the mixture. If there is a net attraction between species from polymer and solvent (i.e. they like each other better than they like themselves),
and a single-phase mixture is favourable for all compositions. However, the most often situation is that a net repulsion exists between species (i.e. they like themselves more than each other) and this will oppose the mixing process. Therefore, the sign of the interaction parameter
determines the energetic tropism for mixing, that is positive for opposing mixing, zero for ideal mixtures and negative for promoting mixing (Rubinstein and Colby Citation2003).
Several methods (Liu and Shi Citation2008), such as vapour pressure lowering, osmometry, light scattering and inverse gas chromatography, were proposed to estimate the value of . However, these tests are generally time-consuming and need cautious operations. With the help of solubility parameters, the interaction parameter can be rapidly estimated. The solubility parameter is a good indicator of solubility of a specific solvent. It is very useful to predict miscibility and compatibility of polymers. Liquids with similar solubility parameters will be miscible, and polymers will dissolve into solvents whose solubility parameters are close to their own (Rubinstein and Colby Citation2003). For non-polar, non-associating polymer–solvent system with species interacting mainly by dispersion forces, the interaction parameter
can be estimated from the Hildebrand solubility parameters as (Hansen Citation2002)
(16)
(16) where
is the molar volume of the solvent;
and
are the Hildebrand solubility parameters for the solvent and polymer, respectively; R is the universal gas constant; T is the absolute temperature;
is the empirical constant. However, for complex polymer systems, the Hansen solubility parameters (HSP), which consider the non-polar/dispersion forces, the polar forces and hydrogen bonding forces, usually provides a better approximation. Previous studies have successfully applied the HSP to express the solubility and internal stability of bitumen (Redelius Citation2004). A similar equation to estimate the interaction parameter based on the HSP can be obtained (Hansen Citation2002)
(17)
(17) where
,
and
are, respectively, the dispersive, polar and hydrogen bonding components of the HSP. Because polymers are not volatile,
is often obtained through an indirect method in which polymers are mixed with a series of solvents of varying but known solubility parameters. The solubility parameter of the polymer is taken as the
value of the solvent which enables the maximum extent of swelling (Redelius Citation2000, Citation2004). Comparing to the average solubility parameter values, the solubility body of a material in the three-dimensional Hansen space is more realistic and useful (Zhu et al. Citation2019).
4.4. Disentanglement of rubber polymer chains
As discussed previously, polymer chains will disentangle if contacted with thermodynamically compatible solvents. Disentanglement occurs in a form of diffusional motions of chains out of the swollen polymer gel. The disentanglement of polymer chains can be described by the famous reptation model (De Gennes Citation1971). In the reptation model, an entangled chain diffuses along its confining tube as shown in . The time needed for the chain to diffuse out of its original tube is the reptation time . It is predicted to be proportional to the square of the chain size (radius of gyration)
divided by the reptation diffusion coefficient
(Equation (20))
(18)
(18) In the context of CRMB system, it is important to know the rubber dissolution rate in bitumen to control the binder properties. The dissolution rate is related to the disentanglement rate
of the polymer chain, which is taken to be proportional to the chain size divided by the reptation time. Thus
(19)
(19) It is reported that
and
can be related to polymer molecular weight and concentration (Papanu et al. Citation1989). Therefore, the disentanglement rate of rubber in bitumen can be expressed as
(20)
(20) where A is an empirical constant;
is the molecular weight of rubber; a is related to the rubber molecular weight distribution and b is related to rubber concentration. Both a and b are larger than 1.
4.5. Chain scission of rubber network
As mentioned before, during the degradation process of rubber into bitumen, crosslinked bonds and main chain bonds will break up successively based on the interaction condition. For three-dimensional crosslinked network of rubber, the chain scission reaction has three limiting cases: (a) chain scission at random; (b) cleavage of crosslinks and (c) directed scission. It was found that the soluble fraction of a network that has undergone scission can be linked to the effective number of chains in the gel fraction using a simple relationship (Horikx Citation1956). Specifically, the number of scissions can be measured by the soluble fraction of in the degraded network. This offers an opportunity to monitor the rubber network degradation process in bitumen by measuring the dissolving fraction of rubber. The effective number of chains can be determined by swelling measurements (Flory and Rehner Citation1943).
For the case of chain scission at random, the total number of crosslinks is assumed to be constant during the degradation reaction. Therefore, the following relationship in a rubber can be established(21)
(21) where
is the soluble fraction in the crosslinked rubber and
is the effective number of chains in the gel fraction (De Sousa et al. Citation2017). In the above equations, subscript ‘1’ and ‘2’, respectively, indicates the states before and after the degradation (chain scission reaction).
The soluble fraction in a polymer is given by (Charlesby Citation1953)(22)
(22) where
is the crosslinking index of the whole polymer (average number of crosslinks per original chain). The crosslinking index can be calculated by
(23)
(23) where
is the number of primary molecules of polymer. Therefore, the number of chain scissions (n) of polymer is given by
(24)
(24) For the case of crosslinking scission, no chain scission but only severance of crosslinks happens. Equation (21) becomes as follows:
(25)
(25) The number of scissions is equal to the number of severed crosslinks given by
(26)
(26) For the case of directed scission, only qualitative conclusions can be drawn because of the invalidity of the interchange of the crosslinking and scission reactions. To sum up, the number of scissions can be computed from the soluble fraction of a degraded network, as determined by the effective number of chains in the gel fraction with the help of swelling measurements.
5. Critical factors in practice and their theoretical bases
Similar to polymer modified bituminous binders, the interaction conditions and raw material characteristics will have a great impact on the rubber swelling and degradation process and consequently the binder properties. A thorough understanding of these factors will guide the material selection and process optimisation to obtain the desired properties from the modification.
5.1. Effect of interaction conditions
5.1.1. Temperature
The temperature has a great influence on both bitumen and rubber as they are both temperature-sensitive materials. When the temperature increases, both the mobility of bitumen molecules and rubber polymer chains increase, which enhances the diffusion process of bitumen into the rubber (Rubinstein and Colby Citation2003). The mobility and diffusion coefficients are highly dependent on temperature. From Equations (1) and (2), it can be found that at higher temperatures, the diffusion speed of bitumen into rubber and associated swelling rate of rubber greatly increase, which are also verified by experimental results (Green and Tolonen Citation1977, Artamendi and Khalid Citation2006, Wang et al. Citation2020). summarises the diffusion coefficients and equilibrium swelling data from different researchers. Theoretically, as the temperature increases, the equilibrium swelling extent of rubber decreases because the rubber network is harder to expand due to the entropy-driven retractive forces as the temperature increases (Mark Citation1981). However, experimental data usually show an increase in swelling with temperature, indicating other reactions which decrease the crosslink density may occur (i.e. chain disentanglement, devulcanisation etc.) (Green and Tolonen Citation1977). Moreover, the main conditioning parameter that influences the bitumen–rubber interaction is the temperature while interaction duration and mixing speed contribute significantly as well but with minor effects on the rubber dissolution mechanism (Ghavibazoo et al. Citation2013b). Only when reaching certain temperatures, the chemical degradation of rubber occurs. Depending on the CRM composition, the chemical degradation starts at different temperatures. At an intermediate interaction temperature (e.g. 190°C), rubber dissolution in bitumen can happen with the help of high-speed mixing, which generates a more homogenous blend (Ghavibazoo et al. Citation2013b). On the other hand, a lower interaction temperature (e.g. 160°C) was not sufficient to trigger the formation of the three-dimensional network even at high mixing speeds. Also, a higher interaction temperature (e.g. 220–240°C) led to the dramatic degradation of rubber in bitumen, hence the degradation of rheological properties of the modified blend (Ragab et al. Citation2013, Yao et al. Citation2016, Wang et al. Citation2018b).
Table 4. Diffusion coefficients and equilibrium mass uptake of bitumen into rubber.
5.1.2. Time
The interaction between bitumen and rubber is a continuous process which needs a certain time to achieve desired properties. The properties of CRMB developed significantly during the early stage of interaction (e.g. the first 30 min). This coincides with the results of the diffusion process in which solvent diffuses into polymer faster at the early stage and slows down later until reaches the diffusion equilibrium. Through simulated swelling of rubber particles with SBR sheets, the swelling process increases enormously in the beginning and then reaches an equilibrium. However, as the swelling time increases, the crosslinking network of rubber is destroyed resulting in a decrease in crosslink density and an increase in the glass transition temperature of rubber (Frantzis Citation2004, Artamendi and Khalid Citation2006, Dong et al. Citation2012). A decrease in apparent viscosity of the bitumen–rubber system is noticed. Later, continuing extending the interaction time has a minor effect on the physical properties of CRMB (Abdelrahman Citation2006). To sum up, schematically plots the viscosity evolution curves of CRMB over time at different interaction temperatures (Abdelrahman Citation2006, Lo Presti and Airey Citation2013). At low interaction temperatures (∼160°C), rubber swelling is dominating the interaction process, the viscosity keep increasing until reach a plateau. At intermediate interaction temperatures (∼180°C), the rubber in bitumen first experiences the swelling stage and then starts the chemical degradation stage, which is reflected by the decreased viscosity. At high interaction temperatures (>200°C), rubber swelling finishes in a very short time and chemical degradation plays a dominant role during the interaction. Different interaction temperatures correspond to different types of rubberised binder products, which can be determined based on the user requirements.
In addition, increasing the mixing speed has an equivalent effect of extending mixing time. However, the effect of interaction time and mixing speed is highly dependent on the interaction temperature which has dominant effects during the processing. The other two parameters can only facilitate the interaction process when the temperature meets the critical value (Ghavibazoo et al. Citation2013b). An ambiguous time–temperature superposition principle may exist, meaning increasing the interaction temperature can decrease the required mixing time to achieve comparable results.
5.1.3. Mixer type
The mixer type also influences the property development of CRMB. Conventionally, there are two types of mixers that exert the mechanical mixing energy during the bitumen modification process: the common blade type mixer and the high shear mixer. The mixing head varies with different application scenarios. The main difference is whether shearing energy is applied or not. Using high shear mixing can significantly reduce the rubber particle size, accelerating the interaction process. In addition, the exerted mixing energy can break the bonding between rubber particles and contribute to reducing the agglomeration (Celauro et al. Citation2012). Furthermore, carbon black and inorganic fillers attached on the rubber network are released into the bitumen under the high shear force (Abdelrahman Citation2006, Attia and Abdelrahman Citation2009). It was reported that carbon black also tends to absorb the lightweight fraction of bitumen. The existence of carbon black in bitumen improves the anti-ageing, high-temperature performance and thermal conductivity (Cong et al. Citation2014, Wang et al. Citation2019c).
5.2. Effect of rubber particle characteristics
5.2.1. Tire type
The main difference between truck tires and car tires is the different proportions of NR, SR and other ingredients they consist of. Truck tire rubber consists mainly of NR, whereas car tire rubber consists of more SR. From the chemical structure of different rubbers shown before, it can be found that SR with a more complex network is less susceptible to degradation than NR. In addition, it is easier for NR to swell in bitumen due to the relatively simple long-chain structure with less network constraints. Therefore, under the same interaction conditions, bitumen diffusion into truck tire rubber is usually faster than into car tire rubber and the equilibrium swelling extent of truck tire rubber is also higher than car tire rubber as reflected by the experimental data in (Artamendi and Khalid Citation2006). From Equation (20), it can be found that polymers with higher molecular weight have a lower dissolution rate (Miller-Chou and Koenig Citation2003). This indicates that NR is also easier to be dissolved into hot bitumen than SR.
5.2.2. Morphology and processing method
There are two conventional methods of processing scrap tires: ambient grinding and cryogenic grinding. Ambient ground tire rubber particles usually have irregular shapes and porous appearance. In contrast, rubber particles produced through the cryogenic process are found to be more angular with a smooth cracked surface. The surface area of ambient ground rubber particles is about twice larger than the cryogenic counterpart (Shen et al. Citation2009). The large specific surface area of rubber particles increases the chances of contact with bitumen, hence promoting the interaction with bitumen. The resulted faster absorption of light fractions from bitumen into rubber causes more swelling and enhances the binder properties (Billiter et al. Citation1997). Other studies also suggested that CRMB binders prepared with ambient ground crumb rubber exhibited higher viscosity and higher elasticity with higher complex modulus and lower phase angle (Shen and Amirkhanian Citation2007, Lee et al. Citation2008).
5.2.3. Rubber particle size
Regarding the size of crumb rubber particles, it has a significant influence on its swelling kinetics and degradation into the bitumen, considering also that swelling is a Fickian diffusion process. For one-dimensional diffusion, the following relationship can be derived from Equation (1)(27)
(27) where
and
represent the total mass of the diffused substance at time t and at equilibrium, respectively;
is the sample thickness and
is the diffusion coefficient. Therefore, the required interaction time for rubber with bitumen to achieve the same swelling ratio increases with the square of the particle size (Buckley and Berger Citation1962). shows the swelling ratio change of different size rubber particles (from 0.2 to 1.0 mm) with time based on the numerical simulation. Fine rubber particles require less time to react and thus swell faster and achieve the swelling equilibrium earlier than coarse rubber particles (Wang et al. Citation2019a). Consequently, fine rubber particles also degrade faster, affecting the properties of the binder liquid phase. However, coarse rubber particles need more time to reach the swelling equilibrium. They have more influence on the binder instead of the liquid phase because of their higher swelling volume and less degradation rate (Abdelrahman and Carpenter Citation1999). They also yield higher complex modulus/viscosity and lower phase angle values of CRMB than finer particles do under the same interaction conditions (Attia and Abdelrahman Citation2009, Shen et al. Citation2009). Reducing crumb rubber size can produce a more homogenous bitumen–rubber blend and improve the storage stability of binders (Ghavibazoo et al. Citation2013a). However, CRM with finer sizes significantly increases the cost because of the required additional grinding.
5.2.4. Rubber particle content
From the micromechanical theory of particulate-filled composite materials, the particulate content is always of great importance to the mechanical properties of the composite system. Many studies have confirmed that the rheological properties and performance-related parameters of CRMB are functions of the rubber content (Huang Citation2008, Lee et al. Citation2008). Unlike the common aggregate fillers which primarily have stiffening effects in bituminous binders, CRM particles also result in physiochemical interactions with bitumen which will alter both the bitumen composition and the rubber microstructure. From the multilayer structure of swollen rubber in , the mechanical properties of rubber after swelling will be changed. Results show that swollen rubber is softer than dry rubber due to the absorption of bitumen and exhibit noteworthy viscoelasticity (Wang et al. Citation2020). Based on the micromechanical models, the reinforcing effect of CRM on the binders will be enhanced with the increasing rubber content (Huang Citation2008, Medina and Underwood Citation2017, Wang et al. Citation2020).
5.2.5. Pre-treatment of CRM
The pre-treatment of CRM particles influences the interaction efficiency between rubber and bitumen. As mentioned before, the crosslinks in rubber formed by vulcanisation can significantly prevent the rubber from swelling by absorbing organic solvents. The concept of devulcanisation is introduced as selective scission of crosslinks with negligible main chain scission. Therefore, CRM pre-treated with devulcanisation can have a better interaction (swelling) with bitumen. Various devulcanisation processes have been developed by the rubber industry, including thermochemical, thermo-mechanical, microwave, ultrasonic, biotechnological devulcanisation (Joseph et al. Citation2016). Microwave (Ma et al. Citation2015) and ultrasonic methods (Xu et al. Citation2015) were reported to activate crumb rubber and promote the miscibility between bitumen and crumb rubber. CRMB prepared with activated crumb rubber exhibited superior storage stability and low-temperature performance. It is also common to use organic solvents to pretreat CRM to improve the miscibility (Subhy et al. Citation2015). Besides the physical treatment of CRM, chemical modification of CRMB was also achieved by adding activators or other chemicals. For instance, trans-polyoctenamer rubber (TOR) can promote the interaction between the sulphur in the rubber network and the sulphur within the asphaltenes (Liang et al. Citation2017). Polyphosphoric acid (PPA) can react with many functional groups in bitumen. It breaks the asphaltenes agglomerates and creates the possibility for better dispersion of asphaltenes in the maltenes phase, which allows for better interactions with rubber (Yadollahi and Mollahosseini Citation2011). This either enhances the performance of CRMB or increases the storage stability of CRMB.
5.3. Effect of bitumen characteristics
Bitumen from different crude oil sources usually has varying chemistry, with different SARA fractions. This will influence the compatibility with crumb rubber thereof the swelling of rubber. It is reported that the total solubility parameters of NR and SBR are around 16.9 and 18.1 MPa0.5, respectively (Mark et al. Citation2013). In addition, solubility parameters for bitumens are in the range 17.2–18.8 MPa0.5 (Zhu et al. Citation2019). Thus, the similarity between the solubility parameters of bitumen and natural and SR indicates that bitumen is a swelling agent of both rubbers. Recalling the solubility parameters of different fractions of bitumen, then, theoretically, aromatics with a solubility parameter of 17–18.5 MPa0.5 should have the highest miscibility with crumb rubber from scrap tires based on the classical ‘like dissolve like’ compatibility principle. Therefore, in general, softer bituminous binders with lower molecular weight are more prone to diffuse into and absorbed within the rubber network (Artamendi and Khalid Citation2006). Various laboratory tests of preparing CRMBs have validated the above statement. Among the same graded bitumens, the one with a higher fractions of maltenes yields a higher swelling extent of crumb rubber particles, leading to faster dissolution during the later interactions (Airey et al. Citation2004).
6. Conclusions and recommendations
The interaction between rubber and bitumen plays a very important role in controlling the properties of rubberised bituminous binders. From the viewpoint of polymer science, in the binary binder system, bitumen can be regarded as the low-molecular-weight solvent, while rubber can be regarded as the polymer with high molecular weight. The bitumen–rubber interaction at high temperatures is generally a rubber dissolution process (which consists of swelling and chain disentanglement) and a chemical degradation process. In principle, the rubber swelling process can be systematically modelled by the Flory–Rehner theory in combination with the diffusion theory. The chain disentanglement of the swollen polymer network is a subsequent process to swelling. The chemical degradation of rubber in bitumen mainly includes the chain scission reactions which are observed as devulcanisation and depolymerisation. Admittedly, it is of great difficulty to do quantitative analysis on the model in the context of rubber swelling in bitumen since both rubber and bitumen are very complex systems instead of pure matters. However, the fundamental knowledge in polymer–solvent interaction is still of great importance to understand the interaction process and guide the material selection and process optimisation to obtain desired binder properties.
The effects of interaction conditions (temperature, time and mixing energy) and raw material characteristics (rubber type, particle size, particle content, pre-treatment, etc.) were analysed based on the proposed theoretical framework. These critical factors can fundamentally alter the bitumen–rubber interaction parameters, and consequently the binder properties. In view of the diversity of raw materials (bitumen and rubber) and varying demands of binder properties, it is difficult to draw any universal conclusion. However, the following recommendations are made in terms of specific application scenarios.
Temperature is the dominant factor that determines the product type of rubberised binders. Different CRMB products can be produced by manipulating the interaction temperature ranges which correspond to different interaction mechanisms.
To maximise the swelling effect of rubber in bitumen, bitumen with more maltenes (soft grade bitumen) and tire rubber high NR components should be chosen to create resemblance in solubility parameters.
Devulcanised rubber is encouraged to be used in bitumen modification to eliminate the acrid smell and to improve the mechanical property and storage stability of rubberised binders.
For future research, the following points are recommended to enhance the understanding of this type of material and use it in a more rational way.
The volumetric, chemical and mechanical properties of rubber in the blend during interaction should be monitored. The rubber in the blend undergoes multiple effects, including thermal ageing, diffusion of bitumen, mechanical energy from mixing. It is useful to know the nature of rubber before and after the interaction to control the binder property and to predict its behaviour in the long-term service.
More dedicated laboratory tests should be done to establish a database for different bitumen and rubber systems, for instance, solubility parameter, diffusion coefficient, swelling coefficient, dissolution rate, etc.
Disclosure statement
No potential conflict of interest was reported by the author(s).
Additional information
Funding
References
- Abdelrahman, M., 2006. Controlling performance of crumb rubber-modified binders through addition of polymer modifiers. Transportation Research Record: Journal of the Transportation Research Board, 1962, 64–70. Available from: <Go to ISI>://WOS:000243748500008.
- Abdelrahman, M.A. and Carpenter, S.H., 1999. Mechanism of the interaction of asphalt cement with crumb rubber modifier. Transportation Research Record: Journal of the Transportation Research Board, 1661, 106–113.
- Airey, G., Rahman, M., and Collop, A.C., 2004. Crumb rubber and bitumen interaction as a function of crude source and bitumen viscosity. Road Materials and Pavement Design, 5 (4), 453–475.
- Artamendi, I. and Khalid, H.A., 2006. Diffusion kinetics of bitumen into waste tyre rubber. Journal of the Association of Asphalt Paving Technologists, 75, 133–164. Available from: <Go to ISI>://WOS:000243589000004.
- Attia, M. and Abdelrahman, M., 2009. Enhancing the performance of crumb rubber-modified binders through varying the interaction conditions. International Journal of Pavement Engineering, 10 (6), 423–434. Available from: <Go to ISI>://WOS:000272700900003.
- Billiter, T., et al., 1997. Production of asphalt-rubber binders by high-cure conditions. Transportation Research Record: Journal of the Transportation Research Board, 1586, 50–56.
- Brochard, F. and Degennes, P., 1983. Kinetics of polymer dissolution. PhysicoChemical Hydrodynamics, 4 (4), 313–322.
- Buckley, D.J. and Berger, M., 1962. The swelling of polymer systems in solvents. II. Mathematics of diffusion. Journal of Polymer Science, 56 (163), 175–188.
- Buckley, D.J., Berger, M., and Poller, D., 1962. The swelling of polymer systems in solvents. I. Method for obtaining complete swelling–time curves. Journal of Polymer Science, 56 (163), 163–174.
- Burfield, D.R. and Lim, K.L., 1983. Differential scanning calorimetry analysis of natural rubber and related polyisoprenes. Measurement of the glass transition temperature. Macromolecules, 16 (7), 1170–1175.
- Celauro, B., et al., 2012. Definition of a laboratory optimization protocol for road bitumen improved with recycled tire rubber. Construction and Building Materials, 37, 562–572.
- Charlesby, A., 1953. Solubility and molecular size distribution of crosslinked polystyrene. Journal of Polymer Science, 11 (6), 513–520. Available from: https://onlinelibrary.wiley.com/doi/abs/https://doi.org/10.1002/pol.1953.120110601.
- Chemistry Libretexts Library, 2017. Bond energies [online]. Available from: https://chem.libretexts.org/Textbook_Maps/Physical_and_Theoretical_Chemistry_Textbook_Maps/Supplemental_Modules_(Physical_and_Theoretical_Chemistry)/Chemical_Bonding/Fundamentals_of_Chemical_Bonding/Bond_Energies [Accessed 2018].
- Cong, P., Xu, P., and Chen, S., 2014. Effects of carbon black on the anti aging, rheological and conductive properties of sbs/asphalt/carbon black composites. Construction and Building Materials, 52, 306–313.
- Cui, H., Yang, J.L., and Liu, Z.Y., 1999. Thermogravimetric analysis of two Chinese used tires. Thermochimica Acta, 333 (2), 173–175. Available from: <Go to ISI>://WOS:000082343900011.
- Daly, W.H., 2017. Relationship between chemical makeup of binders and engineering performance. Washington, DC: Transportation Research Board of the National Academies.
- De Gennes, P.G., 1971. Reptation of a polymer chain in the presence of fixed obstacles. The Journal of Chemical Physics, 55 (2), 572–579.
- De Sousa, F.D.B., et al., 2017. Devulcanization of waste tire rubber by microwaves. Polymer Degradation and Stability, 138, 169–181.
- Dong, D., et al., 2012. Swelling process of rubber in asphalt and its effect on the structure and properties of rubber and asphalt. Construction and Building Materials, 29, 316–322.
- Feng, W., et al., 2015. Investigation of swelling and dissolution process of natural rubber in aromatic oil. China Petroleum and Petrochemical Technology, 17, 76–86.
- Flory, P.J, 1950. Statistical mechanics of swelling of network structures. The Journal of Chemical Physics, 18 (1), 108–111.
- Flory, P.J, 1985. Molecular theory of rubber elasticity. Polymer Journal, 17, 1–12.
- Flory, P.J. and Rehner Jr., J, 1943. Statistical mechanics of cross-linked polymer networks. II. swelling. The Journal of Chemical Physics, 11, 521–526.
- Frantzis, P, 2004. Crumb rubber-bitumen interactions: diffusion of bitumen into rubber. Journal of Materials in Civil Engineering, 16 (4), 387–390.
- Ganji, F., Vasheghani-Farahani, S., and Vasheghani-Farahani, E., 2010. Theoretical description of hydrogel swelling: a review. Iranian Polymer Journal, 19 (5), 375–398.
- Gawel, I., Stepkowski, R., and Czechowski, F., 2006. Molecular interactions between rubber and asphalt. Industrial & Engineering Chemistry Research, 45 (9), 3044–3049. Available from: <Go to ISI>://WOS:000237166800020.
- Ghavibazoo, A. and Abdelrahman, M., 2013. Composition analysis of crumb rubber during interaction with asphalt and effect on properties of binder. International Journal of Pavement Engineering, 14 (5), 517–530. Available from: <Go to ISI>://WOS:000320574900008.
- Ghavibazoo, A., Abdelrahman, M., and Ragab, M., 2013a. Effect of crumb rubber modifier dissolution on storage stability of crumb rubber-modified asphalt. Transportation Research Record: Journal of the Transportation Research Board, 2370, 109–115.
- Ghavibazoo, A., Abdelrahman, M., and Ragab, M., 2013b. Mechanism of crumb rubber modifier dissolution into asphalt matrix and its effect on final physical properties of crumb rubber-modified binder. Transportation Research Record: Journal of the Transportation Research Board, 2370, 92–101. Available from: <Go to ISI>://WOS:000328441200013.
- Ghavibazoo, A., Abdelrahman, M., and Ragab, M., 2015. Evaluation of oxidization of crumb rubber–modified asphalt during short-term aging. Transportation Research Record: Journal of the Transportation Research Board, 2505, 84–91.
- Green, E.L. and Tolonen, W.J., 1977. The chemical and physical properties of asphalt-rubber mixtures, part I – basic material behavior. Phoenix, Arizona, U.S.: Arizona Department of Transportation.
- Hansen, C.M., 2002. Hansen solubility parameters: a user’s handbook. Boca Raton, Florida, US: CRC Press.
- Horikx, M., 1956. Chain scissions in a polymer network. Journal of Polymer Science Part A: Polymer Chemistry, 19 (93), 445–454.
- Huang, S.C., 2008. Rubber concentrations on rheology of aged asphalt binders. Journal of Materials in Civil Engineering, 20 (3), 221–229. Available from: <Go to ISI>://WOS:000253392200004.
- Joseph, A.M., et al., 2016. The current status of sulphur vulcanization and devulcanization chemistry: devulcanization. Rubber Science, 29 (1), 62–100.
- Lee, S.-J., Akisetty, C.K., and Amirkhanian, S.N., 2008. The effect of crumb rubber modifier (CRM) on the performance properties of rubberized binders in hma pavements. Construction and Building Materials, 22 (7), 1368–1376.
- Lee, P.I. and Peppas, N.A., 1987. Prediction of polymer dissolution in swellable controlled-release systems. Journal of Controlled Release, 6 (1), 207–215.
- Lesueur, D., 2009. The colloidal structure of bitumen: consequences on the rheology and on the mechanisms of bitumen modification. Advances in Colloid and Interface Science, 145 (1–2), 42–82. Available from: http://www.ncbi.nlm.nih.gov/pubmed/19012871.
- Liang, M., et al., 2017. Characterization of fume composition and rheological properties of asphalt with crumb rubber activated by microwave and tor. Construction and Building Materials, 154, 310–322.
- Liu, Y. and Shi, B., 2008. Determination of flory interaction parameters between polyimide and organic solvents by hsp theory and igc. Polymer Bulletin, 61 (4), 501–509.
- Lo Presti, D., 2013. Recycled tyre rubber modified bitumens for road asphalt mixtures: a literature review. Construction and Building Materials, 49, 863–881.
- Lo Presti, D. and Airey, G., 2013. Tyre rubber-modified bitumens development: the effect of varying processing conditions. Road Materials and Pavement Design, 14 (4), 888–900.
- Lo Presti, D., Izquierdo, M.A., and Jiménez Del Barco Carrión, A., 2018. Towards storage-stable high-content recycled tyre rubber modified bitumen. Construction and Building Materials, 172, 106–111.
- Luo, R., et al., 2017. Water vapor diffusion in asphalt mixtures under different relative humidity differentials. Construction and Building Materials, 136, 126–138.
- Luo, R. and Huang, T., 2018. Development of a three-dimensional diffusion model for water vapor diffusing into asphalt mixtures. Construction and Building Materials, 179, 526–536.
- Ma, T., et al., 2015. Characteristics of desulfurized rubber asphalt and mixture. KSCE Journal of Civil Engineering, 20 (4), 1347–1355.
- Mark, J.E., 1981. Rubber elasticity. Journal of Chemical Education, 58 (11), 898–903.
- Mark, J.E., 2009. The polymer data handbook (2nd ed). Oxford, UK: Oxford University Press.
- Mark, J.E., Erman, B., and Roland, M., 2013. The science and technology of rubber. Waltham, Massachusetts, USA: Academic Press.
- Medina, J.R. and Underwood, B.S., 2017. Micromechanical shear modulus modeling of activated crumb rubber modified asphalt cements. Construction and Building Materials, 150, 56–65.
- Miller-Chou, B.A. and Koenig, J.L., 2003. A review of polymer dissolution. Progress in Polymer Science, 28 (8), 1223–1270.
- Narasimhan, B., 2001. Mathematical models describing polymer dissolution: consequences for drug delivery. Advanced Drug Delivery Reviews, 48 (2–3), 195–210. Available from: https://www.ncbi.nlm.nih.gov/pubmed/11369082.
- Narasimhan, B. and Peppas, N.A., 1996a. On the importance of chain reptation in models of dissolution of glassy polymers. Macromolecules, 29 (9), 3283–3291. Available from: <Go to ISI>://WOS:A1996UG97300033.
- Narasimhan, B., and Peppas, N.N., 1996b. Disentanglement and reptation during dissolution of rubbery polymers. Journal of Polymer Science Part B: Polymer Physics, 34, 947–961.
- Papanu, J.S., et al., 1989. Transport models for swelling and dissolution of thin polymer-films. Journal of Applied Polymer Science, 38 (5), 859–885. Available from: <Go to ISI>://WOS:A1989AN37100009.
- Peppas, N.A., Wu, J.C., and Vonmeerwall, E.D., 1994. Mathematical-modeling and experimental characterization of polymer dissolution. Macromolecules, 27 (20), 5626–5638. Available from:<Go to ISI>://WOS:A1994PJ32400017.
- Quesada-Pérez, M., et al., 2011. Gel swelling theories: the classical formalism and recent approaches. Soft Matter, 7 (22), 10536.
- Ragab, M., Abdelrahman, M., and Ghavibazoo, A., 2013. Performance enhancement of crumb rubber-modified asphalts through control of the developed internal network structure. Transportation Research Record: Journal of the Transportation Research Board, 2371, 96–104.
- Redelius, P.G., 2000. Solubility parameters and bitumen. Fuel, 79 (1), 27–35. Available from: http://www.sciencedirect.com/science/article/pii/S0016236199001039.
- Redelius, P., 2004. Bitumen solubility model using hansen solubility parameter. Energy & Fuels, 18 (4), 1087–1092.
- Redelius, P. and Soenen, H., 2015. Relation between bitumen chemistry and performance. Fuel, 140, 34–43.
- Rubinstein, M. and Colby, R.H., 2003. Polymer physics. New York: Oxford University press.
- Scott, Ewan, 2016. End-of-life tyre report 2015. Brussels, Belgium: European Tyre and Rubber Manufacturers' Association.
- Shen, J., et al., 2009. Surface area of crumb rubber modifier and its influence on high-temperature viscosity of crm binders. International Journal of Pavement Engineering, 10 (5), 375–381.
- Shen, J. and Amirkhanian, S., 2007. The influence of crumb rubber modifier (CRM) microstructures on the high temperature properties of crm binders. International Journal of Pavement Engineering, 6 (4), 265–271.
- Shu, X. and Huang, B.S., 2014. Recycling of waste tire rubber in asphalt and portland cement concrete: an overview. Construction and Building Materials, 67, 217–224.
- Sienkiewicz, M., et al., 2012. Progress in used tyres management in the European Union: a review. Waste Management, 32 (10), 1742–1751. Available from: https://www.ncbi.nlm.nih.gov/pubmed/22687707.
- State of California Department of Transportation, 2003. Asphalt rubber usage guide. Sacramento, CA, USA: California Department of Transportation.
- Stroup-Gardiner, M., Newcomb, D.E., and Tanquist, B., 1993. Asphalt-rubber interactions. Transportation Research Record, 1417, 99.
- Subhy, A., Lo Presti, D., and Airey, G., 2015. An investigation on using pre-treated tyre rubber as a replacement of synthetic polymers for bitumen modification. Road Materials and Pavement Design, 16, 245–264. Available from: <Go to ISI>://WOS:000355122400015.
- Tang, N., Huang, W., and Xiao, F., 2016. Chemical and rheological investigation of high-cured crumb rubber-modified asphalt. Construction and Building Materials, 123, 847–854.
- Torretta, V., et al., 2015. Treatment and disposal of tyres: two EU approaches. A review. Waste Management, 45, 152–160. Available from: https://www.ncbi.nlm.nih.gov/pubmed/25943287.
- Tu, Y. and Ouano, A.C., 1977. Model for the kinematics of polymer dissolution. IBM Journal of Research and Development, 21 (2), 131–142.
- Vrentas, J.S. and Vrentas, C.M., 1998. Dissolution of rubbery and glassy polymers. Journal of Polymer Science Part B: Polymer Physics, 36 (14), 2607–2614. Available from: <Go to ISI>://WOS:000076115700013.
- Wang, T., et al., 2017b. A review on low temperature performances of rubberized asphalt materials. Construction and Building Materials, 145, 483–505.
- Wang, H., et al., 2018a. Review of warm mix rubberized asphalt concrete: towards a sustainable paving technology. Journal of Cleaner Production, 177, 302–314.
- Wang, H., et al., 2018b. Asphalt-rubber interaction and performance evaluation of rubberised asphalt binders containing non-foaming warm-mix additives. Road Materials and Pavement Design, 1–22.
- Wang, H., et al., 2019a. Numerical investigation of rubber swelling in bitumen. Construction and Building Materials, 214, 506–515.
- Wang, H., et al., 2019c. Characterization of bitumen modified with pyrolytic carbon black from scrap tires. Sustainability, 11 (6), 1–13.
- Wang, H., et al., 2020. Experimental investigation of rubber swelling in bitumen. Transportation Research Record: Journal of the Transportation Research Board, 1–10.
- Wang, H., et al., 2020. Micromechanical modeling of complex shear modulus of crumb rubber modified bitumen. Journal of Materials and Design, 188, 1–12.
- Wang, S., Cheng, D., and Xiao, F., 2017a. Recent developments in the application of chemical approaches to rubberized asphalt. Construction and Building Materials, 131, 101–113.
- Wbcsd, 2010. End-of-life tires: a framework for effective management systems. Geneva, Switzerland: Conches.
- Xu, M., et al., 2015. Novel method to prepare activated crumb rubber used for synthesis of activated crumb rubber modified asphalt. Journal of Materials in Civil Engineering, 27 (5), 1–7.
- Yadollahi, G. and Mollahosseini, H.S., 2011. Improving the performance of crumb rubber bitumen by means of poly phosphoric acid (PPA) and vestenamer additives. Construction and Building Materials, 25 (7), 3108–3116. Available from:<Go to ISI>://WOS:000290081800015.
- Yao, H., Zhou, S., and Wang, S., 2016. Structural evolution of recycled tire rubber in asphalt. Journal of Applied Polymer Science, 133 (6), 1–7.
- Zanzotto, L. and Kennepohl, G., 1996. Development of rubber and asphalt binders by depolymerization and devulcanization of scrap tires in asphalt. Transportation Research Record: Journal of the Transportation Research Board, 1530, 51–58.
- Zhu, J., Balieu, R., and Wang, H., 2019. The use of solubility parameters and free energy theory for phase behaviour of polymer-modified bitumen: A review. Road Materials and Pavement Design, 1–22.