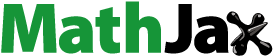
ABSTRACT
Porous friction courses (PFC) are thin layers of asphalt mixtures with open gradations and high air void contents, which are placed over conventional flexible pavements. Although they improve various safety and environmental issues, their main difficulty is their short service life. A common practice to enhance PFC durability is the use of modified asphalt binders (e.g. regular polymer-modified asphalt (PMA) or asphalt rubber). However, this approach has not been enough to achieve life-spans close or equal to those of dense-graded hot mix asphalt. The use of highly polymer-modified asphalts (HiMA), which are binders modified at polymer doses near 7–8% by total weight of the binder, constitutes an alternative to overcome this challenge. This work evaluates the mechanical performance of four PFC mixtures fabricated with a regular polymer modified (PMA) and a HiMA binder, and two aggregate sources. The experimental plan included the semicircular bending (SCB), IDEAL-CT, Cantabro abrasion loss and Tensile Strength Ratio (TSR) tests at intermediate temperatures and various ageing conditions. Results indicate an enhanced cracking and degradation resistance for PFC mixtures containing HiMA and suggest that the use of this type of asphalt binder could increase the service life of these mixtures.
1. Introduction
Porous friction courses (PFC) are thin layers (e.g. 2.5–6.0 cm) placed on top of regular flexible pavement structures to improve the serviceability of roads, mainly regarding traffic safety during rainy events (i.e. enhanced visibility and increased friction), and reduction of the noise produced by the tire-pavement interaction (Huddleston et al. Citation1991; Kandhal Citation2002; Cooley et al. Citation2009; Putman and Kline Citation2012). Unlike conventional dense-graded hot mix asphalt (HMA) mixtures, where the air void (AV) content ranges between 4% and 7%, the AV content in PFC mixtures varies between 18% and 22%. Therefore, these mixtures are highly-permeable, which translates into the superior performance of the material under wet-weather conditions, as water can be quickly removed from the surface of the pavement.
Despite these benefits, there are several unsolved challenges related to the use of PFC mixtures. One of them is their short durability due to ravelling, which is the loss of aggregate particles from the surface of the layers. The reported average durability of PFC mixtures is between 6 and 12 years, which is less than the typical service life of conventional HMA (i.e. 12–18 years), as stated in several studies (Huber Citation2000; Huurman et al. Citation2010; Yildirim et al. Citation2007). The shorter service lives of these materials in comparison to dense-graded HMA imply frequent maintenance and rehabilitation interventions, generating high overall maintenance pavement costs.
Efforts to prevent ravelling include improvements in: (i) the selection of the materials – such as the use of additives in asphalt binders, like polymers, asphalt rubber and anti-stripping agents, and high-quality aggregates; (ii) design and construction practices, (iii) maintenance techniques (Gonzalez et al. Citation2020), and (iv) the identification of suitable project operation-related conditions (e.g. high-speed roads) (Putman and Kline Citation2012; Cooley et al. Citation2000; Hernandez-Saenz et al. Citation2016; Jenks Citation2017; Alfonso et al. Citation2017; Arámbula-Mercado et al. Citation2019). Although these strategies have demonstrated a positive impact on the longevity of PFC mixtures, there is still a need to explore other possibilities to improve durability.
One alternative to increase the performance of asphalt mixtures under demanding loading or weather conditions, which has been gaining popularity, is the use of highly or heavy polymer-modified asphalt binders (HiMA). HiMA binders contain polymer doses between 7% and 8% by weight of binder, which is greater than what is typically used for conventional polymer-modified asphalt (PMA) binders (i.e. polymer dose between 2.5% and 3.5% by weight of binder Airey Citation2004). The increase in the polymer dose induces a predominant phase transition, from the main asphaltene phase to a polymeric phase (Yong et al. Citation2012). As a result, these modified binders have a rubbery network, which typically prompts higher resistance to long-term ageing (Zhu Citation2015; Habbouche et al. Citation2021a), higher viscosity, improved susceptibility to permanent deformation, improved elastic response and better cracking resistance at low and intermediate temperatures (Zhu et al. Citation2014, Greene et al. Citation2014; Blazejowski et al. Citation2015, Yan et al. Citation2020).
Previous research has demonstrated that conventional dense-graded HMA mixtures, Stone Mix Asphalt (SMA) mixtures and Stress Absorbing Membrane Interlayers (SAMI) fabricated with HiMA enhance the mechanical properties of the material (Willis et al. Citation2012; Timm et al. Citation2013; Kluttz Citation2015; Suzuki et al. Citation2010; Greene et al. Citation2014; Quevedo et al. Citation2016; Mazurek and Iwański Citation2017; Gaspar et al. Citation2017). A recent study on the use of HiMA binders in the fabrication of asphalt mixtures in the United States concluded that these mixtures have been used in a variety of applications, including full-depth dense-graded HMA layers and thin overlays under heavy traffic (Habbouche et al. Citation2021b). This study also identified that mixtures with HiMA binders are mainly used in interstate roads or in roads with special loading demands, such as slow-braking loads at intersections. Based on the positive results related to the use of HiMA binders in the variety of asphalt mixture's applications previously described, it is also probable that this type of binder could develop stronger and more durable stone-on-stone contacts in PFC mixtures.
Experiences with the use of HiMA in PFC mixtures, however, are more limited. An extensive literature review on this topic showed that Japan has used HiMA binders produced with up to 15% of styrene–butadiene–styrene (SBS) by weight of binder to overcome major difficulties of PFC mixtures, such as durability and functionality in cold winters, bridge pavements, and manipulation and use of small aggregates (Suzuki et al. Citation2010; Shimeno et al. Citation2010). According to Suzuki et al. (Citation2010), PFC mixtures with asphalt binders produced with 8% polymer by weight of binder endured up to 20 years in-service. Also, Shimeno et al. (Citation2010) reported that the use of a HiMA binder containing 9% SBS with high viscosity was successful in extending the service life of a PFC. These encouraging experiences motivated Jellema and Vonk (Citation2016) to evaluate the functionality and durability of Dutch PFC mixtures with HiMA. The authors concluded that the use of this type of binder improved the functionality of the mixtures and helped to control ageing processes. However, the use of HiMA binders for this application is still not regulated in The Netherlands. Finally, it was also identified that HiMA binders are currently permitted in Germany for the fabrication of PFC mixtures, through the German designation ZTV/TL Asphalt-StB 07 (ZTV-Asphalt StB-Citation07 Citation2007) that regulates the percentage of polymer that can be used by the contractor.
Within this context, it is clear that more research is needed to further evaluate the feasibility of HiMA binders to increase the durability of PFC mixtures. The objective of this study is to perform a comprehensive experimental evaluation of the impact of HiMA binders in key mechanical properties related to the expected durability of PFC mixtures at various ageing conditions. The performance of these mixtures was compared to that of a PFC mixture manufactured with a PMA binder currently used in the state of Florida in the United States. This evaluation included: (1) fracture properties at intermediate temperatures through the Semi-Circular Bending (SCB) tests and the indirect tensile asphalt cracking (IDEAL-CT) test, (2) moisture damage susceptibility through the tensile strength ratio (TSR) specified by the Florida Department of Transportation (FDOT), and (3) durability through the Cantabro abrasion loss test at various standard cycles and ageing conditions.
It is noteworthy that no research effort has previously assessed the durability of PFC mixtures at various ageing conditions and by means of degradation curves obtained from multiple cycles of the Cantabro abrasion loss test. The first aspect is particularly relevant, since it has been proven that temperature and oxidation degrade the polymeric structure of polymer-modified binders (Yan et al. Citation2020) and that asphalt binder oxidation occurs at a higher rate in PFC mixtures due to its open microstructure. In addition, there is no published information on the fracture properties of PFC mixtures fabricated with HiMA binders. Thus, the advanced experimental plan proposed in this work constitutes a novel and relevant contribution to assess the convenience of using HiMA binders to extend the durability of PFC mixtures.
2. Materials and mixture fabrication
Two types of SBS modified binders were used in this study: (i) a conventional PMA binder produced with 2–3% SBS by weight of binder, classified as PG 82-22E, and (ii) a HiMA binder produced with 6–8% SBS by weight of binder, classified as PG 82-28E. Information about the doses used during modification was not provided by the asphalt producers. Specific information about the rheological, chemical and thermodynamic properties of these binders can be found elsewhere (Rivera et al. Citation2021). Additionally, two types of aggregates that are commonly used for the production of PFC mixtures in Florida were selected for this study: limestone and granite.
Four distinct PFC mixtures that comply with FC-5 specification in Florida (USA) (FDOT Citation2018) were produced using combinations of these materials. Two PFC mixtures included PMA or HiMA binder and granite aggregate, and two PFC mixtures were fabricated with PMA or HiMA binder and limestone aggregate. Only virgin materials were used in the fabrication of the PFC mixtures. In terms of anti-stripping additives, the former mixtures included 1.0% of hydrated lime by weight of aggregate, while the latter mixtures included 0.5% by weight of binder of a liquid antistrip (LAS) additive. All mixtures included 0.3% cellulose fibres by weight of mixture, which constitutes a common added material for this type of mixtures. summarises the characteristics of the four PFC mixtures.
Table 1. Characteristics of the PFC mixtures.
The evaluation of ageing effects in the experimental test plan for the PFC mixtures was critical, since oxidative hardening is one of the main causes that diminishes the quality and strength of the stone-on-stone contact network and, consequently, their durability (Lin et al. Citation1995; Alvarez et al. Citation2006; Alvarez et al. Citation2008), and because it has been well-demonstrated that ageing causes polymer degradation in polymer-modified binders (Lu and Isacsson Citation1998; Yan et al. Citation2020). Therefore, this work assessed the response of the four PFC mixtures under both short- and long-ageing conditions.
Short-term ageing was achieved by conditioning the loose mix at 135°C for 2 h before compaction to simulate ageing effects during production and construction, as recommended by the NCHRP project 09-52 (Newcomb et al. Citation2015). These specimens were labelled A0. The procedure to simulate long-term ageing was based on a previous work conducted as part of the NCHRP project 09-54 (Kim et al. Citation2017), which showed that subjecting loose dense-graded asphalt mixtures to oven-ageing at 95°C for 5 days prior to compaction, was equivalent to approximately 2–3 years of field ageing. This ageing state was denoted as A5. Although the equivalence of this ageing process in terms of the actual pavement service life is expected to be different for PFC mixtures, it was still considered representative of some initial service years after A0. For the durability evaluation of the PFCs, which was performed through the Cantabro abrasion loss test, an additional ageing state was included, which consisted in subjecting the loose mix prior to compaction to oven ageing at 95°C for 10 days. This additional ageing state was denoted A10 and could be approximately equivalent to 5 or more years of pavement service life for dense-graded mixtures (Kim et al. Citation2017).
PFC testing samples were fabricated using the Superpave Gyratory Compactor (SGC) at a target AV of 20 ± 1% for all specimens. Compaction was controlled using the weight and expected height of the samples, which values changed depending on the final geometry required for each test. The specimens were confined using 150-mm diameter PVC pipe sleeves to prevent sagging and crumbling, which has been observed in SGC samples with high air void content (Arámbula-Mercado et al. Citation2016). The sleeves were left for a minimum of 12 h during cool down to ensure the integrity of the compacted specimens.
3. Testing plan
lists the tests performed on the PMA and HiMA PFC compacted specimens: SCB, IDEAL-CT, and Cantabro Abrasion Loss (hereafter referred to as Cantabro), and TSR. This table also specifies the testing condition, number of replicates and outcome parameter of each test. The testing plan did not include low-temperature cracking performance tests since this work was limited to the ravelling evaluation of PFCs under the prevalent weather conditions of the State of Florida. A brief description of these tests is presented next.
Table 2. Experimental plan to characterise the PFC mixtures.
3.1. SCB test
The SCB test was performed according to AASHTO TP 124-16 (AASHTO Citation2016). The test specimens were obtained from SGC specimens of 150 mm in diameter and 160 mm in height. A diamond-tipped saw blade with a water-cooling system was used to obtain two smaller cylindrical specimens with a height of 50 ± 1 mm. Afterwards, these specimens were cut in half and a notch 15 ± 1 mm deep and 1.5 ± 0.1 mm wide was introduced in the centre of the flat side of the specimen.
The test consists of placing the planar face of the semi-circular sample on two equally-spaced roller cylindrical supports and applying a monotonic load at a rate of 50 mm/min at 25°C at the top centre of the specimen until fracture. The result of the test is the curve of the applied force versus the vertical displacement of the specimen, also called the vertical load-displacement curve. In this study, the selected output parameters were: (i) the Flexibility Index (FI), which is calculated by dividing the fracture energy or Gf (i.e. total area under the vertical load-displacement curve divided by the ligament area of the fracture specimen) by the slope of the post–peak vertical load-displacement curve (Al-Qadi et al. Citation2015), and (ii) the Cracking Resistance Index (CRI), which is obtained by dividing the Gf computed up to peak load over the magnitude of the peak load (Kaseer et al. Citation2018). Larger values of FI and CRI indicate mixtures with enhanced cracking resistance. These two parameters were selected in this study, as previous works have demonstrated that the speed of crack propagation correlates with the brittleness of the material, and they are able to distinguish cracking susceptibly of mixtures with distinct asphalt binder and other mix characteristics (Al-Qadi et al. Citation2015; Kaseer et al. Citation2018; Zhou et al. Citation2017b).
3.2. IDEAL-CT test
This test consists of applying a monotonic load (P) at a rate of 50 mm/min at 25°C at the top centre of cylindrical specimens of 150 mm in diameter by 50 mm in height using the indirect tensile (IDT) strength test equipment. Although non-standard, the dimensions of the specimens were selected to match those of the SCB specimens. The main advantages of this test are that no instrumentation, cutting, gluing, drilling, or notching of the specimen are required. The resulting parameter is the cracking test index (CTindex), which is calculated through Equation (1). Larger CTindex values are preferred, as they indicate slower cracking growth rates.
(1)
(1) where
is the work of fracture,
is the specimen thickness,
is the slope of the load (P) vs. vertical displacement (l) curve, and
is the ‘strain’ tolerance under loading (i.e. vertical displacement to diameter ratio, D).
The CTindex is computed on the post-peak load section on the load-displacement curve, at a load value that corresponds to 75% of the peak (i.e. post-peak point at 75% or PPP75). Therefore, is considered a ‘modulus’ of the specimen and it is calculated as the absolute value of the load-displacement curve slope between the post-peak points at 85% and 65% of the peak load (PPP85 and PPP65) to minimise variability.
3.2. Cantabro test
Among the existing tests to quantify PFC mixture durability, the Cantabro abrasion or mass loss (AASHTO Citation2014a) has shown to better predict the durability of PFC mixtures when compared to field observations (Alvarez et al. Citation2008; Arámbula-Mercado et al. Citation2016). In this work, a standard and a modified version of the Cantabro test were used to assess the impact of both asphalt binders on the durability of PFC mixtures.
The standard Cantabro test consists of subjecting cylindrical compacted specimens of 150 mm in diameter and at least 114 mm in height to 300 revolutions in the Los Angeles Abrasion test machine without the steel balls at room temperature. The output of the test is the total mass loss of the specimen after one testing cycle (300 revolutions). Larger values of mass loss in this test indicate a higher propensity of the mixture to disintegrate and, therefore, a lower expected durability. In this research work, however, the specimens were further subjected to multiple cycles of 300 revolutions at different ageing conditions. The specimens were weighed after the completion of the first cycle, corresponding to the standard test, the material that detached from the specimen was removed from the drum, and then the specimens were subjected to additional cycles of 300 revolutions. This process was repeated until one of the following two criteria was met: (i) the % mass loss reached 80 ± 2% with respect to the original weight of the specimen, or (ii) a total of 4,500 revolutions were applied (i.e. 15 Cantabro abrasion loss cycles). These two procedures were conducted for the three ageing conditions mentioned in , to assess the influence of ageing on the durability of the four PFC mixtures.
3.4. TSR Test
Moisture susceptibility was evaluated as per FM 1-T 283 test (FDOT Citation2018). In this test, cylindrical specimens of 150 mm in diameter by 50 mm in height were subjected to the IDT test in two states: (i) in ‘dry’ condition (i.e. room condition), and (ii) in ‘wet’ condition, which consisted of applying one freeze–thaw cycle after subjecting the specimens to vacuum saturation during 10 min and leaving the specimen submerged in water, as recommended in AASHTO PP 77-14 (AASHTO Citation2014b). During the freeze cycle used in the moisture conditioning process, the specimens were placed in an environmental chamber at –18 ± 3°C for 18 h. Afterwards, the specimens were subjected to the thaw cycle after submerging them in a water bath at 60 ± 1°C for 24 h. Subsequently, the specimens were placed in water at 25 ± 0.5°C during 2 h prior to testing.
The IDT test consisted of placing the specimen in the loading frame and applying a vertical loading at a rate of 50 mm/min until failure. The resulting parameter is the peak load, which is used to compute the IDT strength through Equation (2).
(2)
(2) where Pmax is the peak load (N), H is the height of the specimen (mm), and D is the diameter of the specimen (mm).
The TSR of the PFC mixtures, which is a measurement of the retained strength of the mixture after moisture conditioning, was computed as the ratio between the wet and the dry IDT strengths. Larger TSR values indicate better resistance of the mixture to moisture damage.
Results and discussion
4.1. SCB test
and present the FI and CRI values obtained from the SCB test for all mixtures, respectively.
These figures show that for a specific asphalt-aggregate combination, ageing negatively affects the fracture resistance of the materials, as reflected by a decrease in both parameters. This is an expected outcome due to the increase in brittleness of the asphalt binders with ageing. Results also show that the HiMA mixtures exhibited better fracture performance than all the PMA mixtures at both ageing conditions, since higher mean values of both cracking parameters were obtained for the HiMA mixtures. For instance, the FI at the A0 condition for the HiMA-granite and HiMA-limestone mixtures were, in average, 2.0 and 5.0 times higher than those for the PMA-granite and PMA-limestone mixtures, respectively. A similar trend was observed at the A5 condition, with the FI for the HiMA-granite and HiMA-limestone mixtures being 2.4 and 3.6 times larger than those of the PMA-granite and PMA-limestone mixtures, respectively.
For the same type of binder, the influence of the aggregate type and ageing state presented mixed results. At A0, the FI values were 1.7 times larger for the HiMA mixtures with limestone than those with granite, while the FI values at A5 were 1.8 times larger with granite than with limestone. Conversely, for the PMA binder, the FI was consistently larger for the granite mixtures than for the limestone mixtures in both ageing states.
Overall, the FI results among replicates were highly variable, particularly for mixtures in unaged state (i.e. COV between 24.7% and 42.3%), and those containing PMA binder (i.e. COV between 30.0% and 42.3%). It should be noted that previous works have already demonstrated that FI is a parameter with high variability in HMA mixtures (Espinosa et al. Citation2020). The results herein obtained, corroborate that high variability in the FI index is also obtained for PFC mixtures. One-way ANOVA tests were performed at a significance level of 5% in order to verify if the differences observed in the average FI values were statistically significant. The selected significance level means that there is a risk of 5% of incorrectly concluding about the existence of differences between different groups of data. The comparison of the FI results for the mixtures at each ageing condition with the same type of aggregate and the two binders (e.g. PMA-limestone vs. HiMA-limestone) resulted in p-values lower than .05 (between 5*10−7 and 2.8*10−5), which corroborated that the differences induced by the type of binder were indeed significant. Regarding the aggregate type, the differences in the FI results were also statistically significant for all cases, except for the mixtures fabricated with PMA in unaged conditions (i.e. PMA-limestone vs. PMA-granite) that presented a p-value of .077.
The CRI results in are in good agreement with the trends observed for FI. Mixtures produced with the HiMA binder exhibited the highest CRI values in both ageing conditions. For instance, the mean CRI values for the HiMA-limestone mixtures in the A0 and A5 conditions were 2.2 and 1.7 times larger than that of the limestone-PMA mixtures, respectively. Similarly, in the A0 and A5 conditions, CRI values for the HiMA-granite mixtures were 1.4 and 1.6 times larger than that of the PMA-granite mixtures. These results corroborate the superior fracture response of the PFC mixtures fabricated with HiMA binder with both types of aggregates. In terms of the aggregate type, HiMA-limestone mixtures exhibited higher CRI values than did the HiMA-granite mixtures in the unaged state, but this condition was reversed at A5 state (i.e. HiMA-granite mixtures exhibited higher CRI). In the PMA mixtures, similarly to what was observed previously for the FI values, PMA-granite mixtures exhibited higher mean values of CRI than those of the PMA-limestone mixtures in both ageing states. It should be also noted that the CRI presented lower variability results among replicates (COV values between 8.7 and 18.7%), as compared to FI results. One-way ANOVA tests verified the statistical differences in the CRI results among mixtures fabricated with HiMA and PMA with the same aggregate source and ageing state (p-values between 1.0*10−7 and 3.2*10−4), and for the mixtures with different sources of aggregates and the same type of binder (p-values between 1.0*10−5 and 7.9*10−3).
Mixed results were also observed when assessing the relative impact of ageing in the reduction of the fracture resistance of the mixtures. Data in , for example, show that the reduction of the mean CRI values between A5 and A0 was 40.0% in the PMA-granite mixtures, while it was 31.8% in the HiMA-granite mixtures. This suggests that ageing had a more negative impact on the fracture resistance of the mixture with PMA. However, the average reduction in CRI between A5 and A0 was larger in the HiMA-limestone mixtures when compared to the PMA-limestone mixtures (i.e. 46.1% versus 54%, respectively). Even though the fracture properties of the mixtures with HiMA and limestone were more severely impacted by ageing than in the mixtures with PMA and limestone, the magnitude of the fracture properties of the HiMA mixtures was higher at each ageing state (i.e. 2.2. and 1.7 times higher), as explained before.
In general, these results are in good agreement with the differences in cracking resistance of dense-graded HMA mixtures fabricated with HiMA binders in comparison to those with regular polymer-modified or -unmodified binders. Chen et al. (Citation2018), for example, assessed the performance of an in-service test road built in 2012 with dense-graded mixtures with three types of binders: (i) unmodified, (ii) SBS regular polymer modified and (iii) SBS highly polymer modified. The evaluation of the road segments demonstrated a superior resistance to cracking of the HiMA mixture and, more importantly, a good correlation between field cracking performance and SCB laboratory results. In a different study, Kwon et al. (Citation2020) performed a full-scale experiment in the Florida Department of Transportation (FDOT)’s accelerated pavement testing (APT) to compare the results of dense-graded asphalt mixtures fabricated with the same type of aggregate but with three different binders: (i) a PMA, (ii) a HiMA binder and (iii) a HiMA binder with an additional 0.5% binder content by total weight of the mixture. The results demonstrated a better performance in terms of fatigue cracking and rutting of the two mixtures with HiMA binders. Similarly, Willis et al. (Citation2012) reported the performance of four road trials tested in National Center for Asphalt Technology (NCAT) Test Track that included a dense-graded surface layer and a dense-graded base layer fabricated with HiMA and with PMA binders. The results show that the mixtures with HiMA binder were more resistant to rutting and fatigue cracking. In fact, the fatigue endurance limit of the base layer with HiMA was three times higher than that in the control PMA base layer. In conclusion, the fracture properties of PFC mixtures herein obtained through SCB testing demonstrate that HiMA binders improve the cracking resistance of these mixtures in a similar way to what has been observed for regular dense-graded HMA mixtures.
4.2. IDEAL-CT test
shows the CTindex results for all mixtures at both ageing states. As mentioned previously, larger values of CTindex indicate slower cracking growth rates and, consequently, better cracking performance.
The results were consistent in both ageing states, and they show that HiMA mixtures had the best performance, similar to what was obtained from the SCB tests. For instance, at A0, the mean CTindex values for HiMA-granite and HiMA-limestone mixtures were 4.6 and 3.9 times larger than those of the PMA-granite and PMA-limestone mixtures, respectively. Similarly, at A5, the average CTindex values for HiMA-granite and HiMA-limestone mixtures were 2.5 and 2.8 times larger than those of the PMA-granite and PMA-limestone mixtures. However, CTindex results among replicates were highly variable, with COV values ranging between 11 and 82%. This variability was larger for the HiMA mixtures, which would imply higher uncertainty in the expected fracture performance of the mixtures. Nevertheless, one-way ANOVA tests confirmed that the CTindex values were statistically different depending on the type of binder, with the exception of the A5 PFC mixture fabricated with limestone (p-value = .247). This result was expected due to the high standard deviation of the CTindex for the HiMA-limestone mixture (i.e. COV 82%).
In terms of the aggregate type, granite mixtures resulted in larger mean values of CTindex than limestone mixtures for both binders and in both ageing states. For example, at A0, the CTindex value for the HiMA-granite mixture was 1.7 times larger than that of the HiMA-limestone mixture, and the CTindex value for the PMA-granite was 1.4 times higher than that of the PMA-limestone mixture. However, one-way ANOVA tests proved that the differences in CTindex of the mixtures fabricated the same type of binder and ageing condition but with different aggregate sources were not significant, as the p-values were .119 and .057 for the PFC mixtures fabricated with PMA and both types of aggregates in the A0 and A5 condition, respectively, and .084 and .256 for those with HiMA and the two aggregates, for both ageing conditions. When comparing the influence of the components of the mixture in the CTindex results, it is then concluded that the type of binder has a more prominent effect on the cracking performance of the PFC mixtures than the aggregate type.
It is worth mentioning that the order of magnitude of the values obtained for the mixtures at A0 were significantly higher than those reported by Zhou et al. (Citation2017a) for dense HMA mixtures. However, the IDEAL-CT test is well known for being sensitive to the AV content (Zhou et al. Citation2017a, Citation2017b). Results for dense HMA mixtures with 9% AV content, for example, had CTindex values that were 1.6 times greater than those for mixtures with 5% AV content (Zhou et al. Citation2017a). Thus, higher CTindex values are expected for the PFC specimens due to their AV content of 20%.
Finally, it should be highlighted that the consistency in the cracking resistance trends observed between the IDEAL-CT and the SCB tests confirms the superior cracking performance of the HiMA PFC mixtures in both ageing conditions. In practical terms, this means that the structural integrity of the stone-on-stone contacts within the PFC mixtures will be more durable in the HiMA mixtures through time and, therefore, that these mixtures will exhibit a higher resistance to ravelling.
4.3. Cantabro test
The previous two sections demonstrated that PFC mixtures fabricated with HiMA were more resistance to cracking. It is then expected that these mixtures will have a better performance in the Cantabro test in comparison to the mixtures fabricated with the PMA binder. summarises the Cantabro results at three ageing states (i.e. A0, A5 and A10). Recalling, mixtures with larger mass loss in this figure are more prone to degradation and, consequently, less durable.
The results demonstrate that the HiMA mixtures were consistently less prone to degradation at all ageing states, as these mixtures had lower values of mass loss. Results also show that ageing considerably affected the performance of all mixtures. The maximum allowable mass loss suggested in AASHTO TP 108 (AASHTO Citation2014a) to assure proper durability of PFC mixtures is 20%. All PFC mixtures passed this requirement in the A0 state, as the mean maximum mass loss was 18.2%, which corresponded to the PMA-granite mixture. However, ageing fostered the degradation of the mixtures, especially for those manufactured with PMA binder. Specifically, the mass loss in the PMA-limestone mixture increased about three times in between ageing states (i.e. A0 to A5 and A5 to A10). These results are in agreement with previous experiences in the state of Florida with PFC mixtures, which indicate that after 5 years of service, some PMA-limestone mixtures experience signs of ravelling (Massahi et al. Citation2018). Conversely, the HiMA binder mixtures passed the standard mass loss requirement in all ageing states. Specifically, the HiMA-granite and HiMA-limestone mixtures in the A5 state presented mean mass loss values of only 10.1% and 8.1%, respectively, and values of 14.7% and 19.0% in A10 state.
In terms of the binder type, the results in show that the mean values of the mass loss of the HiMA mixtures did not reach the 20% threshold at any ageing state, whereas mixtures with PMA binder exceeded this threshold in the A5 and A10 ageing states. Indeed, the 20% mass loss threshold was achieved at the A5 and A10 ageing states for the PMA-limestone and PMA-granite mixtures, respectively. It should be noted, however, that even though the HiMA mixtures in the A10 ageing state was below the 20% limit, the results among replicates had COV values ranging between 28% and 46%, so there is a chance that they could also exceed this limit criterion at this condition. Nevertheless, for all ageing conditions, PMA mixtures exhibited higher mean values of mass loss compared to HiMA mixtures. For example, at A10, the mean mass loss of the PMA-limestone was 3.4 times larger than in the HiMA-limestone mixture. One-way ANOVA tests corroborated that the differences caused by the type of asphalt binder were significant at both ageing conditions, with the exception of the A0 mixtures with granite that resulted in a p-value larger than .05 (p-value = .061).
In terms of the aggregate type, it is observed that the granite mixtures presented smaller mean values of mass loss compared to the limestone mixtures. However, one-way ANOVA tests proved that these differences were not significant (p-values between .082 and .86), with the exception of the mixtures fabricated with both aggregates and PMA in the A10 condition (p-value of 1.25*10−3).
In order to further assess the degradation process of these mixtures, the specimens in different ageing states were subjected to various Cantabro cycles. Results from these tests are shown in . Lines with circle markers in this figure correspond to PFC mixtures with PMA binder, and those with triangles correspond to PFC mixtures with HiMA binder. Different ‘types’ of lines in this figure denote different ageing states. Each ‘Cantabro cycle’ in the horizontal axis corresponds to a set of 300 revolutions. Lower values in the vertical axis of this plot represent mixtures with enhanced durability.
These results show a better performance for the HiMA mixtures. For example, at A0, the mass loss after one Cantabro cycle for the HiMA-granite and HiMA-limestone mixtures was 68% and 46% smaller than when compared to the equivalent PMA mixtures. Besides, in five out of six cases, the PMA mixtures achieved the test stopping criterion of 80% mass loss during the 15 Cantabro cycles; while the HiMA mixtures achieved this condition in more extreme ageing states. Indeed, the less durable HiMA mixture was the HiMA-limestone in the A10 ageing state, which reached the 80% threshold after 10 Cantabro cycles.
Another major finding was the clear effect of ageing in the degradation of the PFC mixtures. The influence of the binder on the resistance to abrasion with ageing progression was significant, since the HiMA-limestone mixtures in the A10 state were able to withstand five more Cantabro cycles until reaching the 80% degradation test stop criterion in comparison to PMA-limestone mixtures at the same ageing state. In accordance to previous results, the limestone mixtures were more susceptible to degradation, in contrast to the granite mixtures. In fact, the target 80% mass loss test stopping criterion was achieved more rapidly for the PMA-limestone mixtures, except for the PMA on the A0 state; and all PMA-limestone mixtures presented a faster mass loss than those with PMA-granite aggregate.
As expected, the Cantabro results are consistent with the fracture properties obtained from the SCB and IDEAL-CT tests, and they confirm that PFC mixtures with HiMA binders can enhance the service-life of these mixtures. Moreover, the Cantabro results are also is good agreement with existing numerical works on the durability of PFC. Specifically, Kluttz et al. (Citation2013) used the rheological properties of regular and highly polymer-modified asphalt binders and the fatigue response of asphalt mortars fabricated with the same binders, to estimate the service-life of PFC mixtures using the Lifetime Optimisation Tool (LOT). This tool evaluates the mechanical results obtained through a finite element (FE) model to estimate the durability of PFC mixtures based on the stress conditions at the stone-on-stone contacts within the microstructure of the material. The results demonstrated that PFC mixtures with HiMA had a meaningful improvement in ravelling resistance, especially under low-temperature conditions (i.e. winter season).
4.4. TSR Test
presents the TSR results for the four PFC mixtures. This figure indicates that the moisture susceptibility of the PFC mixtures changes with time due to ageing. In the original state, the PMA-limestone mixture had the lowest TSR value and, contrary to all other mixtures, it was closed but did not comply with the typical acceptance criterion of a minimum TSR value of 70% (represented with black dotted line in ) based on AASHTO PP 77-14 (AASHTO Citation2014b). At A5, all mixtures satisfied the minimum mean TSR value of 70%, even though some mixtures presented high variability.
shows that the effects of the binder type and ageing in the TSR results were not consistent. For example, the mean value of TSR at A0 was 10% higher in the PMA-granite mixtures when compared to the HiMA-granite mixtures but the opposite occurred with the limestone mixtures, where the mean TSR was higher for the HiMA PFCs. In terms of ageing, the mean value of TSR of the HiMA-granite mixtures in the A5 state increased 6.8% with respect to the A0 state, while the mean TSR of the HiMA-limestone mixtures presented a reduction of 4.9% between both ageing states. In the case of the PMA mixtures, the mean TSR in the granite mixtures exhibited a reduction of 16% between the A0 and A5 states, while the limestone mixtures had an increase in the mean TSR value of 32% between the two ageing states. In terms of the type of aggregate, most limestone mixtures in both ageing states had lower TSR values than the granite mixtures (except for the PMA-granite mixture versus the PMA-limestone mixture in the A5 state), independently of the type of binder.
Since the effects of the asphalt binder or ageing state in the susceptibility to moisture damage of these mixtures were diverse, it is not possible to state a clear influence of the type of binder, aggregate or ageing condition on the expected moisture susceptibility of the mixtures. However, it should be recalled that all mixtures included anti-stripping agents to improve their moisture sensitivity. Therefore, it is possible that the presence of these agents has a more relevant role than a change in the type of asphalt binder. Moreover, the absence of relevant differences in the TSR results for PFC mixtures with HiMA is consistent with the results reported in a previous work for dense-graded mixtures (Willis et al. Citation2012), where it was observed that the use of HiMA binder did not have an impact in the moisture damage susceptibility of two different mixtures when compared to the susceptibility of equivalent regular polymer-modified mixtures. Furthermore, surface free energy (SFE) measurements performed on mastics fabricated with the same set of binders and aggregate as those used in the present study demonstrated that the type of binder had minimal influence in the Energy Ratio, which is a value used to estimate the adhesion quality and durability of asphalt-aggregate interfaces under the presence of moisture (Rivera et al. Citation2021).
4.5. Performance diagrams
To summarise the impact of the binder type on the overall performance of the PFC mixtures, performance diagrams were constructed following the concept used by Rochlani et al. (Citation2019), which was also applied by Rivera et al. (Citation2021), to compare the performance of PMA and HiMA binders. This evaluation was based on six parameters, all of which were described previously:
CRI in unaged (A0) and aged conditions (A5),
CTindex in unaged (A0) and aged conditions (A5),
Cantabro mass loss after one Cantabro cycle in unaged (A0) and the most critical aged condition (A10).
Although the fracture properties of the different PFC mixtures were evaluated through two parameters (i.e. FI and CRI), only the CRI was reported in these diagrams as both parameters exhibited the same tendency in all mixtures and with ageing condition, but CRI had lower variability (i.e. increased reliability). Also, TSR results were not included, as it was not possible to obtain a conclusive trend in the moisture susceptibility among mixtures.
In these diagrams, an improvement in a material property is observed with an increasing distance from the origin or centre of the plot. Thus, the PFC mixture that covers a larger area in the diagram is expected to have a superior overall performance both in terms of cracking resistance, based on the SCB and IDEAL-CT test results, and in terms of durability, based on the Cantabro mass loss results. For the two types of binders herein used to produce PFC mixtures, and show that the PFC mixtures manufactured with HiMA binder covered the largest area within the performance diagram. Hence, HiMA binders constitute a promising alternative to the PMA binders to achieve longer life-spans in PFC mixtures.
5. Conclusions and recommendations
This paper evaluated the role of a HiMA binder on the mechanical and long-term durability of PFC mixtures in flexible pavements and compared these results with a PMA binder commonly used to fabricate these mixtures. In both cases, limestone and granite aggregates were used in the fabrication of the mixtures, for a total of four PFC mixtures. The experimental plan included the evaluation of cracking resistance at intermediate temperatures through SCB and IDEAL-CT tests, durability through Cantabro tests, and moisture susceptibility through TSR tests, at various ageing conditions. Low-temperature cracking was not included in the work plan because the PFC mixtures herein studied are expected to be used in regions with predominant warm-hot weather.
The main conclusions obtained from the work are:
Overall, SCB and IDEAL-CT fracture test results (i.e. FI, CRI and CTindex) demonstrated that the PFC mixtures with HiMA had a superior resistance to fracture than the mixture fabricated with PMA binder at the two ageing conditions evaluated. These results are consistent to what has been already reported in terms of cracking performance in dense-graded mixtures with HiMA binders. In terms of the aggregate source, granite PFC mixtures had a better resistance to fracture than the limestone PFC mixtures for both types of binders.
HiMA mixtures were consistently less prone to degradation, as shown by small percentages of mass loss in the Cantabro test at all ageing conditions and multiple sets of 300 revolution cycles. HiMA mixtures did not surpass the maximum 20% mass loss standard recommendation at the A5 ageing condition, and only the mixture fabricated with limestone could exceed this threshold at the A10 condition. These results, which are consistent to the fracture testing results, suggest that mixtures with HiMA are less prone to ravelling and, consequently, more durable. On the other hand, the differences in mass loss obtained for mixtures with different aggregate sources (granite vs. limestone) were not statistically significant.
In terms of moisture susceptibility, no conclusive results were obtained on the influence of the asphalt binders or the different ageing states on the moisture resistance of the PFC mixtures. However, surface free energy results of the two types of aggregates and of mastics fabricated with the same set of materials as those used in this study, showed similar results, suggesting that the use of HiMA binders does not impact the moisture susceptibility of the mixtures.
Based on the experimental results obtained from this study, it is possible to conclude that PFC mixtures produced with the HiMA binder showed an enhanced long-term performance and a similar durability in terms of moisture damage in comparison to the PFC mixtures produced with the PMA binder, which was consistent in all ageing conditions. Thus, the inclusion of higher dosages of polymer modification (HiMA) constitutes a promising alternative to achieve longer life spans of these mixtures. However, life cycle cost analyses are required to conclude if the enhanced durability of PFC mixtures fabricated with HiMA binders compensates for the initial cost of the binder material.
Finally, the results from this study also highlight the importance of assessing the impact of ageing in the mechanical response of the PFC mixtures, regardless of the binder and aggregate type, especially for cracking or degradation processes. It was clear that ageing fostered the degradation process of the mixtures, especially for those manufactured with PMA binder.
Acknowledgements
The authors would like to acknowledge the financial support provided by FDOT. The authors would also like to thank Mr Greg Sholar for his constant support and feedback in the execution of this project.
Disclosure statement
No potential conflict of interest was reported by the author(s).
Additional information
Funding
References
- AASHTO, 2014a. AASHTO TP 108 -14. Standard method of test for determining the abrasion loss of asphalt mixture specimens. Washington DC: AASHTO.
- AASHTO, 2014b. AASHTO PP 77-14 standard practice for materials selection and mixture design of permeable friction courses (PFCs). Washington DC: AASHTO.
- AASHTO, 2016. Standard method of test for determining the fracture potential of asphalt mixtures using semicircular bend geometry (SCB) at intermediate temperature standard method of test for mixtures using semicircular bend geometry. Washington DC: AASHTO.
- Airey, G. D, 2004. Styrene butadiene styrene polymer modification of road bitumens. Journal of Material Science, 39 (3), 951–959. doi:10.1023/B:JMSC.0000012927.00747.83.
- Al-Qadi, I.L., Ozer, H., Lambros, J., El Khatib, A., Singhvi, P., Khan, T., and Doll, B., 2015, “Testing protocols to ensure performance of high asphalt binder replacement mixes using RAP and RAS,” Urbana-Champaign, IL: Illinois Center for Transportations Series.
- Alfonso, M.L., Dinis-Almeida, M., and Fael, C.S., 2017. Study of the porous asphalt performance with cellulosic fibres. Construction and Building Materials, 135, 104–111. doi:10.1016/j.conbuildmat.2016.12.222.
- Alvarez, A.E., et al., 2006. Report No. FHWA/TxDOT Report 0-5262-1: Synthesis of current practice on the design, construction, and maintenance of porous friction courses. Texas Transportation Institute, Texas A&M University, vol. 7, no. 2, p. 86.
- Alvarez, A.E., et al., 2008. Evaluation of durability tools for porous friction courses. In: 87th Transportation Research Board (TRB) Annual Meeting, Washington, DC (USA). https://trid.trb.org/view/848525.
- Arámbula-Mercado, E., et al., 2016. Understanding mechanisms of raveling to extend open graded friction course (OGFC) service life. Texas A&M Transportation Institute, Final Report No. BDR74-977-04. College Station, TX: Florida Department of Transportation.
- Arámbula-Mercado, E., et al., 2019. Evaluation of FC-5 with PG 76-22 HP to reduce raveling. Texas A&M Transportation Institute, Final Report No. BE287. College Station, TX: Florida Department of Transportation.
- Blazejowski, K., Olszacki, J., and Peciakowski, H., 2015. Highly modified binders orbiton HiMA, ORLEN Asfalt, application guide, version 1e, Poland.
- Chen, J.S., Wang, T.J., and Lee, C., 2018. Evaluation of a highly-modified asphalt binder for field performance. Construction and Building Materials, 171, 539–545. doi:10.1016/j.conbuildmat.2018.03.188.
- Cooley, L.A., et al., 2009. Construction and maintenance practices for permeable friction courses. Washington, DC: The National Academies, NCHRP Report 640 - Project 09-41.
- Cooley, L.A., Brown, R., and Watson, D.E., 2000. Evaluation of open-graded friction course mixtures containing cellulose fibers. Transportation Research Record, 1723 (1), 19–25. doi:10.3141/1723-03
- Espinosa, L., Caro, S., and Wills, J., 2020. Study of the influence of the loading rate on the fracture behaviour of asphalt mixtures and asphalt mortars. Construction and Building Materials, 262, 120037. doi:10.1016/j.conbuildmat.2020.120037.
- FDOT, 2018. Florida method of test for resistance of compacted bituminous mixture to moisture-induced damage. Specification FM 1-T 283. Gainesville, FL: FDOT.
- Gaspar, M.S., et al., 2017. Highly modified asphalt binder for asphalt crack relief Mix. Transportation Research Record, 2630 (1), 110–117. doi:10.3141/2630-14.
- Gonzalez, I.F., et al., 2020. Life extension of porous asphalt pavements through the application of a rejuvenating bituminous emulsion. Proceedings of the 7th E&E Congress EURASPHALT & EUROBITUME, Madrid.
- Greene, J., Chun, S., and Choubane, B., 2014. Evaluation and implementation of a heavy polymer modified asphalt binder through accelerated pavement testing. Gainesville, FL: Florida Department of Transportation (FDOT), Research Report Number FL/DOT/ SMO/14-564.
- Habbouche, J., et al., 2021a. Influence of aging on rheology- and chemistry-based properties of high polymer-modified asphalt binders. International Journal of Pavement Engineering, doi:10.1080/10298436.2021.1890727.
- Habbouche, J., et al., 2021b. State of the practice for high polymer-modified asphalt binders and mixtures. Transportation Research Record, doi:10.1177/0361198121995190.
- Hernandez-Saenz, M.A., et al., 2016. Mix design, performance and maintenance of permeable friction courses (PFC) in the United States: state of the art. Construction and Building Materials, 111, 358–367. doi:10.1016/j.conbuildmat.2016.02.053.
- Huber, G., 2000. Performance survey on open-graded friction course mixes. Synthesis of Highway Practice 284. In: Transportation Research Board (TRB), National Research Council. Washington, DC: The National Academies Press.
- Huddleston, I.J., Zhou, H., and Hicks, R. G., 1991. Performance evaluation of open-graded asphalt concrete mixtures used in Oregon. Journal of the Association of Asphalt Paving Technologists, 60, 19–42.
- Huurman, M., Moore, L., and Woldekidan, M.F., 2010. Porous asphalt raveling in cold weather. Pavement Research Technology, 3, 110–118.
- Jellema, E., and Vonk, W.C., 2016. Improving durability and functionality retention of porous asphalt by using high performing bituminous binders. Proceedings of the 6th Eurasphalt & Eurobitume Congress, Prague, Czech Republic.
- Jenks, C.W., 2017. Final report: performance-based mix design of porous friction course. National Center for Asphalt Technology (NCAT); Auburn University, Report NCHRP 01-55.
- Kandhal P., 2002. Design, construction and maintenance of open-graded asphalt friction courses. National Asphalt Pavement Association (NAPA). Information Series No. 115.
- Kaseer, F., et al., 2018. Development of an index to evaluate the cracking potential of asphalt mixtures using the semi-circular bending test. Construction and Building Materials, 167, 286–298. doi:10.1016/j.conbuildmat.2018.02.014.
- Kim, Y.R., et al., 2017. Long-term aging of asphalt mixtures for performance testing and prediction. Washington, DC: The National Academies, NCHRP Report 871.
- Kluttz, R.Q., et al., 2013. Highly modified bitumen for prevention of winter damage in OGFCs. In: Proceedings of Airfield and Highway Pavements (ASCE conference), Los Angeles, 1075–1087.
- Kluttz, R., 2015. Use of highly polymer modified HMA (HiMA). In: Proceedings of the Ohio Asphalt Paving Conference, Columbus, Ohio.
- Kwon, O., Choubane, B., Green, J., and Sholar, G., 2020. Evaluation of the performance of highly modified asphalt binder using accelerated pavement testing. International Journal of Pavement Engineering. doi:10.1080/10298436.2020.1714617.
- Lin, M.S., Lunsford, K.M., Glover, C.J., Davison, R.R., and Bullin, J.A., 1995. The effects of asphaltenes on the chemical and physical characteristics of asphalt BT - asphaltenes: fundamentals and applications. In: E.Y. Sheu and O. C. Mullins, eds. Boston, MA: Springer US, 155–176.
- Lu, X., and Isacsson, U., 1998. Chemical and rheological evaluation of ageing properties of SBS polymer modified bitumens. Fuel, 77 (9), 961–972. doi:10.1016/S0016-2361(97)00283-4.
- Massahi, A., et al., 2018. Investigation of pavement raveling performance using smartphone. International Journal of Pavement Research Technology, 11 (6), 553–563. doi:10.1016/j.ijprt.2017.11.007.
- Mazurek, G., and Iwański, M., 2017. Relaxation modulus of SMA with polymer modified and highly polymer modified bitumen. Procedia Engineering, 172, 731–738. doi:10.1016/j.proeng.2017.02.093.
- Newcomb, D., et al., 2015. Short-term laboratory conditioning of asphalt mixtures. Washington, DC: The National Academies, NCHRP Report 815.
- Putman, B.J., and Kline, L.C., 2012. Comparison of mix design methods for porous asphalt mixtures. Journal of Materials in Civil Engineering, 24 (11), 1359–1367. doi:10.1061/(ASCE)MT.1943-5533.0000529.
- Quevedo, C., Sanziani, M., Muzzulini, J., Alasia, F., 2016. Modificación de Asfaltos. In: XVII Congreso Argentino de Vialidad y Tránsito, Rosario, Argentina.
- Rivera, C., et al., 2021. Comparative evaluation of ageing effects on the properties of regular and highly polymer modified asphalt binders. Construction and Building Materials, 302, 124163. doi:10.1016/j.conbuildmat.2021.124163.
- Rochlani, M., et al., 2019. Influence of filler properties on the rheological, cryogenic, fatigue and rutting performance of mastics. Construction and Building Materials, 227, 116974. doi:10.1016/j.conbuildmat.2019.116974.
- Shimeno, S., Oi, A., and Tanaka, T., 2010. Evaluation and further development of porous asphalt pavement with 10 years experience in Japanese expressways. In: Proceedings of the 11th International Conference on Asphalt Pavements, Nagoya, Japan.
- Suzuki, T., et al., 2010. Development and study of polymer modified asphalt in Japan. In: Proceedings of the ISAP 11th International Conference on Asphalt Pavements, Nagoya, Japan.
- Timm, D.H., Robbins, M.M., and Willis, J.R., 2013. Field and laboratory study of high-polymer mixtures at the NCAT test track: final report. Auburn, AL: National Center for Asphalt Technology (NCAT), Auburn University, Report 13-03.
- Willis, J.R., et al., 2012. Laboratory evaluation of high polymer plant-produced mixtures. Road Materials and Pavement Design, 13 (Suppl 1), 260–280. doi:10.1080/14680629.2012.657077.
- Yan, C., et al., 2020. Characterizing the SBS polymer degradation within high content polymer modified asphalt using ATR-FTIR. Construction and Building Materials, 233, 117708. doi:10.1016/j.conbuildmat.2019.117708.
- Yildirim, Y., et al., 2007. Winter maintenance issues associated with new generation open-graded friction courses. Center of Transportation Research of the University of Texas at Austin, Rep. No. FHWA/TX-08/0-4834-2.
- Yong, B., et al., 2012. Sustainable transportation systems: plan, design, building management and maintain. Reston, VA: American Society of Civil Engineering (ASCE).
- Zhou, F., et al., 2017a. Development of an IDEAL cracking test for asphalt mix design and QC/QA. Road Materials and Pavement Design, 18 (suppl 4), 405–427. doi:10.1080/14680629.2017.1389082.
- Zhou, F., et al., 2017b. Selection and preliminary evaluation of laboratory cracking tests for routine asphalt mix designs. Road Materials and Pavement Design, 18 (suppl 1), 62–86. doi:10.1080/14680629.2016.1266741.
- Zhu, C., 2015. Evaluation of thermal oxidative aging effect on the rheological performance of modified asphalt binders. Master Thesis, University of Nevada, Reno.
- Zhu, J., Birgisson, B., and Kringos, N., 2014. Polymer modification of bitumen: advances and challenges. European Polymer Journal, 54, 18–38. doi:10.1016/j.eurpolymj.2014.02.005.
- ZTV-Asphalt StB-07, 2007. German designation. Additional technical terms of contract and guidelines for the construction of road surfacing from asphalt. German Roads and Transportation Research Association. The ‘Asphalt constructions’ working group.