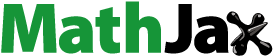
Abstract
A non-targeted method for analyzing petroleum hydrocarbons in soil was established by extracting a wide range of PAC compounds using a model system. Here, sand was spiked with a heavy crude oil, which simulated a hydrocarbon contaminated soil of low soil organic matter (SOM). Soxhlet and supercritical fluid extraction (SFE) was optimized for PAXHs analysis in soil. A concept of using multidimensional ionization for ultrahigh resolution mass spectrometry was employed for detection using electrospray ionization (ESI), atmospheric pressure chemical ionization (APCI) and atmospheric pressure photo ionization (APPI) for the analysis of polycyclic aromatic hydrocarbons (PAHs) and polycyclic aromatic heterocycles. Fourier transform mass spectrometry (FT-MS) with its ultrahigh mass resolution and high mass accuracy enables an unambiguous assignment of the detected ions obtained from different ionization methods, which provides a secure and comprehensive view of contaminants in soil on a molecular level. Over 95% of the spiked crude oil could be recovered by Soxhlet extraction using toluene, dichloromethane and acetone:n-hexane (1:1, v:v). In comparison, when using SFE with supercritical CO2 the recovery was only about 50% but showed more polar compounds. Detailed analyses showed that besides 16 PAHs, a wide range with more than 10,000 PAXHs could also be successfully extracted.
Introduction
Polycyclic aromatic hydrocarbons (PAHs) originated from petrogenic, pyrogenic, or biogenic sources are omnipresent contaminants in soils.Citation1 Alone in Europe over half a million contamination sites have been identified as results of hundred years of industrialization. Annual clean-up cost ranging from 2 to 17 billion were estimated for these contamination sites.Citation2 Among others, PAHs produced from coking and manufactured gas plants are of great concern because of their carcinogenic, mutagenic and ecotoxicological effects.Citation3
PAHs in soil are mostly analyzed in a targeted approach, where only 16 PAHs were selected, which belong to the priority pollutant list from the U.S. Environmental Protection Agency (EPA), and monitored for over four decades.Citation4 Concerns have been arisen for an extension of this list of PAHs, since high-molecular-weight PAHs, alkylated derivatives (alkyl-PAHs) and polycyclic aromatic heterocycles containing N, S, or O (PAXH, X = N, S, O), which co-occur with those 16 PAHs as contaminants and might have higher toxicity, are not included.Citation5
Recently targeted analyses of selected alkyl-PAHs and PAXHs have been reported using a combination of gas chromatography and mass spectrometry (GC-MS).Citation6–8 Inductively coupled plasma mass spectrometry (ICP-MS) is another powerful tool for the quantification of priority compounds or emerging contaminants, such as sulfur compounds in crude oil.Citation9,Citation10 In addition, a non-targeted approach for PAH contaminated soils was enabled by coupling time-of-flight mass spectrometry (ToF MS) with high performance liquid chromatography (HPLC) or two-dimensional gas chromatography (GC × GC).Citation11–13 However, GC related techniques are restricted to volatile and semi-volatile compounds. Furthermore, using HPLC-ToF MS both the separation and mass resolving power could be challenged as the sample complexity and molecular weight of analytes increases.
Fourier transform ion cyclotron resonance (FT-ICR) and Orbitrap mass spectrometry with their ultrahigh mass resolution and mass accuracy ensure an unambiguous assignment of each detected signal in complex mixtures such as crude oil, bio oils, tar oils or lignin derivatives.Citation14–20 A combination of various atmospheric pressure ionization (API) methods, such as electrospray ionization (ESI), atmospheric pressure chemical and photo ionization (APCI/APPI), enables a comprehensive view of the sample.Citation21 Negative mode ESI coupled to FT MS opens the possibility for detailed characterization of acidic compounds especially oxygenated classes. This combination is already applied for the analysis of naphtenic acids from environmental samples,Citation22–24 natural organic matter (NOM),Citation25–28 oil-sand-derived organic material,Citation29 and soil organic matters (SOM).Citation30–32 In contrast to these, basic compounds for instance pyridine-containing molecules are favored under positive mode ESI.Citation33 Both APCI and APPI are suitable for ionizing nonpolar compounds especially PAXHs, which provide complementary data to the ESI measurements for the same sample.Citation21,Citation34
In the environmental analysis sample preparation especially extraction is the crucial step.Citation35 Around the beginning of this century various extraction methods such as Soxhlet extraction,Citation36 mechanic shaking,Citation37 ultrasonic extraction,Citation38 accelerated solvent extractionCitation39 or supercritical fluid extraction (SFE),Citation40 for extracting PAHs from soil were investigated and compared.Citation41–44 Despite the long extraction duration and large solvent consumption, the conventional Soxhlet extraction with its continuous and inexpensive character still serves as a standard method for PAHs extraction.Citation45 Its extraction efficiency was usually compared with other newer techniques. Among them SFE using inert, reusable CO2 with/without addition of few organic solvents as modifier offers a more environmental friendly way of extracting contaminants in soil.Citation46–48
Analyzing different chemical compounds in a matrix such as soil is not easy. Different parameters play a role, such as how the compounds can be fully removed from the soil for a whole determination and characterization. This is followed by answers to the question which analytical method gives the best information. And doing this for an extremely complex analyte mixture such as tar oils makes matters worse. In this study, we perform a non-target analysis of a spiked sand sample with heavy crude oil, which simulates a soil sample contaminated with petroleum hydrocarbons and low SOM to better understand and optimize the extraction procedures and ionization conditions. FT Orbitrap MS coupled with different API methods including ESI, APCI, and APPI in both polarities were applied in order to gain a panorama of the contaminants in the sample. Afterwards the extraction efficiency of Soxhlet extraction with different solvents for petroleum hydrocarbons in this model sample was investigated. Finally, SFE using CO2 was compared to Soxhlet extraction in detail on a molecular level.
Materials and methods
Model sample
Pure sand (50–70 mesh, Sigma-Aldrich, Germany) was spiked with a heavy crude oil (5%, w/w), which was diluted in dichloromethane. Afterwards the remaining dichloromethane was rotary-evaporated to obtain the spiked sand sample.
Sample preparation
The Soxhlet extractions were performed using 5.00 g of spiked sand and additionally 5.00 g of anhydrous sodium sulfate (99.5%, Acros Organics) with 100 mL of toluene (Tol), dichloromethane (DCM), acetone:n-hexane (1:1, v:v, AceHex) or methanol (MeOH) at the boiling point of the solvents for around 300 cycles. All solvents used were HPLC-grade and purchased from Sigma-Aldrich (Germany) or Acros Organics (Germany). The extracts were concentrated in a rotary evaporator and dried under gentle argon stream.
The SFE experiments were performed using a lab-built SFE system (for detailed description see Figure S1). 5.00 g of spiked sand were added into a stainless steel column and heated to 40 °C. Then samples were extracted at 180 bar and 40 °C with supercritical CO2 (99.995%, Air Liquide, Germany) at a flow rate of 2 mL min−1 for 4 h. The SFE extracts were rotary-evaporated and dried under gentle argon stream. The SFE residues were Soxhlet extracted using dichloromethane with the procedure described above.
Mass spectrometric measurements and data analysis
Mass analysis was performed on a research type linear quadrupole ion-trap (LTQ) FT Orbitrap MS (Thermo Fisher, Bremen, Germany) with LTQ Tune Plus 2.7.0 data processing system (Thermo Fisher, Bremen, Germany). The mass spectra were collected from 200 to 1200 Da using the spectral stitching method with 30 Da mass windows with 5 Da overlap.Citation15,Citation49,Citation50 Additionally positive/negative mode ESI or positive mode APCI/APPI with a mass resolution of 960,000 at m/z 400 was applied. For ESI measurements samples were diluted to 250 μg mL−1 in toluene:methanol (1:1, v:v), and injected at a flow rate of 5 μL min−1 with a spray voltage of 4/−3.5 kV for the positive/negative mode. Sheath, auxiliary and sweep gases were set to 5, 2, and 1 arbitrary unit for both polarities. Samples for APCI or APPI were diluted to 250 μg mL−1 in toluene and ionized under the following conditions: flow rate = 20 μL min−1, vaporizer temperature = 350 °C, sheath gas = 20 arbitrary unit, auxiliary gas = 5, and sweep gas = 2. The corona discharge for the APCI was set to 5 μA and a Krypton VUV lamp (10.0 and 10.6 eV, Syagen, Tustin, CA, U.S.A.) was used for the APPI.
Data were exported into Composer64 v1.5.3. (Sierra Analytics, Modesto, CA, U.S.A.) and processed with the following chemical constraints: 0 < C < 200, 0 < H < 1000, 0 < N < 4, 0 < S < 5, 0 < O < 11, 0 < double bond equivalent (DBE) < 11. A maximum mass error of 1 ppm was accepted and only classes with a relative abundance higher than 1% were compared and discussed.
The DBE value for a given elemental composition CcHhNnSsOo was calculated with the following equation:
Results and discussion
Recovery of crude oil in spiked sand
A non-targeted analysis of petroleum hydrocarbons in soil is an adventure due to the high complexity of compounds present in such a sophisticated system. As one of the most important sample preparation steps, the extraction of petroleum based contaminants from soil needs to be investigated in detail.
Therefore, pure sand spiked with a heavy crude oil served as a model sample for a better understanding of the extraction step. Here, we compared the traditional Soxhlet extraction using different solvents with supercritical CO2 extraction. Toluene, dichloromethane and a 1:1 (v:v) mixture of acetone and n-hexane are commonly used solvents for the Soxhlet extraction of 16 EPA PAHs in soils on a routine scale.Citation36,Citation41–44,Citation51
Using these three solvent systems a recovery of over 95% for crude oil in the spiked sand was achieved (see ), where dichloromethane with 97% ± 1% recovery delivered the highest extraction efficiency. Methanol was applied as a mild extraction solvent to study the solubility in polar solvents and therefore, the bioavailability of hydrocarbons in soil.Citation52 Here, a lower recovery of 73% ± 4% compared to other Soxhlet extraction solvents was obtained. This implies the complex character of the heavy crude oil.
Figure 1. Recovery of heavy crude oil in the spiked sand using Soxhlet extraction (in green) with toluene (Tol), dichloromethane (DCM), acetone:n-hexane (1:1, v:v) (AceHex), methanol (MeOH), and supercritical CO2 extraction (extract in blue, residue in brown).
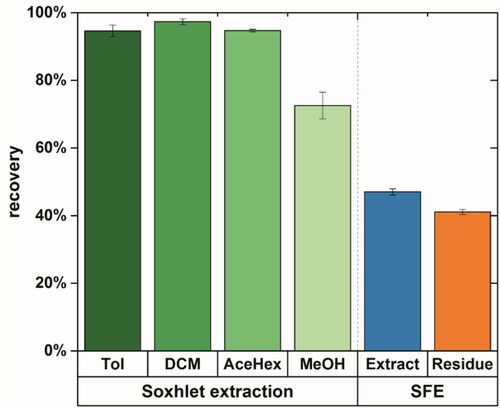
When using supercritical CO2 extraction 47% ± 1% of petroleum components were extracted. Reports in the literature showed a wide range of results for the extraction using SFE. A good recovery of over 80% was reported for lighter jet-diesel oil and fuel oil from spiked sand after the SFE at 65 °C and 400 bar.Citation46 However, Hawthorne and Miller showed that at a comparable extraction temperature of 50 °C and accelerated pressure of 405 bar, lower extraction efficiency was found for higher molecular weight and heteroatom containing PAHs.Citation47 This explained the lower extraction efficiency gained in our study, thus the heavy crude oil contained to a proper portion high molecular weight and heteroatom containing PAXHs.
Although SFE is more environmental friendly and time saving, it cannot extract all contaminants from the soil, especially compounds with higher molecular weight. In contrast to that, the traditionally Soxhlet extraction with dichloromethane offers a simple and feasible way to extract petroleum hydrocarbons from the soil for the non-targeted analysis, albeit the large volume and long extraction time needed.
Mass spectra
Because of the sample complexity, the selectivity of different ionization methods and polarities can be used to provide a comprehensive view of the sample.Citation21 This is presented in the recombined mass spectra, which was recorded using spectral stitching method,Citation15,Citation49,Citation50 for the original crude oil (, left column). For all ionization methods applied ions were detected throughout the whole mass range starting from 200 to 1200 Da. In the zoomed in mass spectra between m/z 400.00 and 400.50 (, right column) up to 42 elemental compositions were detected, which was depended on the selected ionization method. Compounds containing basic nitrogen dominated in the ESI(+) spectrum. Acidic oxygen classes and neutral nitrogen containing components are well ionized in ESI(−).
Figure 2. Recombined (left column) and zoomed in mass spectra at m/z 400 (right column) for the original crude oil sample, analyzed by FT Orbitrap MS using positive mode ESI (red), negative mode ESI (blue), positive mode APCI (green), and APPI (orange), respectively.
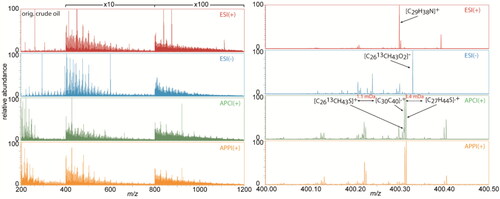
Nonpolar classes such as pure hydrocarbons and sulfur containing PAHs appear in both APCI and APPI measurements. To resolve isobaric peaks of C2613CH43S+ (m/z 400.31135) and C30H40.+ (m/z 400.31245) with a Δm = 0.00110 Da, a resolving power higher than 400,000 is needed. The result shows the necessity of combining different ionization methods with ultrahigh resolution MS for a non-targeted analysis of complex petroleum hydrocarbons.
In , the zoomed in positive mode APPI mass spectra between m/z 400.00 and 400.50 for the original crude oil, Soxhlet extract of the spiked sand using dichloromethane, SFE extract and residue of the spiked sand were compared. In total, 42 elemental compositions were found at this mass range for the original crude oil (Table S1). Here, radical cations of pure hydrocarbons and PASHs dominated the mass spectra. C30H40 and C27H44S with a double bond equivalent (DBE) of 11 and 6 were the first and second highest signal detected in the mass spectra for the original crude oil, Soxhlet and SFE extracts. By decreasing m/z value the DBE value of isobaric pure hydrocarbon ions (C29H52+, C30H40+, C31H28+, C32H16+, highlighted in red from right to left in ), which have a Δm = 0.09390 Da between each other, increases from 4 to 25. The extraction efficiency of SFE decreases the higher the aromaticity is. As can be seen in the spectra, C29H52+ (DBE = 4) and C30H40+ (DBE = 11) were to a great degree extracted using SFE, while C31H28+ and C32H16+ with a DBE of 18 and 25 remained mainly in the residue. This is also applicable for S class compositions shown in .
Figure 3. Zoomed in mass spectra at m/z 400 for the original crude oil (black), Soxhlet extract using dichloromethane (green), SFE extract (blue), and SFE residue (brown) using positive mode APPI FT Orbitrap MS. Some signals are highlighted with calculated elemental compositions and double bond equivalent (DBE) values, separated with a comma.
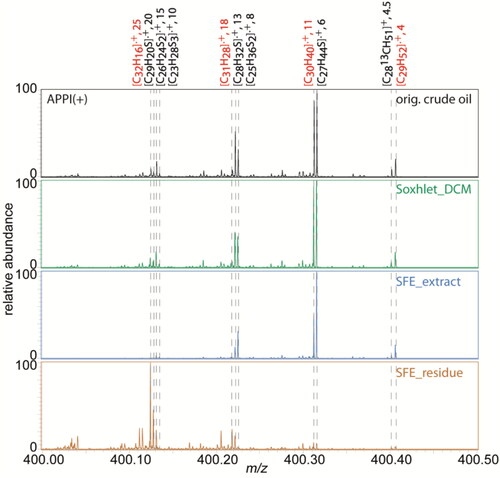
Furthermore, positive and negative mode ESI mass spectra for the original crude oil, the extract and residue after SFE reveal that basic and acidic crude oil related contaminants could also be extracted from the spiked sand (Figures S2–S4 are documented in the supporting information). Compounds with lower DBE were preferentially extracted than compounds with higher DBE. Using API-FT MS a detailed comparison between Soxhlet and SFE on a molecular level can be fulfilled.
Class distribution
Due to the high data load obtained from the non-targeted analysis using ultrahigh resolution FT MS, a suitable, concise and explicit data interpretation is obligatory. This can be done by sorting each signal from the mass spectrum into elemental compositions, which then can be categorized into classes according to their counts of heteroatoms. Different classes such as pure hydrocarbon or heteroatom (N, S, and O) containing classes from different ionization techniques or samples can be compared in a bar chart with their corresponding relative abundances or numbers of assigned compositions.
In , a summary of intensity based class distributions for the Soxhlet extract using dichloromethane is shown. Protonated basic nitrogen containing classes such as N, N2, NS, and NS2 dominate the ESI(+) spectrum with few compounds form radical ions as well as oxygenated sulfur containing class ionized with sodium adduct. In ESI(−) the mass spectrum consists of mainly oxygenated classes especially O2 and O4, which can correspond to molecules contain one or two carboxylic acids.Citation53 Other oxidized heteroatom containing classes such as NOx and SOx were also present with a lower intensity. The selective ionization of acidic components in the sample makes it a perfect method for examining polar transformation products, which are created during the chemical/biological oxidation of PAHs contaminated soil.Citation54–56
Figure 4. Intensity based class distributions for the Soxhlet extracted sample using dichloromethane, analyzed by positive/negative mode ESI, positive mode APCI/APPI (top to bottom) FT Orbitrap MS with protonated/deprotonated molecules in red, radical cations in blue, and molecules with sodium adduct in green.
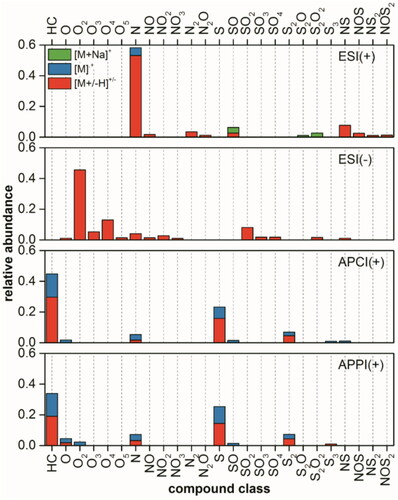
Both APCI and APPI form radical cations [M]+ and protonated molecules [M + H]+ of predominantly nonpolar pure hydrocarbons (HC), Sx, and N classes. In particular APPI by exciting the double bonds in a molecule which suits the best for the targeted and non-targeted analyses of pure PAHs and PAXHs (X = S, N, O).Citation21,Citation57 Results show how complex such a system, which is simulating a petroleum hydrocarbon contaminated soil, can be. Additionally, when analyzing petroleum hydrocarbons in soil in a non-targeted way, ion suppression and discrimination effects can occur.Citation58 This consolidates the need of multidimensional ionization techniques for the deep and comprehensive analysis of petroleum hydrocarbon contaminated soils.
For the targeted analysis, only the 16 EPA PAHs are of interest. But by using positive mode APPI FT MS other high molecular weight and heteroatom containing PAHs present as contaminants can be efficiently ionized and analyzed as well.Citation21 For the original crude oil the highest number of assigned compositions was detected by APPI(+) (25,943), followed by APCI(+) (22,802), ESI(+) (15,264), and finally ESI(−) (12,221) (). Results confirmed the nonpolar character of the spiked contamination source. For a comparison the extraction efficiency of Soxhlet extraction using different solvents and SFE is summarized in . Here, both the relative abundance and the number of assigned compositions for each detected class were considered.
Figure 5. Intensity (top) and population (bottom) based class distributions for the original crude oil, spiked sand Soxhlet extracted using Tol, DCM, AceHex, or MeOH and its SFE extract (SFE E) and residue (SFE R), analyzed by positive mode APPI FT Orbitrap MS. Protonated molecules and radical cations are denoted in red and blue, respectively.
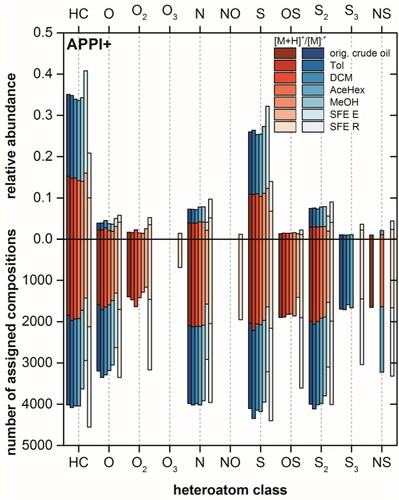
Figure 6. Intensity (top) and population (bottom) based class distributions for the original crude oil, spiked sand Soxhlet extract using Tol, DCM, AceHex, or MeOH and its SFE extract (SFE E) and residue (SFE R), analyzed by positive mode-APCI FT Orbitrap MS.
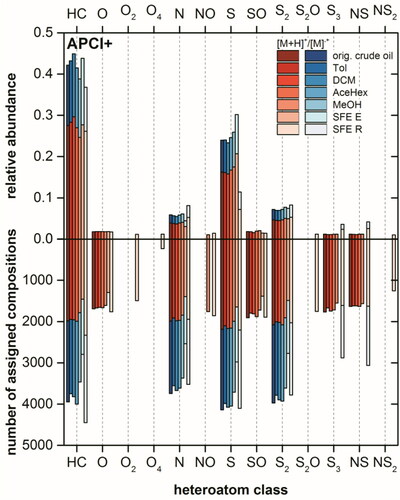
Figure 7. Intensity (top) and population (bottom) based class distributions for the original crude oil, spiked sand Soxhlet extract using Tol, DCM, AceHex, or MeOH and its SFE extract (SFE E) and residue (SFE R), analyzed by positive (left) and negative (right) mode-ESI FT Orbitrap MS. Protonated molecules and radical cations are denoted in red and blue, respectively.
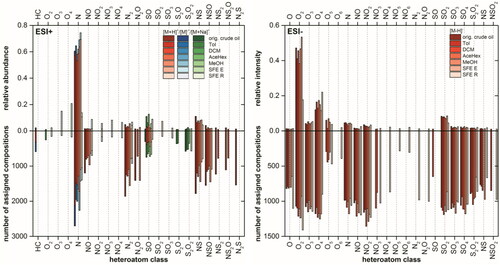
Among these 25,943 assigned compositions by APPI(+) around 4000 were detected as either protonated or radical hydrocarbons in the mass spectrum, which display a relative intensity of around 35%. With an increase in polarity from toluene to methanol, the number of assigned compositions in the HC class decreases from 4082 to 3629. For the polycyclic aromatic heterocycles, such as compounds in S, S2, and N classes, over 95% of the assigned compositions from the original crude oil could be found in the Soxhlet extracts using toluene, dichloromethane, and acetone-n-hexane solvent mixture. For methanol as extraction solvent a recovery of over 90% of the assigned compositions was observed.
In comparison, less than 67% (17,367 out of 25,943 compositions from the original crude oil) were present in the SFE extract, which consisted of predominantly pure hydrocarbons (41%), followed by S (32%), S2 (6%), O (5%), and N (4%) classes. As a result of extraction, the relative intensities of HC and S classes found in the SFE residue decreased dramatically to 21% and 14%, respectively.
However, the numbers of assigned compositions between the original crude oil and SFE residue did not differ too much, which indicates lower extraction efficiency for the complex sample when SFE was applied. The slightly higher amount of HC class compounds and new classes found in the SFE residue could be explained, as there was discrimination and ion suppression occurred during ionization for the original crude oil.Citation58 Additionally, SFE separated the sample into two fractions which led to an increasing analysis depth.Citation59 Similar results were observed by APCI(+) studies (see ). There, compositions from pure hydrocarbon und heteroatom containing classes such as S, S2, and N could be extracted well by Soxhlet extraction using toluene, dichloromethane and acetone/n-hexane solvent mixture. Only a portion (≈70%) of the compositions in the above mentions classes could be extracted using SFE.
Results from APPI(+) and APCI(+) were mutually supportive and implied a better extraction efficiency for nonpolar compounds by Soxhlet extraction using toluene, dichloromethane and acetone-n-hexane solvent mixture than SFE. In ESI(+) the N class with the highest intensity of 60% in the original crude oil consists of mainly basic pyridine-like azaarenes (see , left).Citation13 Out of 2701 assigned compositions as either protonated or radical cations for the N class in original crude oil 1951, 2002, 1569, and 2259 compositions were recovered using toluene, dichloromethane and acetone-n-hexane solvent mixture and methanol, respectively. For the second and third highest intensity classes in the original crude oil, namely NS and N2, same trend was observed. As the most polar solvent investigated methanol could extract over 80% of the assigned compositions for these two classes, whereas for other solvent systems the recovery was between 60% and 70%. The appearance of [SO + Na]+ class with relative high intensity for the Soxhlet extracts was probably a result of sodium salt added for the removal of water.Citation60 Compared to the Soxhlet extraction also a good extraction of polar N class compounds from the spiked sand was achieved when supercritical CO2 was used. Here, a decrease of relative abundance from 74% (SFE E) to 14% (SFE R) was observed.
Compared to ESI(+) with 15,264 assignments, in ESI(−) 12,221 compositions were detected for the original crude oil, which comprise polar compounds containing acidic functional groups (, right). Unlike in the positive mode the assigned compositions in ESI(−) were distributed to O2–4, N0–2, and S2–4 quite equally. A comparable high recovery was achieved by Soxhlet extraction using dichloromethane and methanol. In comparison to this, SFE could only partially extract polar compositions in each class.
These findings obtained from each ionization method showed, Soxhlet extraction is an easy and efficient method, which can be applied prior to the non-targeted analysis of crude oil related contaminants in soil and gives the best representation of the sample.
DBE distribution
The absolute intensity within a given DBE was plotted against compound classes for the APPI(+) measurement of the samples (). The radical cation and protonated molecule classes are denoted as M and M[H], respectively.
Figure 8. Intensity based DBE vs. class distributions for the original crude oil, Soxhlet extract using DCM, SFE extract and residue (from top to bottom) using positive mode APPI FT Orbitrap MS (radical cations: M, protonated molecules: M[H]).
![Figure 8. Intensity based DBE vs. class distributions for the original crude oil, Soxhlet extract using DCM, SFE extract and residue (from top to bottom) using positive mode APPI FT Orbitrap MS (radical cations: M, protonated molecules: M[H]).](/cms/asset/2bc02ea3-1355-4b18-8a18-b7b4b938fbf7/gpol_a_1748665_f0008_c.jpg)
A DBE range of 1–50 was observed for the pure hydrocarbon and heteroatom containing classes, such as S1–3 and N, found in the original crude oil, Soxhlet extract and SFE residue. However, in the SFE extract compounds with DBE only up to 37 were detected. Considering a benzene ring has a DBE value of 4 and by adding another aromatic ring into the system to form naphthalene, anthracene, and so on, the DBE increases by 3 every time with up to 12 aromatic rings. Similar results were observed using APCI(+) as shown in Figure S5. Since predominant compounds with lower DBE could be extracted using SFE, this reduces the sample complexity and increases the intensity of compounds with lower abundance (),Citation59 which explains the additional classes found in the SFE residue.
As summarized in , the mean m/z value for the SFE extract was shifted to the lower mass range compared to the original crude oil sample. Besides, the DBE value decreased from 19 to 15 while H/C increased from 1.23 to 1.38. Accordingly for the SFE residue the mean DBE value increased to 20 while H/C decreased to 1.17. These indicate that SFE favors low aromatized hydrocarbons, which is in agreement with previous studies.Citation40
Table 1. Calculated mean values of m/z, DBE, number of carbon atoms and X/C for the samples, analyzed using APPI(+) FT Orbitrap MS.
Moreover, polar compounds such as basic pyridine-like or neutral pyrrole-like nitrogen containing compounds and oxygenated classes, analyzed using ESI(+/−), could also be extracted with a maximum DBE of 35 (Figures S6 and S7).
DBE vs. carbon count/intensity plot
A closer look into the radical HC class on a molecular level is shown in left column of using the so-called Kendrick plot.Citation14 Here, each detected composition was visualized by its DBE value and number of carbon atoms per molecule. In addition, the total monoisotopic abundance as a sum of all compositions within one given DBE was displayed in line.
Figure 9. DBE vs. carbon count/intensity (Kendrick) plots for the radical HC (left column), [N + H]+ (middle column) and [N − H]− (right column) classes in the original crude oil, Soxhlet extract using DCM, SFE extract and residue (from top to bottom) using positive mode APPI or positive/negative mode ESI FT Orbitrap MS. Upper abscissa for the absolute intensity in a given DBE and lower abscissa for the number of carbon atoms in a assigned composition.
![Figure 9. DBE vs. carbon count/intensity (Kendrick) plots for the radical HC (left column), [N + H]+ (middle column) and [N − H]− (right column) classes in the original crude oil, Soxhlet extract using DCM, SFE extract and residue (from top to bottom) using positive mode APPI or positive/negative mode ESI FT Orbitrap MS. Upper abscissa for the absolute intensity in a given DBE and lower abscissa for the number of carbon atoms in a assigned composition.](/cms/asset/5392abb9-7070-4c79-9f5f-7f2c56854ca0/gpol_a_1748665_f0009_c.jpg)
2107 and 1515 out of in total 2173 radical cations found in the original crude oil could be fully or partially extracted using Soxhlet or SFE, respectively. For the original crude oil, Soxhlet and SFE extracts pure hydrocarbons with DBE of 12 exhibited the highest intensity, which correspond to PAHs containing 4 rings, such as pyrene or fluoranthene with different length of side chains. Instead, in the SFE residue the maximum intensity of DBE shifted to 15, indicating PAHs involving 5 aromatic rings, such as benzo[a]anthracene, benzo[b]fluoranthene or benzo[k]fluoranthene, were dominant in the mass spectrum. Furthermore, the maximum intensity at DBE 15 in the SFE residue (105) was reduced by one order of magnitude compared to the value in the extract (106).
The PASHs in the original crude oil, Soxhlet, and SFE extracts showed a maximum intensity at DBE of 9, which correspond to mostly dibenzothiophene and its alkylated derivatives (Figure S8, left column).Citation61 However, in the SFE residue compounds with DBE = 19 gained the highest intensity. Also for PANHs a shift of the maximum intensity from DBE = 12 to DBE = 17 was observed after SFE (Figure S8, right column). Overall, the results from APPI(+) measurements showed that SFE as compared to Soxhlet extracts only contains nonpolar PAHs and PAXHs with DBE below 35 and carbon number below 90.
As an example for ESI(+) measurements, basic nitrogen containing compounds are shown in the middle column of . From 2134 polar pyridine-like compounds 1538 and 1101 could be extracted using Soxhlet and SFE, which contains mainly 1–5 aromatic rings. However, as the length of alkyl side chains increases, the extraction efficiency decreases. The neutral pyrrole-like nitrogen containing compounds were analyzed using ESI(−) (, right column). Since in the original crude oil N class compounds with only DBE up to 36 were detected, they can all be extracted to a certain extent using both methods. Comparing the SFE extract with its corresponding residue, neutral PANHs could be largely (about 90%) extracted through SFE.
Conclusion
In this work, different organic solvents, which are commonly used for the quantitative analysis of 16 EPA PAHs by Soxhlet extraction, were extended to extract other PAHs and PAXHs containing N or S and their alkylated or oxygenated derivatives. The analysis on a molecular level showed that nonpolar compounds could be extracted quite well by the solvents applied, whereas for polar compounds the sample extracted using dichloromethane and methanol exhibited the highest recovery. Results demonstrated that prior to mass spectrometric analysis the Soxhlet extraction could be used for extracting a large variety of hydrocarbon related contaminants from the soil, which are co-occurring with these 16 PAHs.
Additionally, SFE using supercritical CO2, which is usually referred to as a green extraction method, was studied in detail for extracting petroleum hydrocarbons from spiked sand. Compared to the standard Soxhlet extraction SFE could extract a range of compounds from nonpolar to polar hydrocarbons with a DBE and carbon count lower than 35 and 90, respectively.
In our study, a non-targeted method was developed to examine oil contamination in soil by using ultrahigh resolution mass spectrometry with different ionization methods. A heavy crude soil sample was spiked into pure sand to produce a simple model simulating a hydrocarbon contaminated soil sample with low organic content. Results showed that the selectivity of ionization methods applied offers sometime comparable but more importantly complementary data, each of which reflects only a part of the real sample. Therefore, for the non-targeted analysis of petroleum hydrocarbons in soil, a multidimensional ionization is indispensable. In addition, the coupling to FT MS instrument after ionization ensures a distinct assignment of the signals for such a complex sample.
Supplemental Material
Download PDF (1.4 MB)Acknowledgments
The authors thank Niklas Fuhrmann Max-Planck Institut für Kohlenforschung for assistance with the SFE system. The authors thank Dr. David Stranz (Sierra Analytics, Modesto, CA, USA) for access to new data handling software.
Funding by the ZIM Project KF 3121303SK4 of the Bundesministerium für Wissenschaft und Energie is gratefully acknowledged.
References
- S. A. Stout, S. D. Emsbo-Mattingly, G. S. Douglas, A. D. Uhler, and K. J. McCarthy, “Beyond 16 Priority Pollutant PAHs: A Review of PACs Used in Environmental Forensic Chemistry,” Polycyclic Aromatic Compounds 35, no. 2–4 (2015): 285–315.
- SEC(2006)1165, “Commission staff working document, accompany document to the communication from the commission to the council, the European parliament, the European economic and social committee and the committee of the regions, thematic strategy for soil protection, summary of the impact assessment” (Commission of the European Communities, 2006).
- B. Ranc, P. Faure, V. Croze, and M. O. Simonnot, “Selection of Oxidant Doses for in Situ Chemical Oxidation of Soils Contaminated by Polycyclic Aromatic Hydrocarbons (PAHs): a Review,” Journal of Hazardous Materials 312 (2016): 280–97.
- L. H. Keith, “The Source of U.S.,” Polycyclic Aromatic Compounds 35, no. 2–4 (2015): 147–60.
- J. T. Andersson and C. Achten, “Time to Say Goodbye to the 16 EPA PAHs? Toward an Up-to-Date Use of PACs for Environmental Purposes,” Polycyclic Aromatic Compounds 35, no. 2–4 (2015): 330–54.
- S. Lundstedt, B. A. M. Bandowe, W. Wilcke, E. Boll, J. H. Christensen, J. Vila, M. Grifoll, P. Faure, C. Biache, C. Lorgeoux, et al. “First Intercomparison Study on the Analysis of Oxygenated Polycyclic Aromatic Hydrocarbons (Oxy-PAHs) and Nitrogen Heterocyclic Polycyclic Aromatic Compounds (N-PACs) in Contaminated Soil,” TrAC Trends in Analytical Chemistry 57 (2014): 83–92.
- W. Wilcke, B. A. M. Bandowe, M. G. Lueso, M. Ruppenthal, H. del Valle, and Y. Oelmann, “Polycyclic Aromatic Hydrocarbons (PAHs) and Their Polar Derivatives (Oxygenated PAHs, Azaarenes) in Soils along a Climosequence in Argentina,” Science of the Total Environment 473–474 (2014): 317–25.
- B. A. M. Bandowe, M. G. Lueso, and W. Wilcke, “Oxygenated Polycyclic Aromatic Hydrocarbons and Azaarenes in Urban Soils: A Comparison of a Tropical City (Bangkok) with Two Temperate Cities (Bratislava and Gothenburg),” Chemosphere 107 (2014): 407–14.
- D. Pröfrock and A. Prange, “Inductively Coupled Plasma–Mass Spectrometry (ICP-MS) for Quantitative Analysis in Environmental and Life Sciences: A Review of Challenges,” Applied Spectroscopy 66, no. 8 (2012): 843–68.
- A. Vetere, D. Profrock, and W. Schrader, “Quantitative and Qualitative Analysis of Three Classes of Sulfur Compounds in Crude Oil,” Angewandte Chemie International Edition 56, no. 36 (2017): 10933–37.
- L. Chibwe, C. L. Davie-Martin, M. D. Aitken, E. Hoh, and S. L. M. Simonich, “Identification of Polar Transformation Products and High Molecular Weight Polycyclic Aromatic Hydrocarbons (PAHs) in Contaminated Soil following Bioremediation,” Science of the Total Environment 599–600 (2017): 1099–107.
- C. A. Manzano, C. Marvin, D. Muir, T. Harner, J. Martin, and Y. Zhang, “Heterocyclic Aromatics in Petroleum Coke, Snow, Lake Sediments, and Air Samples from the Athabasca Oil Sands Region,” Environmental Science & Technology 51, no. 10 (2017): 5445–53.
- Z. Tian, J. Vila, H. Wang, W. Bodnar, and M. D. Aitken, “Diversity and Abundance of High-Molecular-Weight Azaarenes in PAH-Contaminated Environmental Samples,” Environmental Science & Technology 51, no. 24 (2017): 14047–54.
- S. K. Panda, J. T. Andersson, and W. Schrader, “Characterization of Supercomplex Crude Oil Mixtures: What is Really in There?,” Angewandte Chemie International Edition 48, no. 10 (2009): 1788–91.
- A. Vetere and W. Schrader, “Mass Spectrometric Coverage of Complex Mixtures: Exploring the Carbon Space of Crude Oil,” ChemistrySelect 2, no. 3 (2017): 849–53.
- D. C. P. Lozano, R. Gavard, J. P. Arenas-Diaz, M. J. Thomas, D. D. Stranz, E. Mejía-Ospino, A. Guzman, S. E. F. Spencer, D. Rossell, M. P. Barrow, et al. “Pushing the Analytical Limits: New Insights into Complex Mixtures Using Mass Spectra Segments of Constant Ultrahigh Resolving Power,” Chemical Science 10, no. 29 (2019): 6966–78. [https://doi.org/10.1039/C9SC02903F].
- B. C. Owen, L. J. Haupert, T. M. Jarrell, C. L. Marcum, T. H. Parsell, M. M. Abu-Omar, J. J. Bozell, S. K. Black, and H. I. Kenttämaa, “High-Performance Liquid Chromatography/High-Resolution Multiple Stage Tandem Mass Spectrometry Using Negative-Ion-Mode Hydroxide-Doped Electrospray Ionization for the Characterization of Lignin Degradation Products,” Analytical Chemistry 84, no. 14 (2012): 6000–07.
- Y. Qi, R. Hempelmann, and D. A. Volmer, “Shedding Light on the Structures of Lignin Compounds: photo-Oxidation under Artificial UV Light and Characterization by High Resolution Mass Spectrometry,” Analytical and Bioanalytical Chemistry 408, no. 28 (2016): 8203–8.
- Y. Qi, R. Luo, W. Schrader, and D. A. Volmer, “Application of Phase Correction to Improve the Characterization of Photooxidation Products of Lignin Using 7 Tesla Fourier-Transform Ion Cyclotron Resonance Mass Spectrometry,” FACETS 2, no. 1 (2017): 461–75.
- C. P. Rüger, M. Sklorz, T. Schwemer, and R. Zimmermann, “Characterisation of Ship Diesel Primary particulate matter at the Molecular Level by Means of Ultra-High-Resolution Mass Spectrometry Coupled to Laser Desorption Ionisation—Comparison of Feed Fuel, Filter Extracts and Direct Particle Measurements,” Analytical and Bioanalytical Chemistry 407, no. 20 (2015): 5923–37.
- A. Gaspar, E. Zellermann, S. Lababidi, J. Reece, and W. Schrader, “Impact of Different Ionization Methods on the Molecular Assignments of Asphaltenes by FT-ICR Mass Spectrometry,” Analytical Chemistry 84, no. 12 (2012): 5257–67.
- J. V. Headley, K. M. Peru, and M. P. Barrow, “Mass Spectrometric Characterization of Naphtenic Acids in Environmental Samples: A Review,” Mass Spectrometry Reviews 28, no. 1 (2009): 121–34.
- J. V. Headley, M. P. Barrow, K. M. Peru, and P. J. Derrick, “Salting-out Effects on the Characterization of Naphthenic Acids from Athabasca Oil Sands Using Electrospray Ionization,” Journal of Environmental Science and Health Part A: Toxic/Hazardous Substances & Environmental Engineering 46, no. 8 (2011): 844–54.
- J. V. Headley, K. M. Peru, and M. P. Barrow, “Advances in Mass Spectrometric Characterization of Naphthenic Acids Fraction Compounds in Oil Sands Environmental Samples and Crude Oil—A Review,” Mass Spectrometry Reviews 35, no. 2 (2016): 311–28.
- T. Reemtsma, A. These, M. Linscheid, J. Leenheer, and A. Spitzy, “Molecular and Structural Characterization of Dissolved Organic Matter from the Deep Ocean by FTICR-MS, Including Hydrophilic Nitrogenous Organic Molecules,” Environmental Science & Technology 42, no. 5 (2008): 1430–07.
- T. Reemtsma, A. These, A. Springer, and M. Linscheid, “Differences in the Molecular Composition of Fulvic Acid Size Fractions Detected by Size-Exclusion Chromatography–On Line Fourier Transform Ion Cyclotron Resonance (FTICR–) Mass Spectrometry,” Water Research 42, no. 1–2 (2008): 63–72.
- T. Reemtsma, “Determination of Molecular Formulas of Natural Organic Matter Molecules by (Ultra-) High-Resolution Mass Spectrometry: Status and Needs,” Journal of Chromatography A 1216, no. 18 (2009): 3687–701.
- J. Raeke, O. J. Lechtenfeld, M. Wagner, P. Herzsprung, and T. Reemtsma, “Selectivity of Solid Phase Extraction of Freshwater Dissolved Organic Matter and Its Effect on Ultrahigh Resolution Mass Spectra,” Environmental Science: Processes & Impacts 18, no. 7 (2016): 918–27.
- M. Noah, S. Poetz, A. Vieth-Hillebrand, and H. Wilkes, “Detection of Residual Oil-Sand-Derived Organic Material in Developing Soils of Reclamation Sites by Ultra-High-Resolution Mass Spectrometry,” Environmental Science & Technology 49, no. 11 (2015): 6466–73.
- J. Guigue, M. Harir, O. Mathieu, M. Lucio, L. Ranjard, J. Lévêque, and P. Schmitt-Kopplin, “Ultrahigh-Resolution FT-ICR Mass Spectrometry for Molecular Characterisation of Pressurised Hot Water-Extractable Organic Matter in Soils,” Biogeochemistry 128, no. 3 (2016): 307–26.
- G. Fleury, M. Del Nero, and R. Barillon, “Effect of Mineral Surface Properties (Alumina, Kaolinite) on the Sorptive Fractionation Mechanisms of Soil Fulvic Acids: Molecular-Scale ESI-MS Studies,” Geochimica et Cosmochimica Acta 196 (2017): 1–17.
- M. M. Tfaily, R. K. Chu, J. Toyoda, N. Tolić, E. W. Robinson, L. Paša-Tolić, and N. J. Hess, “Sequential Extraction Protocol for Organic Matter from Soils and Sediments Using High Resolution Mass Spectrometry,” Analytica Chimica Acta. 972 (2017): 54–61.
- T. Ohno, R. L. Sleighter, and P. G. Hatcher, “Comparative Study of Organic Matter Chemical Characterization Using Negative and Positive Mode Electrospray Ionization Ultrahigh-Resolution Mass Spectrometry,” Analytical and Bioanalytical Chemistry 408, no. 10 (2016): 2497–504.
- C. P. Rüger, T. Miersch, T. Schwemer, M. Sklorz, and R. Zimmermann, “Hyphenation of Thermal Analysis to Ultrahigh-Resolution Mass Spectrometry (Fourier Transform Ion Cyclotron Resonance Mass Spectrometry) Using Atmospheric Pressure Chemical Ionization for Studying Composition and Thermal Degradation of Complex Materials,” Analytical Chemistry 87, no. 13 (2015): 6493–99.
- V. Lopez-Avila, “Sample Preparation for Environmental Analysis,” Critical Reviews in Analytical Chemistry 29, no. 3 (1999): 195–230.
- O. H. J. Szolar, H. Rost, R. Braun, and A. P. Loibner, “Analysis of Polycyclic Aromatic Hydrocarbons in Soil: Minimizing Sample Pretreatment Using Automated Soxhlet with Ethyl Acetate as Extraction Solvent,” Analytical Chemistry 74, no. 10 (2002): 2379–85.
- A. P. Schwab, J. Su, S. Wetzel, S. Pekarek, and M. K. Banks, “Extraction of Petroleum Hydrocarbons from Soil by Mechanical Shaking,” Environmental Science & Technology 33, no. 11 (1999): 1940–05.
- F. Sun, D. Littlejohn, and M. David Gibson, “Ultrasonication Extraction and Solid Phase Extraction Clean-up for Determination of US EPA 16 Priority Pollutant Polycyclic Aromatic Hydrocarbons in Soils by Reversed-Phase Liquid Chromatography with Ultraviolet Absorption detection1,” Analytica Chimica Acta 364, no. 1–3 (1998): 1–11.
- B. E. Richter, B. A. Jones, J. L. Ezzell, N. L. Porter, N. Avdalovic, and C. Pohl, “Accelerated Solvent Extraction: A Technique for Sample Preparation,” Analytical Chemistry 68, no. 6 (1996): 1033–39.
- J. M. Becnel and K. M. Dooley, “Supercritical Fluid Extraction of Polycyclic Aromatic Hydrocarbon Mixtures from Contaminated Soils,” Industrial & Engineering Chemistry Research 37, no. 2 (1998): 584–94.
- Y. F. Song, X. Jing, S. Fleischmann, and B.-M. Wilke, “Comparative Study of Extraction Methods for the Determination of PAHs from Contaminated Soils and Sediments,” Chemosphere 48, no. 9 (2002): 993–1001.
- J. Hollender, B. Koch, C. Lutermann, and W. Dott, “Efficiency of Different Methods and Solvents for the Extraction of Polycyclic Aromatic Hydrocarbons from Soils,” International Journal of Environmental Analytical Chemistry 83, no. 1 (2003): 21–32.
- W. Wang, B. Meng, X. Lu, Y. Liu, and S. Tao, “Extraction of Polycyclic Aromatic Hydrocarbons and Organochlorine Pesticides from Soils: A Comparison between Soxhlet Extraction, Microwave-Assisted Extraction and Accelerated Solvent Extraction Techniques,” Analytica Chimica Acta. 602, no. 2 (2007): 211–22.
- N. Itoh, M. Numata, Y. Aoyagi, and T. Yarita, “Comparison of Low-Level Polycyclic Aromatic Hydrocarbons in Sediment Revealed by Soxhlet Extraction, Microwave-Assisted Extraction, and Pressurized Liquid Extraction,” Analytica Chimica Acta 612, no. 1 (2008): 44–52.
- M. D. Luque de Castro, and L. E. Garcı́a-Ayuso, “Soxhlet Extraction of Solid Materials: An Outdated Technique with a Promising Innovative Future,” Analytica Chimica Acta 369, no. 1–2 (1998): 1–10.
- S. E. Eckert-Tilotta, S. B. Hawthorne, and D. J. Miller, “Supercritical Fluid Extraction with Carbon Dioxide for the Determination of Total Petroleum Hydrocarbons in Soil,” Fuel 72, no. 7 (1993): 1015–23.
- S. B. Hawthorne and D. J. Miller, “Direct Comparison of Soxhlet and Low- and High-Temperature Supercritical CO2 Extraction Efficiencies of Organics from Environmental Solids,” Analytical Chemistry 66, no. 22 (1994): 4005–12.
- M. T. Tena, M. D. Luque de Castro, and M. Valcárcel, “Screening of Polycyclic Aromatic Hydrocarbons in Soil by On-Line Fiber-Optic-Interfaced Supercritical Fluid Extraction Spectrofluorometry,” Analytical Chemistry 68, no. 14 (1996): 2386–91.
- A. D. Southam, T. G. Payne, H. J. Cooper, T. N. Arvanitis, and M. R. Viant, “Dynamic Range and Mass Accuracy of Wide-Scan Direct Infusion Nanoelectrospray Fourier Transform Ion Cyclotron Resonance Mass Spectrometry-Based Metabolomics Increased by the Spectral Stitching Method,” Analytical Chemistry 79, no. 12 (2007): 4595–602.
- A. Gaspar and W. Schrader, “Expanding the Data Depth for the Analysis of Complex Crude Oil Samples by Fourier Transform Ion Cyclotron Resonance Mass Spectrometry Using the Spectral Stitching Method,” Rapid Communications in Mass Spectrometry 26, no. 9 (2012): 1047–52.
- U.S. Environmental Protection Agency (U.S. EPA), SW-846 test methods for evaluating solid waste, Method 3540C: Soxhlet extraction, Washington, DC, USA (1996).
- J. W. Kelsey, B. D. Kottler, and M. Alexander, “Selective Chemical Extractants to Predict Bioavailability of Soil-Aged Organic Chemicals,” Environmental Science & Technology 31, no. 1 (1997): 214–17.
- L. A. Stanford, S. Kim, G. C. Klein, D. F. Smith, R. P. Rodgers, and A. G. Marshall, “Identification of Water-Soluble Heavy Crude Oil Organic-Acids, Bases, and Neutrals by Electrospray Ionization and Field Desorption Ionization Fourier Transform Ion Cyclotron Resonance Mass Spectrometry,” Environmental Science & Technology 41, no. 8 (2007): 2696–702.
- M. Lu, Z. Zhang, W. Qiao, Y. Guan, M. Xiao, and C. Peng, “Removal of Residual Contaminants in Petroleum-Contaminated Soil by Fenton-Like Oxidation,” Journal of Hazardous Materials 179, no. 1–3 (2010): 604–11.
- M. Lu, Z. Zhang, W. Qiao, X. Wei, Y. Guan, Q. Ma, and Y. Guan, “Remediation of Petroleum-Contaminated Soil after Composting by Sequential Treatment with Fenton-like Oxidation and Biodegradation,” Bioresource Technology 101, no. 7 (2010): 2106–13.
- J. Wang, X. Zhang, and G. Li, “Detailed Characterization of Polar Compounds of Residual Oil in Contaminated Soil Revealed by Fourier Transform Ion Cyclotron Resonance Mass Spectrometry,” Chemosphere 85, no. 4 (2011): 609–15.
- S.-S. Cai, J. A. Syage, K. A. Hanold, and M. P. Balogh, “Ultra Performance Liquid Chromatography-Atmospheric Pressure Photoionization-Tandem Mass Spectrometry for High-Sensitivity and High-Throughput Analysis of U.S.,” Analytical Chemistry 81, no. 6 (2009): 2123–28.
- P. Schmitt-Kopplin, M. Englmann, R. Rossello-Mora, R. Schiewek, K. J. Brockmann, T. Benter, and O. J. Schmitz, “Combining chip-ESI with APLI (cESILI) as a Multimode Source for Analysis of Complex Mixtures with Ultrahigh-Resolution Mass Spectrometry,” Analytical and Bioanalytical Chemistry 391, no. 8 (2008): 2803–09.
- A. Gaspar, E. Zellermann, S. Lababidi, J. Reece, and W. Schrader, “Characterization of Saturates, Aromatics, Resins, and Asphaltenes Heavy Crude Oil Fractions by Atmospheric Pressure Laser Ionization Fourier Transform Ion Cyclotron Resonance Mass Spectrometry,” Energy & Fuels 26, no. 6 (2012): 3481–87.
- A. Kruve, K. Kaupmees, J. Liigand, M. Oss, and I. Leito, “Sodium Adduct Formation Efficiency in ESI Source,” Journal of Mass Spectrometry 48, no. 6 (2013): 695–702.
- A. H. Hegazi, E. M. Fathalla, S. K. Panda, W. Schrader, and J. T. Andersson, “High-Molecular Weight Sulfur-Containing Aromatics Refractory to Weathering as Determined by Fourier Transform Ion Cyclotron Resonance Mass Spectrometry,” Chemosphere 89, no. 3 (2012): 205–12.