Abstract
Recent years have witnessed an exponential increase in the research on gold nanoparticles (AuNPs)-based colorimetric sensors to revolutionize point-of-use sensing devices. Hence, this review is compiled focused on current progress in the design and performance parameters of AuNPs-based sensors. The review begins with the characteristics of AuNPs, followed by a brief explanation of synthesis and functionalization methods. Then, the mechanisms of AuNPs-based sensors are comprehensively explained in two broad categories based on the surface plasmon resonance (SPR) characteristics of AuNPs and their peroxidase-like catalytic properties (nanozyme). SPR-based colorimetric sensors further categorize into aggregation, anti-aggregation, etching, growth-mediated, and accumulation-based methods depending on their sensing mechanisms. On the other hand, peroxidase activity-based colorimetric sensors are divided into two methods based on the expression or inhibition of peroxidase-like activity. Next, the analytes in environmental and food samples are classified as inorganic, organic, and biological pollutants, and recent progress in detection of these analytes are reviewed in detail. Finally, conclusions are provided, and future directions are highlighted. Improving the sensitivity, reproducibility, multiplexing capabilities, and cost-effectiveness for colorimetric detection of various analytes in environment and food matrices will have significant impact on fast testing of hazardous substances, hence reducing the pollution load in environment as well as rendering food contamination to ensure food safety.
Graphical Abstract
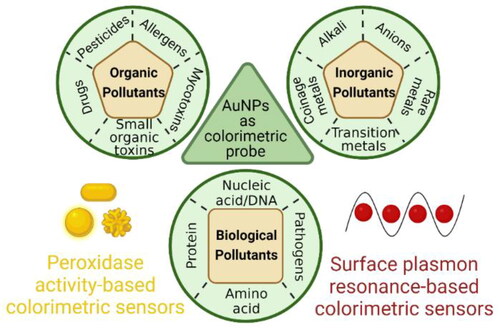
Introduction
Gold nanoparticles (AuNPs) have gained much interest during the past decades, and their applications and characteristics have been investigated in many disciplines, from physics and chemistry to biology and medicine. Historically, the use of AuNPs dates back to the 4th century A.D. when the Romans used them for producing gold ruby glasses, with the Lycurgus cup as a famous example.[Citation1,Citation2] Nonetheless, the modern scientific evaluation of the AuNPs began in 1850s, when Michael Faraday investigated the change in the color of Au particles with respect to their size.[Citation3] Then, through the 20th century, reliable and efficient methods for the synthesis of AuNPs with different characteristics were developed, followed by the upsurge of publications and applications since the beginning of the 21st century.[Citation4–8] AuNPs are usually used in the size range of 2–100 nm and have been developed in several shapes.[Citation6] It is due to their outstanding characteristics such as high surface-to-volume ratio, straightforward synthesis, high stability, unique optical, catalytic, and electrical properties, controllable size, biocompatibility, and versatile surface chemistry amenable to functionalization that they have been used in various applications, including therapeutics, forensics, imaging, and particularly detection.[Citation9–14] displays the timeline of advancements in AuNPs research and applications since Roman times.
Figure 1. Timeline of advancements showing important developments and applications of AuNPs. From left to right, a Lycurgus cup of Roman heritage was created holding Au-Ag NPs to obtain a dichroic effect.[Citation1,Citation2] Then, Michael Faraday made the discovery of gold hydrosols that pushed the gold research first time in 1857.[Citation3] In 1908, Mie theory was discovered as fundamental understanding of the NPs interactions with electromagnetic radiation.[Citation15,Citation16] Afterwards, Turkevich method of AuNPs synthesis in the range of 10-50 nm.[Citation17] In 1960, Feynman mentioned in a famous lecture that “There’s plenty of room at the bottom” meaning at the nanoscale.[Citation18] In 1971, Faulk and Taylor used AuNPs in biomedical field for immunochemical staining.[Citation19] Frens reported an important modification in Turkevich method to achieve wide range 16–147 nm of AuNPs.[Citation20] Then, Brust and Schiffrin had fabricated AuNPs in less than 10 nm in two phases.[Citation21] First report of functionalization of AuNPs as a sensor.[Citation22,Citation23] In 2004, Li and Rothberg reported the detection of complementary DNA (cDNA) using AuNPs.[Citation24] Total 3158 publications had been reported in the detection and sensor field based on Web of Science.
![Figure 1. Timeline of advancements showing important developments and applications of AuNPs. From left to right, a Lycurgus cup of Roman heritage was created holding Au-Ag NPs to obtain a dichroic effect.[Citation1,Citation2] Then, Michael Faraday made the discovery of gold hydrosols that pushed the gold research first time in 1857.[Citation3] In 1908, Mie theory was discovered as fundamental understanding of the NPs interactions with electromagnetic radiation.[Citation15,Citation16] Afterwards, Turkevich method of AuNPs synthesis in the range of 10-50 nm.[Citation17] In 1960, Feynman mentioned in a famous lecture that “There’s plenty of room at the bottom” meaning at the nanoscale.[Citation18] In 1971, Faulk and Taylor used AuNPs in biomedical field for immunochemical staining.[Citation19] Frens reported an important modification in Turkevich method to achieve wide range 16–147 nm of AuNPs.[Citation20] Then, Brust and Schiffrin had fabricated AuNPs in less than 10 nm in two phases.[Citation21] First report of functionalization of AuNPs as a sensor.[Citation22,Citation23] In 2004, Li and Rothberg reported the detection of complementary DNA (cDNA) using AuNPs.[Citation24] Total 3158 publications had been reported in the detection and sensor field based on Web of Science.](/cms/asset/c62e315a-e362-4969-8ace-d79080709aeb/batc_a_2162331_f0001_c.jpg)
Accurate and convenient detection of chemical and biological agents is of high importance for various applications such as food and environmental monitoring, disease diagnostics, and forensics.[Citation12,Citation25–28] Chemical and biochemical sensors have two main components: (1) target molecule recognition which is the specific binding of the sensor reagents to the target analytes and (2) transduction, which is the act of reporting the binding between the sensor reagent and the analyte. Both components are essential in order to have a sensor with high sensitivity and specificity.[Citation29,Citation30] Among various detection techniques, colorimetric sensors have attracted much attention due to their ease-of-use, relative simplicity in design, and allow for equipment-free readout with naked eyes which makes them especially useful for on-site detection.[Citation31] The incorporation of AuNPs in colorimetric sensors further accelerated this area, with AuNPs greatly enhanced signal transducing and opened new gateways for exploration.[Citation29] So far, AuNP-based colorimetric assays have been utilized to detect various types of analytes, for example, small molecules,[Citation32,Citation33] nucleic acids,[Citation34,Citation35] proteins,[Citation36,Citation37] and metal ions.[Citation38–40] Also, these assays have been coupled with convenient device formats such as lateral flow assay, paper-based, and microfluidic devices to enable rapid, portable, and low-cost detection tools.[Citation41–43]
Compared to other metals (e.g. cerium, zinc, and palladium) and polymer nanoparticles (e.g. silica and latex), AuNPs have been the preferred choice for colorimetric applications mainly due to their excellent surface plasmon resonance (SPR), resulting in a characteristic color formation. Also, among other plasmonic materials such as silver and platinum, AuNPs are favored due to various reasons. For example, silver nanoparticles (AgNPs) have high toxicity profile and impart adverse health effects compared to AuNPs.[Citation44] Moreso, AgNPs are light sensitive, and special treatment is required to improve their photostability.[Citation45] Platinum nanoparticles (PtNPs) are usually used as a nanozyme and require a chromogenic agent to produce visible color change.[Citation46,Citation47] Hence, AuNPs have become limelight of research for scientists, researchers, and nanotechnologists. Recent years have witnessed a significant increase in the number of publications related to AuNPs, and many informative reviews have been published discussing colorimetric AuNPs-based sensors.[Citation7,Citation31] A few of them have addressed the use of paper-based microfluidic devices for environmental analysis.[Citation42,Citation48] Nonetheless, rapid progress in this area justifies the need for an updated compilation of information. This state-of-the-art review provides a comprehensive viewpoint on the AuNPs related SPR-based colorimetric sensors and peroxidase activity-based colorimetric sensors in environmental monitoring and food assessment with focus on recent publications. Of course, not all publications can be covered in a single paper, but this review provides an overview of the significant advances in these areas. First, synthetic methods, properties of AuNPs, and mechanisms of colorimetric detection with AuNPs are discussed briefly, followed by in-depth review of the significant publications in environmental and food monitoring. Furthermore, key findings of the references used are summarized in Tables S1–S3 for better comparison of recent colorimetric approaches. Finally, current challenges and future directions are highlighted to point-out less-explored domains of AuNPs-based colorimetric sensors and pave the way for future advancements in this area.
Synthesis and functionalization of AuNPs
Synthesis
Significant efforts have been dedicated over the past half century to develop and understand synthesis strategies for AuNPs. These strategies are divided into two broad categories of top-down and bottom-up, with the latter being the more effective and common one.[Citation49,Citation50] The top-down methods create nanoparticles by removal from bulk gold using techniques such as photolithography,[Citation51] laser ablation,[Citation52] ion sputtering,[Citation53] and aerosol technology.[Citation31,Citation49] Conversely, the bottom-up approaches create AuNPs by reducing the Au ions into Au atoms and subsequently clustering the atoms into nanoparticles, followed by a stabilization step done by capping agents which hinder the agglomeration of the nanoparticles.[Citation31,Citation49] The bottom-up approaches mainly divided into the two categories of chemical and green methods.[Citation31]
Of note, special focus has been on understanding the relations between different strategies and their parameters such as temperature, concentrations, and pH with the characteristics of the resultant AuNPs including size, shape, stability, absorption maximum, and applications to enable controllable synthesis of AuNPs.[Citation7,Citation31] So far, AuNPs in the size range of ∼1.8–190 nm can be readily synthesized, and the shapes include nanosphere, nanorod, nanostar, nanoshell, and nanocage.[Citation6,Citation31] represents various shapes, sizes, colors, functional ligands, stabilization forces, synthetic approaches, and sensor types of AuNPs. For colorimetric detection, the mostly used AuNPs are nanospheres, with the chemical methods being the most used ones for the synthesis.[Citation6]
Figure 2. An overview of the AuNPs profile highlighting their main characteristics. (A) Representation of different shapes, size, and color of AuNPs; (B) surface functionalization of AuNPs with a variety of ligands having different chemical groups; (C) different types of stabilizing forces and surface charge around AuNPs; (D) synthetic approaches into top-down and bottom-up categories for the formation of AuNPs; (E) the main categories of AuNPs-based sensors.
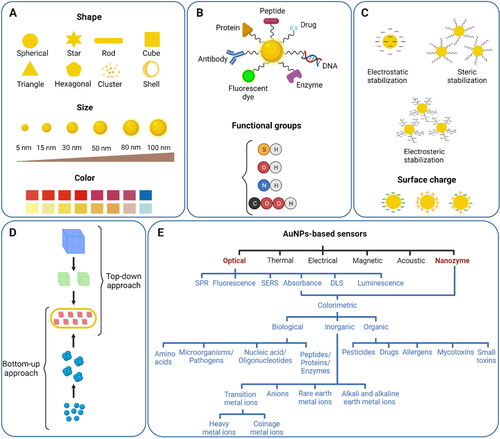
Chemical synthesis of AuNPs
In chemical synthesis, reactions occur in an aqueous or organic medium in the presence of a chemical reducing agent. The most common chemical synthesis method is the Turkevich-Frens method owing to its relative simplicity, controllable size, stability of the prepared colloidal AuNPs, as well as the relatively loose coating of citrates on AuNPs that can be easily replaced by other ligands with desired functionality.[Citation31] Other common methods include the Brust–Schiffrin method and seed-mediated growth. These methods are reviewed in detail elsewhere[Citation6] and are briefly discussed here.
The Turkevich–Frens method, also called sodium citrate reduction method or classical citrate method, was originally developed by Turkevich in 1951 based on many reported studies.[Citation7,Citation17] The basic principle of this method involves reduction of Au ions (Au3+) in chloroauric acid into Au atoms (Au0) by a reducing and capping agent, such as citrate, in reactions that undergo a series of color change from light yellow to ruby red.[Citation7,Citation54] However, the spherical AuNPs prepared by this method had a narrow size range of 10–50 nm, which limited their applications.[Citation17] To solve this issue, in 1973, Frens changed the ratio of sodium citrate and gold chloride and achieved a size range of 16–147 nm by different ratios, after which the Turkevich–Frens method was established and used extensively in various applications.[Citation20] Since then, variations of the method have been reported, including changes in the pH, reducing agents, and stabilizing agents to get varying sizes and stabilities.[Citation6,Citation7] While the Turkevich–Frens method is fairly reproducible and is commonly used, it has its own limitations, including the fact that the size range of stable spherical AuNPs is 10–50 nm beyond which the nanoparticles will aggregate and become less spherical, the susceptibility to environmental conditions such as pH, ionic strength, and salt concentration depending on the stabilizing agent used, and relatively low yield.[Citation7]
The Brust–Schiffrin method was first introduced in 1994 and involves a two-phase liquid–liquid reaction inspired by the Faraday’s two-phase system.[Citation21] In this procedure, the AuCl4 is transferred to the organic phase (i.e. toluene) by a phase-transfer reagent such as tetraoctylammonium bromide (TOAB) from its aqueous solution, followed by reduction using sodium borohydride (NaBH4) in the presence of alkanethiol as the stabilizing agent.[Citation55] The reaction mixture goes from orange to deep brown upon the addition of NaBH4, and the synthesized AuNPs are spherical in the size range of 1.5–5.2 nm.[Citation7] The size of the prepared AuNPs can be controlled by reaction conditions such as temperature, thiol/gold ratio, and reaction rate.[Citation7] Also, several modifications of the Brust–Shiffrin method have been introduced using different thiol containing molecules.[Citation56–59] Of note, one of the main advantages of this method is the direct synthesis of thiol-functionalized AuNPs, which have many applications in sensors area. Other advantages include thermal and air-stability as well as dispersibility in organic solvents without irreversible aggregation.[Citation7,Citation60] Nonetheless, the Brust–Schiffrin method is prone to limitations such as less dispersibility of the prepared AuNPs and the use of organic solvents in the process which may limit the use of AuNPs in some biological applications.
Another common method for the synthesis of AuNPs is the seed-mediated growth which became more common after reported by Wiesner et al. in 1989 in a procedure similar to the Faraday’s original colloidal synthesis method.[Citation61] While the seed-mediated growth is the most common method for the synthesis of rod-shaped AuNPs, spherical AuNPs are also reported, with a size range of 1.5–86 nm.[Citation6] In this procedure, first, gold salts are reduced by a strong reducing agent such as citrate or NaBH4, resulting in small size AuNPs that act as seeds for the fabrication of larger AuNPs. Then, a solution of AuNPs is added to the mixture in the presence of a weak reducing agent such as ascorbic acid, followed by addition of a structure directing agent that prevents further nucleation and enhances the growth of the AuNPs. Overall, since this method uses step-by-step increase of the size, it allows for the controllable synthesis of AuNPs with desired shape and size. Nonetheless, the relatively large number of influential parameters makes the process difficult to control and optimize, including temperature, reducing agent and precursor concentrations, seed concentration, and the rate of the addition of the reducing agent.[Citation7]
Altogether, chemical methods are the most common methods for the synthesis of AuNPs due to their simplicity, ease-of-operation, and allowing for various shapes and sizes of AuNPs. Of note, in addition to the methods mentioned above, many other approaches have been reported in the literature using precursors other than chloroauric acid, various stabilizing reagents and techniques, and different synthesis procedures.[Citation6]
Green synthesis of AuNPs
In order to produce biocompatible AuNPs in eco-friendly processes, green synthesis methods have emerged which aim to reduce or eliminate the harsh chemicals used and produced in the synthesis of AuNPs. These procedures are divided into the two broad categories of physical and biological methods.
Physical techniques utilize the energy of electromagnetic or acoustic waves as well as particle-like radiation to induce chemical reactions in the solution, leading to the reduction of Au ions without the need for chemical reducing agents [Citation7]. These techniques include gamma and X-ray radiations techniques,[Citation62–67] microwave-assisted methods,[Citation68–73] ultraviolet (UV),[Citation74] laser ablation,[Citation75] ultrasonic,[Citation76,Citation77] and electron beam irradiation[Citation78] and encompass methodologies such as photochemical, thermolytic, and sonochemical procedures. The gamma irradiation allows for controllable synthesis of AuNPs, with spherical and nanorod AuNPs in the size range of 2–22 nm been reported. Photochemistry is a relatively simple method that uses a light source like sun or UV light to produce radicals in the system that act as the reducing agent to form AuNPs, while the whole reaction can occur within seconds to few minutes.[Citation74,Citation79] Microwave-assisted methods produce heat in the system that leads to reduction, and the procedure is rapid.[Citation68–70] Overall, the physical methods allow for synthesis of AuNPs with controllable size and structure with reduced time and the possibility of simultaneous synthesis and sterilization of the products. Nonetheless, they have limitations such as low availability of some of the technologies such as X-rays and gamma irradiators, as well as susceptibility of some of the materials to the radiations, especially capping and stabilizing reagents.
Biosynthesis of AuNPs has gained much interest as a cost-effective green method that applies plant-based compounds and extracts (e.g. leaf, flower, and root),[Citation31,Citation80,Citation81] microorganisms (e.g. bacteria, fungi, and algae),[Citation31] and biomolecules (e.g. proteins, amino acids, carbohydrates)[Citation82–84] in the production of AuNPs. Using plant-based compounds is a facile method for producing various sizes of AuNPs (2–300 nm) that requires no additional stabilizers and capping agents.[Citation31] Synthesis with microorganisms can be carried out via intracellular and extracellular methods, but the latter one is favored as it requires less purification steps and hence takes less time and effort. Also, the AuNPs produced with microorganisms showed less cytotoxicity on human cells compared to those of the typical synthesis methods.[Citation85] In addition, studies have shown that AuNPs can be synthesized with biomolecules, including proteins (e.g. sulfite reductase),[Citation83] amino acids (e.g. histidine),[Citation84] and carbohydrates (e.g. dextrose).[Citation82] Of note, one possible issue that may occur when using microorganisms is the improper mixing of the solution that can lead to heterogenous results and products, thus should be carefully controlled.[Citation7] Also, the mechanisms of biological synthesis are not fully understood, possibly due to a large number of interacting molecules, and more research is required to enable more efficient controllable synthesis by biological means.
In conclusion, both chemical and green synthesis methods have their own pros and cons that need to be considered with respect to the required application.[Citation86] The chemical methods offer more flexibility in AuNPs surface chemistry and are simple, fast, and high yielded. Also, the AuNPs produced by chemical methods have reduced dispersity and high thermal stability. Nonetheless, the toxicity of the chemicals used in the chemical synthesis processes or the by-products of the reactions may have detrimental effects on the environment and living organisms, raising concerns for their large-scale production and limiting their use in biological applications, respectively.[Citation7] On the other hand, green route is beneficial due to being cost-effective, sustainable, nontoxic, and eco-friendly which produces less pollution and is considered safer for human health, making them suitable for biomedical applications. However, regarding the biosynthesis of AuNPs, plant extracts have little diversity in functional groups resulting in a restricted choice of surface functionalities around AuNPs. Moreover, the procedure is often time-consuming due to issues related to the extraction of raw materials, often low yielded and produces less uniform particle size due to complexity of natural reducing agents.[Citation86] Of note, green methods related to physical techniques produce uniform morphology of AuNPs with high-speed preparation processes and purity, but such procedures may require bulky equipment that are expensive.
Functionalization
While there is no precise definition of the term “functionalization” in the literature, functionalization in the case of AuNPs is usually referred to anchoring molecules to the AuNPs with specific properties and functions in addition to simple stabilization.[Citation87] Regarding sensors and biosensors, this specific function is typically the facilitation of a specific response to the target analytes in order to provide high selectivity and sensitivity. Historically, the functionalization of AuNPs for sensor applications was first reported in 1996, when two research groups functionalized AuNPs with thiolated oligonucleotides and used them for the detection of DNAs.[Citation22,Citation23] So far, various categorizations have been proposed to classify functionalization of AuNPs based on the type of the binding between the functionalization agents and AuNPs, the procedure of functionalization, and the arrangement of functionalization agents on the surface of AuNPs as shown in .
Functionalizing agents can bind to the surface of AuNPs via physical adsorption or covalent binding. Physical adsorption is based on the forces such as electrostatic interaction between the oppositely charged molecules and van der Waals effects, and it is also referred to as physisorption or electrostatic adsorption.[Citation88] For example, unfolded single-stranded DNAs (ssDNAs)[Citation24,Citation89] and streptavidin[Citation90] were bound to the citrate-capped AuNPs via electrostatic forces and used for the detection of different analytes. Both, neutral ligands (e.g. Tween 80, Tween 20, and poly (ethylene glycol)) and charged ligands (e.g. citrate, phosphine, and borohydride) can be used with this technique[Citation88,Citation91–93] While physical adsorption process is rapid and relatively simple, physical bindings suffer from limitations such as susceptibility to the environmental conditions (e.g. pH and ionic strength) as well as lack of proper orientation of ligands onto AuNPs which makes biological processes difficult to handle. Covalent binding, on the other hand, is the most widely used approach and provides high stability for AuNPs against different salt concentrations, thermal treatment, and attacks by other ligands. Covalent coupling is mainly based on the strong bonding between the S and Au atoms, and thus, many thiol and sulfur containing/modified molecules have been studied for binding onto AuNPs, including alkane thiolates,[Citation21] glutathione,[Citation94] thioethers,[Citation95] disulfide,[Citation96] xanthates,[Citation97] and DNAs and aptamers.[Citation98,Citation99] Amino acids, peptides, and proteins can also bind to the AuNPs via the thiol group on the cysteine amino acid or N-terminal primary amine.[Citation88,Citation100] In case of amino acid functionalized AuNPs, the presence of amino and carboxylic functionalities in their structure make them good cross-linkers for aggregation purposes.[Citation101] Hence, the amino acid modified AuNPs act as a recognition probe for a variety of analytes detection due to the presence of electrostatic interactions between analyte and carboxylate group of amino acid.[Citation102] Additionally, these probes or small peptide modified AuNPs have been used for the detection of some amino acids for instance arginine, histidine, lysine, aspartic acid, and cysteine.[Citation101]
Regarding the procedure of functionalization, functionalization can either be performed simultaneously with the synthesis process or as another step after the synthesis. For the former one, various approaches can be adopted, including using only functionalization agents in the solution as well as a mixture of functionalization agents and stabilization agents.[Citation87,Citation103] Post-synthesis functionalization can be realized by the two approaches of ligand replacement of the stabilization agent with the functionalization agent or reaction onto the surface of AuNPs. Ligand exchange of the citrate-capped AuNPs with amine or thiol groups is relatively easy due to the weak binding of citrate to the AuNPs and can be done under the ambient conditions.[Citation104] Nonetheless, the ligand replacement of thiol bound AuNPs with other thiol-containing molecules is more challenging and is typically done in a solvent such as toluene in ambient condition over several days.[Citation88,Citation104] Also, various techniques have been used to functionalize AuNPs by reaction on the surface of AuNPs as a platform. These techniques include “click” chemistry, which typically refers to Husigen 1,3-dipolar cycloaddition of azides and terminal amides,[Citation88,Citation105] and polymerization procedures such as living radical polymerization (LRP)[Citation106] and surface-initiated atom-transfer radical polymerization (SI-ATRP).[Citation30,Citation107]
For certain applications, AuNPs may need to be co-functionalized with several ligands each with different functionality. For example, AuNPs co-functionalized with poly (ethylene glycol)(PEG) and peptides,[Citation108] and PEG and fluorescent dyes[Citation109] have been reported for in vivo applications, showing high stability and circulation time inside the body. There are three types of co-functionalization according to the arrangement of the ligands on the surface of AuNPs, including mixed monolayers, co-functionalization by means of a linker molecule, and the combination of both, with the mixed monolayers being the most common one.[Citation88,Citation104] Mixed monolayer coatings can be created by first replacement of the citrate on the citrate-capped AuNPs with a certain amount of thiolated PEG to produce partially coated AuNPs, and then reacting the prepared AuNPs with another ligand. In the co-functionalization by using a linker, the AuNPs are first fully coated with a polymer such as PEG and the other ligand is bound to the other end of the polymer chain.
Of note, among various functionalizing agents, intensive research has been done on DNA or aptamer functionalization on AuNPs due to their high binding affinity toward analytes (low detection limit) and high specificity.[Citation110] Also, as DNAs and aptamers are programable entities, they allow AuNPs to be organized uniformly into one-, two- or three-dimensional arrays and superstructures.[Citation111] Different strategies have opted to conjugate DNAs onto AuNPs following either physical adsorption or covalent binding.[Citation112] Physical adsorption takes place either directly or through biomolecule promoted non-covalent binding. Direct physical adsorption is influenced by the natural affinity between nucleobases and AuNPs irrespective of the presence of thiol group. The binding strength of DNA bases to AuNPs via keto and imino groups are in the order of adenine > cytosine ≅ guanine > thymine.[Citation113] This binding affinity helps to regulate/tune DNA density on AuNPs surface by controlling the amount of a specific nucleobase in an engineered DNA structure.[Citation114] Of note, adsorption affinity of adenine base is weaker as compared to thiol moiety. Also, highly specific biomolecular interactions are involved in non-covalent binding of biotinylated DNA and protein-capped AuNPs (e.g. streptavidin or avidin). The streptavidin or avidin has four biotin-binding sites resulting attachment of more than one DNA per protein. On the other side, covalent binding takes place either by chemisorption or ligand-mediated covalent bonding. Commonly used thiolated DNAs have disulfide bond which is reduced to two thiol groups before conjugation.[Citation115] Ligand-mediated covalent bonding is a post-synthesis functionalization and reaction that takes place on the surface of AuNPs by cross-linking between two compatible functional groups of AuNPs and DNA. For example, EDC coupling has been used between an amine and carboxylic groups to form an amide bond as well as click chemistry-based on azide and alkyne groups to form triazole linkage.[Citation112] In short, a wide range of engineered DNAs have been adsorbed onto AuNPs which have significantly advanced the biosensing technology.
Since the DNA functionalization onto AuNPs dramatically affects the colloidal stability, it is highly important to strategically control the density of DNA onto Au surface for various applications. In this regard, many researchers have presented landmark works to solve many issues such as time-consuming preparation process, adsorption of DNAs in a quantitative manner, low density, and limited stability. Mixing of AuNPs with excessive thiolated DNAs cannot yield highly dense DNA surrounded AuNPs as electrostatic repulsion due to DNA phosphate backbones hinder this simple procedure. This challenge is solved by “salt-aging” method which utilizes slow addition of concentrated salt to effectively screen the charge of phosphate backbones in adsorbed thiolated DNAs on AuNPs.[Citation116] However, the salt-aging process for getting highly dense DNA onto Au surface is tedious and time-taking which is replaced with other methods that involve the addition of stabilizing surfactants or polymers, providing acidic environment, or freezing approach. Also, as hybridization between DNA-AuNPs and complementary DNA-AuNPs results in uncontrollable nanoparticles network structure, precise control of DNA strands on Au surface is required which is achieved by getting low density of DNA onto AuNPs. Low density can be achieved by synthesizing discrete dimeric, trimeric, or multimeric AuNPs by controlling stoichiometric ratio.[Citation112] Nonetheless, low grafting density of DNA onto Au surface is challenging with bigger size of AuNPs due to their less colloidal stability. Hence, efficient passivation with other ligands is used on AuNPs surface for low density of DNA strands. In short, conjugation of thiolated DNAs onto AuNPs can be achieved instantaneously (a few minutes) and controllably when considering DNA parameters (e.g. length, sequence, and functional groups) and AuNPs features (e.g. size, shape) in addition to immobilization strategy. Modified DNA bases or backbone with random DNA libraries adsorbed onto AuNPs can be a promising candidate for the detection of new analytes.
Overall, a plethora of methods and ligands have been proposed for the functionalization of AuNPs. Whether which one to use depends on the target analytes, detection mechanism, and the media/matrix and conditions that the sensor is intended to be applied. Of note, functionalization agents should provide sufficient stability for the modified AuNPs against agglomeration as often the stabilization agent is replaced with the functionalization agents. Also, a proper functionalization strategy should be adopted such that it does not alter the shape and size of the prepared AuNPs.[Citation87]
Characterization of AuNPs
After synthesis and functionalization, AuNPs need to be characterized from different aspects to understand whether the synthesis and functionalization have been conducted correctly, or whether the procedures need to be modified and optimized according to the desired properties. The common characteristics of AuNPs include size, size distribution, shape and morphology, surface charge, crystallinity, and surface chemistry. There are various tools and techniques to characterize AuNPs each with their own applications, and often a combination of these techniques is required to comprehensively understand the properties of the AuNPs.[Citation117]
One of the most common methods to get a basic understanding of the nanoparticles is transmission electron microscopy (TEM), which provides images of AuNPs and information about the size, and shape, size distribution and morphology of the particles.[Citation118] Scanning electron microscopy (SEM)[Citation119] and atomic force microscopy (AFM)[Citation120,Citation121] are other imagery techniques used. Dynamic light scattering can also be used to determine the size distribution of AuNPs.[Citation122] Another conventional method is ultraviolet–visible (UV–vis) spectrophotometry, which is usually used to investigate the formation and aggregation of the AuNPs.[Citation118] Near-infrared (NIR) spectroscopy is also reported to investigate AuNP aggregates and different shapes of AuNPs.[Citation123] To understand the surface chemistry of the prepared AuNPs, Fourier-transform infrared spectroscopy (FT-IR) is an effective method that provides information about the functional groups present on the AuNPs.[Citation118] Gel electrophoresis is another technique that is mainly used to confirm the successful attachment of the oligonucleotides/polymers onto the surface of AuNPs through separating nano-conjugates based on their size and charge.[Citation124,Citation125] Other applications such as monitoring the surface density of the attached polymers has also been reported.[Citation126] Another useful technique is measuring zeta potential of the colloidal solution whicht is the amount of the electric charge on the surface of AuNPs and is used for the estimation of the stability of the particles.[Citation127,Citation128] Higher level of stability of colloidal suspension of nanoparticle depends on the value of zeta potential ranging from −25 to +25 mV. Other methods used include X-ray diffraction (XRD),[Citation129] energy-dispersive X-ray (EDX),[Citation130] surface-enhanced Raman spectroscopy (SERS),[Citation131] single particle inductively coupled plasma mass spectrometry (SP-ICP-MS),[Citation132] proton nuclear magnetic resonance (H-NMR) spectroscopy,[Citation133] and laser desorption/ionization time-of-flight (LDI-TOF) mass spectrometry.[Citation134]
Mechanisms of AuNPs-based colorimetric sensors
In colorimetric devices, AuNPs can be used in two different ways. These particles are used as the transducer to produce the detectable signal in SPR-based colorimetric sensors while a capping agent is used as the sensing element. However, AuNPs act as a catalyst to facilitate the reaction between chromogenic substance (transducer) and targeted analyte in peroxidase activity-based colorimetric sensors. The chemistry of molecular recognition step in colorimetric sensors determines the category of detection mechanism.
Mechanisms of AuNPs-related SPR-based colorimetric sensors
In nano-optics community, AuNPs are recognized as noble nanoplasmon due to free electron gas density around the particle which offers limited motion because of their small size as compared to adequate motion of free electrons in case of bulk material. That is why, optical properties are significant in nanoscale material while electrical properties are considerate in bulk metals. The optical properties arise from their size confinement effect produce new electronic properties resulting a distinct feature of vibrant color of their colloidal solution.[Citation135] The principle behind color change of AuNPs is based on SPR phenomena as shown in . Strong optical absorption in the visible range happened by the collective excitation of free electron cloud when Au nanoparticles are exposed to the light irradiation. The oscillation of the electron gas relative to the Au nuclei take place because of two opposite events; (i) the displacement of the electron cloud relative to the Au nuclear framework due to the electric field of light (ii) a reinstating force arises from Coulomb attraction between electrons and nuclei. For a typical spherical AuNP with a size of 13 nm, the maximum light absorption occurs at the wavelength of 520 nm, which is also responsible for the wine-red color of the solution. Nonetheless, the absorption spectrum is dependent on the size, shape, solvent, coating, temperature, and the proximity of other nanoparticles.[Citation30,Citation87] For example, increasing the diameter of spherical AuNPs resulted in the shift toward longer wavelengths (red shift).[Citation136] Also, forming anisotropic forms of AuNPs such as nanorods, stars, and shells resulted in additional peaks in the NIR region, which makes them useful for in vivo imaging and analysis.[Citation123,Citation137] Of note, aggregation of AuNPs result in a strong red shift (i.e. from ∼520 to ∼650 nm) and a significant color change from red to blue,[Citation30,Citation118] which has been the foundation of various AuNP-based colorimetric assays.
Figure 3. Principle of surface plasmon resonance-based colorimetric sensor due to surface plasmon resonance (SPR) phenomena which is the collective oscillation of the Au electrons in resonance with the electromagnetic field in the presence of light irradiation. (A) No SPR happens when particle size is greater than the wavelength of light; (B) SPR phenomena takes place successfully when wavelength of light is greater than AuNPs size to get a colorimetric response.
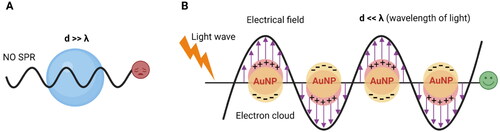
The surface plasmon resonance characteristic of AuNPs used in colorimetric sensing is categorized into five approaches based on the nature of mechanisms involved behind detection. These mechanisms include aggregation, anti-aggregation, etching, growth and accumulation of AuNPs.[Citation138] Aggregation-based colorimetric detection is the most popular method where aggregation of nanoparticles leads to surface plasmon band toward a longer wavelength, resulting in the color change from red to blue. Mainly, two kinds of aggregation behavior exist: non-crosslinking aggregation and cross-linking aggregation. Non-crosslinking aggregation is a widely used mechanism due to its operational simplicity, well-defined sensing guidelines and no need of interparticle bond formation which can be explained by the Derjaguin–Landau–Verwey–Overbeck (DLVO) theory.[Citation139,Citation140] In this case, van der Waals attractive forces between particles become greater than electrostatic repulsive interactions, and hence, aggregation is promoted as shown in . This interparticle aggregation can be induced either using salt or analyte that has higher affinity toward the stabilizer as compared to the stabilizer and AuNPs interactions. However, in some cases, especially with aptamers as the stabilizer, the analyte cannot release the stabilizer from AuNPs and thus modified procedures and conditions are required for biosensing applications.[Citation141,Citation142] The degree of aggregation can be determined according to the absorbance ratio between aggregated and dispersed nanoparticles or blue/red ratio.
Figure 4. Representation of some common mechanisms in AuNPs-related surface plasmon resonance-based colorimetric sensors. A(i–iii) Non-crosslinking aggregation mechanisms involving removal of surface stabilization around nanoparticles whereas A(iv–vi) cross-linking aggregation strategies promote intermolecular bond formation between ligand-functionalized AuNPs and analyte; B(i) anti-aggregation method based on analyte triggered switch of linker molecule from active to suppressed state that promotes the dispersed state of AuNPs whereas B(ii) dispersion of AuNPs happen in the presence of analyte without participating any linker molecule; B(iii) nano-affinity assisted assay utilizing target-specific aptamer to form target-aptamer complex for anti-aggregation-based detection, whereas in the presence of non-binding DNA/aptamer sequence, the target analyte induces aggregation of AuNPs.; C(i–iv) etching-based mechanisms in the presence of an analyte showing morphological changes in shape and/or size of AuNPs by the action of etchants to stimulate colorimetric plasmonic response.
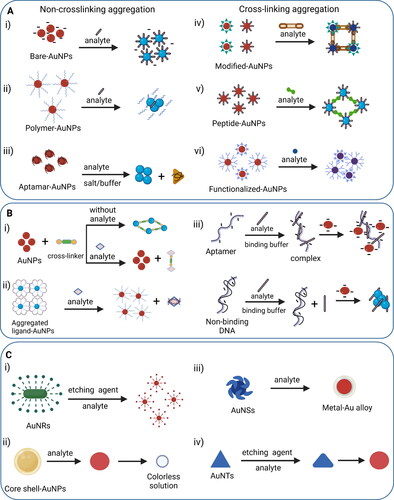
Cross-linking aggregation can happen through direct interactions or requires a cross-linker. In the case of a cross-linker, aggregation is promoted when the analyte possesses at least two binding sites, so interparticle attractive forces dominate over interparticle repulsive forces, resulting in a bond formation either through hydrogen bonding, metal-ligand coordination, electrostatic attraction or hydrophobic interactions as shown in . Common examples of interactions are streptavidin–biotin, aptamer–target, lectin–sugar, antibody–antigen, and DNA hybridization. However, mostly an anti-receptor or a complementary unit is already attached to AuNPs which favors chemical binding between compatible groups adsorbed on AuNPs to promote aggregation when no crosslinker is involved as shown in .[Citation139] A representative example of cross-linking aggregation is azide-AuNPs and alkyne-AuNPs based click chemistry that leads to triazole formation in the presence of copper ions.[Citation143]
Of note, a higher degree of stabilization is achieved when functionality is anchored to AuNPs through covalent linkages. In this case, dispersion/aggregation behavior is flexible to control as compared to the system where functionality is immobilized on AuNPs via electrostatic interactions.[Citation144] The color of the colloidal particle changes from red to purple/blue when the inter-particle spacing between the AuNPs is 2.5 times shorter than the average particle diameter.[Citation145] As a result, the aggregated signal is recognized in the detection zone of a sensor as an “on” output in terms of blue color. This color shift is remarkable due to the extinction coefficients which are three to five orders of magnitude larger than normal organic dyes.[Citation146] Sometimes, the addition of salt (e.g. chloride, sulfate, thiosulfate, and carbonate) becomes essential to improve the sensitivity of the sensor through enhanced aggregation by minimizing the interparticle electrostatic repulsion force.[Citation147,Citation148] Of note, non-crosslinking aggregation occurs quickly in a few minutes as compared to cross-linking aggregation which might require hours. In fact, relatively slow Brownian motion and strict temperature control required for reactions on colloidal surface make cross-linking aggregation based colorimetric detection less common.
AuNPs-based colorimetric sensors employing anti-aggregation mechanism utilize either crosslinker-based method or nano-affinity assisted assay. When a crosslinker is used as a main component of the detection system, linker molecules such as DNA, buffer, or any small unit build preexisting interactions with AuNPs so the probe is aggregated without the presence of an analyte as shown in .[Citation149] However, the active site of the crosslinker is suppressed in the presence of an analyte which stimulates the dispersion of AuNPs, resulting in a characteristic red color as a signal output. The key point of this mechanism is based on analyte prompted shift of crosslinker from a functional state to a suppressed one. On the other hand, nano-affinity assisted assay utilizes target-aptamer binding-inhibited AuNPs aggregation (anti-aggregation) instead of binding-promoted AuNPs aggregation. Initially, positively charged, or neutral target protein is mixed with either a non-binding DNA sequence or a protein-specific aptamer. The AuNPs rapidly aggregate with proteins in the presence of non-binding DNA sequences due to negative charge of nanoparticles and positive charge of protein leading to a color change from red to blue. However, protein incubation with protein-specific aptamers generates aptamer-protein complexes which have a more negative charge leading to strong electrostatic repulsion between aptamer-protein complex and AuNPs resulting in inhibition of AuNPs aggregation (anti-aggregation) and retaining dispersed state of AuNPs as shown in .[Citation150] The key point of this assay is to maintain the pH of a detection system at less or near to the isoelectric point of protein because charge on protein in AuNPs solution is pH-dependant. A variety of metal ions,[Citation151,Citation152] nonmetal ions,[Citation118,Citation153,Citation154] and amino acid[Citation155] have been detected using anti-aggregation mechanism of modified AuNPs. Conclusively, anti-aggregation based detection is more selective compared to the aggregation based detection system due to utilizing specific interactions between AuNPs probe and analytes of interest. Moreover, anti-aggregation based detection system involves a multi-step procedure, whereas aggregation-based assay is a single step detection.
Besides stability of AuNPs, morphology changes also play an important role to develop colorimetric sensors. Certain chemical reactions happen on the surface of AuNPs that induce morphology alterations which result in a colorimetric signal generation for analyte detection.[Citation156] Different shapes of AuNPs such as nanospheres, nanorods (AuNRs), nanotriangles (AuNTs), nanostars (AuNSs), and nanourchins have shown etching-based mechanism for colorimetric sensing, but AuNRs are the representative example in this category due to their high surface energy. The etching reaction involves the use of etchants such as H2O2, I−, and Cu2+ ions that reduce the aspect ratio by etching the terminal ends of AuNRs resulting in a change in shape and size of the nanoparticles as shown in .[Citation157] These morphological changes shift LSPR peak with a visible color change read-out. Sometimes, ligand molecules or analytes facilitate etching process due to their high redox potential. For example, the electron injection from the ligand to the AuNPs is the mechanism of ligand-induced etching of AuNPs.[Citation158] Sometimes, ligand-induced etching is referred as target-mediated direct etching because analyte behaves as ligand to decrease the redox potential of nanoparticles which promotes etching. Bimetallic core–shell AuNPs is the suitable example of this mechanism where outer metallic shell is etched first by analyte and dissolved oxygen producing red color solution and finally Au core is etched giving colorless solution as shown in . This detection method shows higher sensitivity due to emphasized SPR feature of bimetallic alloy. Other common approaches in etching-based sensing mode involve alloy-promoted etching, intermediate-mediated etching such as enzyme or inhibitor mediated etching which changes the morphology and color of AuNPs in the presence of analyte as shown in .[Citation158]
In summary, etching is a non-aggregation based colorimetric method which does not require labeling protocols. This detection mode is favored over aggregation-based sensors due to having no false positive results because of auto-aggregation of nanoparticles. However, the presence of oxidizing agent, acidic condition, longer detection time, and relatively high temperature requirements limit the application of etching-based detection.
Surface plasmon resonance-based colorimetric sensors also follow growth-based and accumulation-based mechanisms for colorimetric detection. The growth-based sensors are mainly divided into seed-mediated or target-mediated growth either by chemical transformation or enzymatic action. Seed-mediated growth assay is a direct reducing-target induced growth system where catalytic seeds of gold are involved to adsorb analyte on their surface, so growth of small sized AuNPs is promoted. As a result, cluster aggregation of AuNPs takes place by chemical transformation which triggers a wavelength shift and color change in the solution from grey-purple to red as demonstrated in .[Citation159–161] In target-mediated growth mechanism, ligand modified AuNPs act as a probe where ligand is detached from AuNPs in the presence of an analyte due to higher ligand analyte affinity whereas ligand-deprived AuNPs behave as an active substrate for AuNPs growth in the presence of HAuCl4/NH2OH. The grown AuNPs appear as a red solution as shown in . In the absence of the target, the ligand modified AuNPs react with HAuCl4/NH2OH and show morphological changes resulting in a blue color solution. Of note, an analyte accelerates the growth of AuNPs in growth-mediated assay but grown AuNPs size is varied and mainly depends on analyte concentration. The growth of bigger size AuNPs leads an aggregated state with blue color.[Citation162] Other possible growth mechanisms are enzyme-promoted and enzyme passivated growth of AuNPs where an enzyme decomposes a non-reducing substrate into reducing agents to start AuNPs growth by redox chemistry or an enzyme produces inhibitor to block the growth of nanoparticles in the presence of an analyte.[Citation158] Common application of growth-based assay is the plasmonic ELISA (enzyme-linked immunosorbent assay) that improves the sensitivity of an assay.
Figure 5. Representation of different mechanisms in AuNPs-related surface plasmon resonance-based colorimetric sensors. A(i) Seed-mediated growth mechanism promotes growth of AuNPs from seeds of Au by chemicals or enzymatic action in the presence of an analyte to produce colorimetric read-out; A(ii) target-mediated growth mechanism involves the ligand modified AuNPs where ligand detaches the Au surface due to strong affinity with analyte; hence ligand-deprived AuNPs start growing in the present of HAuCl4/NH2OH; (B) accumulation-based mechanism for lateral flow immunoassay (LFIA) devices where analyte is detected by the appearance of red line due to accumulation of AuNPs after immunological reaction.
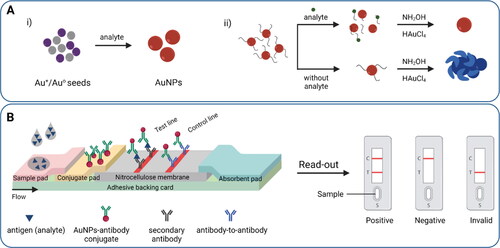
Accumulation-based mechanism of the SPR-based colorimetric sensors is common in lateral flow immunoassay (LFIA) devices which follow an immunological reaction for the detection of an analyte mainly in a qualitative manner. The test strip contains different zones and is equipped with a test line and a control line. Sample containing a target analyte is applied on the sample pad that transfers into the conjugate pad due to the capillary flow of the liquid. The conjugate pad contains specific AuNPs-antibodies which bind to the target analyte, resulting in AuNPs-antibody-analyte conjugates that flow further and reach to the detection zone. Instead of antibodies, aptamers can be used in the conjugate pad.[Citation163] The detection zone comprises porous nitrocellulose membrane with immobilized lines of antibodies that cannot be washed away with liquid. In the test line, the target analyte is sandwiched between two antibodies, resulting in the accumulation of AuNPs and a visible red color. In the control line, AuNPs-antibodies, whether conjugated with the analyte or not, bind with another type of antibody to ensure the proper sample flow by capillary action as shown in . In LFIA, AuNPs has been used for signal amplification in addition to detection.[Citation164] Various AuNPs have been evaluated for the test zone to achieve better detection brightness.[Citation165]
Mechanisms of AuNPs-related peroxidase-activity-based colorimetric sensors
Besides SPR characteristics of AuNPs, peroxidase-like catalytic behavior of colloidal gold has been explored to fabricate colorimetric sensors, also called nanozyme sensors. In the nanozyme mechanism, positive/negative charged AuNPs mimic the function of biological enzymes such as peroxidase, reductase, catalase, glucose oxidase, or superoxidase due to their enzyme-mimetic properties.[Citation166,Citation167] Being highly efficient and highly selective, these AuNPs accelerate chemical reactions like natural enzymes. However, their ease of preparation, cost-effectiveness, high stability due to lack of denaturation and inactivation, and intrinsic catalytic activity put AuNPs nanozyme in a superior position in catalysis which is fundamental to reactions. Among all, peroxidase activity of AuNPs is frequently used in environmental analysis and food safety while other enzymatic properties are beneficial for chemical, pharmaceutical, and clinical applications,[Citation168] which is not the scope of this review. Surface chemistry and morphology of nanoparticles play important role in peroxidase activity-based colorimetricsensors similar to SPR-based colorimetric sensors. However, in contrast to SPR-based colorimetric sensors, AuNPs themselves do not contribute in visual read-out in nanozyme sensors, so chromogenic substances such as 3,3′,5,5′-tetramethylbenzidine (TMB), 2,2′-azino-bis(3-ethylbenzothiazoline-6-sulphonic acid (ABTS), and o-phenylenediamine (OPD) are required to generate color in the presence of analytes.[Citation169] The choice of chromogenic agent depends on different detection parameters such as pH, temperature, and chemical moieties of the target analyte and functionalized-AuNPs. The nanozyme-based mechanism with TMB as a representative example of a chromogenic substance is demonstrated in .
Figure 6. Principle of nanozyme sensors showing peroxidase-mimic role of AuNPs behind colorimetric detection. (A) Analyte detection based on expression of peroxidase-like activity of modified AuNPs that oxidize TMB substrate strongly in the presence of H2O2; (B) analyte detection based on inhibition of peroxidase-like activity of AuNPs where the surface of AuNPs is blocked due to analyte which suppress their peroxidase activity resulting no change in color of TMB.
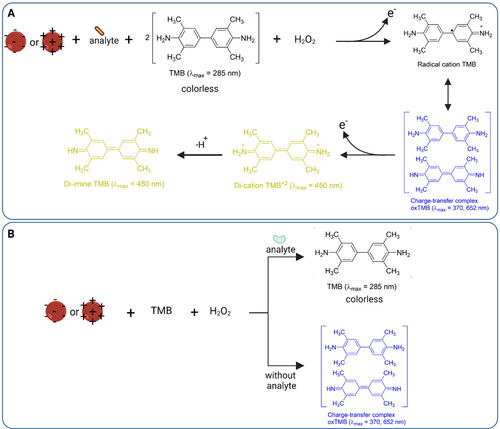
Peroxidase enzyme catalyzes the oxidation of substrate in the presence of hydrogen peroxide which generates a signal being used to detect an analyte. Of note, smaller size of AuNPs shows better activity due to enhanced surface availability for catalytic reaction.[Citation168] In case of peroxidase-like activity under acidic condition, H2O2 adsorbed on AuNPs surface generates ·OH radicals by breaking the O–O bond followed by partial electron exchange interaction between ·OH radicals and the AuNPs that would stabilize the radicals. Whereas under basic conditions, OH groups are adsorbed on AuNPs that trigger the decomposition of H2O2 into H2O and O2.[Citation168] Many toxic substances and contaminants such as heavy metals, pesticides, antibiotics, and micro-organisms have been detected utilizing AuNPs-nanozyme sensors.[Citation168]
Nanozyme sensors employing peroxidase behavior of AuNPs detect analyte of interest either through expression or inhibition of peroxidase activity. Nanozyme sensors based on expression of peroxidase-like activity of AuNPs are common due to their ability to transform into Au0 and Au1+ oxidation state. In this method the target analyte can be detected by the expression or enhancement of peroxidase-like activity of AuNPs with respect to analyte concentration. AuNPs mimic the role of horseradish peroxidase to catalyze the oxidation of TMB/ABTS/OPD in the presence of H2O2 as the oxidizing agent to develop a blue/green/yellow color at absorbance 652/735/450 nm, respectively, in aqueous media which represents colorimetric assay. Bare-AuNPs show peroxidase-like activity which is tuned by ligand modification as well as in the presence of an analyte as shown in . Conclusively, the sensitivity of nanozyme-based assay can be controlled by modifying AuNPs surface which offers adjustable activity. Gold nanoclusters, nanostar, and nanotriangles show better activity due to having more interfaces as compared to nanosphere. In addition to shape, functional groups tailor the surface energy of AuNPs which plays an important role in catalyzing a substrate. Overall, expression or enhancement of peroxidase activity in the presence of analyte is a promising approach to detect and monitor environmental pollutants and food toxic substances.
Peroxidase activity-based chemosensors rely on the inhibition of peroxidase activity in the presence of an analyte is less prevalent due to natural peroxidase-like catalytic ability of AuNPs. Inhibition-based detection strategy is not as straightforward as other colorimetric approaches. In this assay, the surface of gold particles is blocked by an inhibitor, and hence, it is no longer available to express peroxidase-like catalytic role as shown in . For instance, sulfide ions (S2−) inhibit the active sites of gold by forming Au2S species which provide coordination shielding on the surface of AuNPs.[Citation170] Sometimes halide ions (X−) work well to suppress the surface activity of protein-AuNPs by Au–X interactions.[Citation171] In other situations, AuNPs might get aggregated due to the presence of the target analyte and lose their intrinsic-catalytic behavior. For instance, Zhao et al. reported sulfate ions detection through inhibition of peroxidase activity of cysteamine-AuNPs because sulfate induced the aggregation of AuNPs via hydrogen bonding and electrostatic interaction, so a relatively lighter blue color was observed as a reduced colorimetric signal.[Citation172] Overall, inhibitor molecules attached to AuNPs surface are responsible to decrease the peroxidase activity, which is reflected in the color of the solution.
To this end, fundamental mechanisms involved in colorimetric detection of various analytes using AuNPs has been explained. The SPR-based or peroxidase activity-based catalytic sensing behavior of AuNPs mainly depends on surface modifications of nanoparticles which is suitably selected according to the nature of analyte to be detected. In some cases, reversible nature of aggregation and dispersion of AuNPs helps to develop a sensor which is repeatedly “on” and “off” and could be measured by visual read-out. In advance scenario “lab-on-a-nanoparticle” has been successfully developed for the detection of multiple analytes using a single sensing element.[Citation173] Hence, AuNPs have used in several detection applications involving environmental and food monitoring.
Applications of AuNPs in environmental and food monitoring
Gold nanoparticles have been used in environmental and food monitoring as a sensing tool to identify and prevent the potential hazards that could affect the quality of the atmosphere and food. Many environmental pollutants are toxic chemicals produced from inorganic, organic, and biomolecules that are widely dispersed in ambient air, soil, and water.[Citation43] These contaminants release into our environment through various natural and anthropogenic sources like industrial wastes, agricultural fertilizers, and medical diagnostics.[Citation174] These chemical pollutants present risks to humans, animals, plants, and ecosystems through many ways like direct ingestion, food chains, absorption by plants, and consumption of contaminated water. Also, when their amount crosses the threshold limit, these contaminants are considered poisonous and responsible for several ailments that cause serious health issues such as cardiac diseases, brain and nerve damage, pneumonia, liver and kidney failure, and infertility and miscarriages.[Citation174] Hence, modified AuNPs have been used to rapidly detect and quantify various analytes of interest responsible for potential hazards to the environment and humans. Additionally, the colorimetric assay has been used in combination with fluorometric, luminescent, electrochemical or capillary electrophoresis methods for better results.[Citation175] Some researchers have shown luminescent-based G-quadruplex detection technique as a reliable method to monitor small molecules, heavy metals, and other analytes.[Citation176–178]
On the other hand, food inspection agencies are continuously reporting ever-increasing food frauds and adulterants. Food sectors are working hard to ensure food safety and food quality to avoid any possible contaminations such as pesticides, toxins, and foodborne pathogens. Hence, food safety screening and regular monitoring are immensely important to guarantee the purity of food samples. For decades, food microfluidics present improved solutions for food analysis,[Citation179] and in this connection, AuNPs have been used in rapid detection protocols to build on-spot testing kits to inspect food quality.
Of note, AuNPs have also been used for the colorimetric detection of gaseous target molecules. In this case, the AuNPs probe can be solid as films or liquid as solution depends on the nature of targeted gas and detection mechanism. For example, H2S gas was detected using Au-TiO2-NiO nanocomposite film in 2–10 ppm range by catalytic oxidation of H2S into SOx due to the oxide matrix.[Citation180] The film porosity allowed gas molecules to reach the active sites on AuNPs surface where an interaction between sulfur and Au surface led to a reduction in oscillating free electrons resulting in broadening of plasmon peak.[Citation180] On the other side, H2S gas in air was detected using Tween 80 functionalized AuNPs solution with an LOD of 0.5 ppm. The detection principle followed anti-aggregation mechanism.[Citation181] Various other gas molecules such as nitrogen oxide (NO), phosgene (COCl2), and formaldehyde (CH2O) have been detected using SPR property of AuNPs.[Citation181–184] However, detection of gas is a broad area of research that falls out of the scope of this article, so in the following sections only samples in solid and liquid phase will be considered.
Detection of inorganic ions in environment and food
Inorganic pollutants are non-carbon-based substances mainly of mineral origin that have released into the environment as by-product of mining, transportation, and industry and urban activities.[Citation185] Their improper dumping and excessive accumulation in soil and water bodies are a matter of great concern. Their accumulation in living body damages immune system and attacks on different body organs.[Citation186] In this concern, one of the major challenges is the precise on-site analysis of metal contamination in aqueous bodies. Literature contains many examples of alkaline earth metals, heavy metals, trace elements, and rare earth elements that have been detected using SPR and peroxidase-like catalytic properties of functionalized AuNPs.[Citation187,Citation188] Of note, after detecting a particular analyte, interference study with other anions like NO3−, NO2−, F−, Cl−, Br−, I−, CO32−, HCO3−, HSO3−, S2−, SCN−, HPO42−, H2PO4−, H2PO2−, AsO2−, and HAsO42− as well as cations like Li+, K+, Na+, Ca2+, Mg2+, Ba2+, Sr2+, Pb2+, Mn2+, Hg2+, Zn2+, Cd2+, Cu2+, Ni2+, Fe2+, Co2+, Al3+, Cr3+, Fe3+, and Cr6+ is necessary to report the outcomes of the sensor reliably in terms of the selectivity. In this review, we have categorized inorganic pollutants based on their position in the periodic table including alkaline earth metals, transition metals including heavy metals and coinage metals, rare earth elements, and anions. highlights the elements of periodic table that have been detected using AuNPs so far.
Figure 7. The elements of the periodic table that have been detected by using gold nanoparticles so far (the highlighted ones).
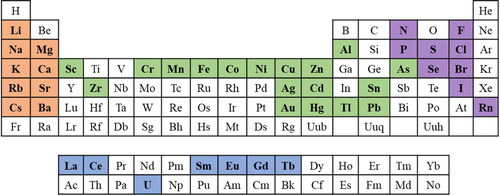
Alkali and alkaline earth metal ions
The alkali and alkaline earth metals occupy the first two columns in the periodic table and are referred to as Groups IA and IIA, respectively. Among all, Na+, K+, and Ca2+ are the most important ions to be detected in water and food samples in addition to their detection as biomarkers in biological fluids including urine, serum, saliva, and sweat samples. Mostly, group IA and IIA salts are utilized to aggregate the AuNPs probe used for the detection of DNA.[Citation189]
Naked eye visualization of K+ ions was made by the interactions of cationic Yellow 5gl dye (Y5GL) with AuNPs that behaved as a new aggregator for colorimetric sensor.[Citation190] In this case, potassium binding aptamers were dissociated from the surface of the AuNPs in the presence of K+ and made a G-quadruplex structure; hence promoting the aggregation of free AuNPs due to balancing the surface charge with the cationic dye. This process is recognized by color change from blue/purple to green as shown in . The use of cationic dye as an aggregator instead of salt have improved the accuracy of the method by offering less interference with existing salts. To improve the sensor’s sensitivity and selectivity toward K+, the pH and incubation time was also important. This approach has also been investigated for paper-based microfluidic systems as a potential method to detect K+ in biological fluids on-spot. Qiu et al. have proposed a new strategy for the detection of Cs+ ions with LOD of 30 µM using ferrocyanide-based Prussian blue precursor (PB-P) and citrate-AuNPs.[Citation191] This colorimetric assay followed nonmorphological transition mechanism similar to anti-aggregation based detection. In the absence of Cs+ ions, PB-P formed nanoshells on Au surface due to reduction of Fe3+ to Fe2+ by the citric acid resulting the color change from red to blue. However, a stable complex formed between Cs+ and PB due to their strong affinity in the presence of targeted analyte which remained AuNPs wine red as shown in . Recently, a transparency sheet-based approach for the detection of Ca2+ in water and artificial urine sample was reported by 4-amino-6-hydroxy-2-mercaptopyrimidine monohydrate capped AuNPs with a LOD of 3.05 ppm at pH 6.[Citation194] The thiol moiety of the functional group was chemisorbed on AuNPs surface, whereas the hydroxyl and amine groups were available for easy binding with Ca2+ ions. This real-time on-site detection method is helpful to develop point-of-use diagnostic systems for Ca2+ ions detection based on aggregation mechanism of AuNPs.
Figure 8. An overview of AuNPs-based colorimetric detection methods for various inorganic analytes. (A) Selective aptasensor for the detection of potassium ions ions using aptamer modified AuNPs as nanoprobe and Cationic Yellow 5GL dye as an aggregating agent which showed green color in the presence of K+ due to interactions between free AuNPs and cationic dye. Reproduced with permission from Ref. [Citation190] with modifications. Copyright 2018 Elsevier; B) detection of cesium ions by nonmorphological transition mechanism where a stable complex between Cs+ ions and Prussian blue precursor (PBP) is formed due to their strong affinity and AuNPs remained dispersed and red in color showing the presence of Cs+ ions whereas the AuNPs color and morphology was changed in the absence of Cs+ ions due to the formation of PB nanoshells on AuNPs surface by the reduction of Fe3+ into Fe2+ in acidic condition[Citation191]; (C) a label-free detection strategy for mercury ions based on Hg2+ and DNA adsorption on AuNPs which affected their stability. The C(i) represents unwashed AuNPs having free citrate molecules which make an amalgam with Hg2+ and promoted DNA adsorption on AuNPs which make the system stable against salt-induced aggregation hence, showing red in color whereas in C(ii) no free citrate molecules were present in a system so a low concentration of Hg2+ was adsorb on AuNPs which promoted higher DNA adsorption, giving more stability to the particles. Hg2+ adsorption was dominated when its concentration was >5 μM which favored less DNA adsorption hence producing blue color in the detection system. Reproduced with permission from Ref. [Citation192] with minor modifications. Copyright 2021 American Chemical Society; (D) detection of cadmium ions using guanidine thiocyanate modified AuNPs by crosslinking aggregation mechanism due to coordinate bonding interaction between Cd2+ and functionalized nanoparticles. Reproduced with permission from Ref. [Citation193] with some modifications. Copyright 2021 Elsevier; (E) detection of inorganic bromide ions using anti-aggregation mechanism of citrate-AuNPs assisted by Cr3+ ions. Reproduced with permission from Ref. [Citation153] with minor modifications. Copyright 2018 Elsevier.
![Figure 8. An overview of AuNPs-based colorimetric detection methods for various inorganic analytes. (A) Selective aptasensor for the detection of potassium ions ions using aptamer modified AuNPs as nanoprobe and Cationic Yellow 5GL dye as an aggregating agent which showed green color in the presence of K+ due to interactions between free AuNPs and cationic dye. Reproduced with permission from Ref. [Citation190] with modifications. Copyright 2018 Elsevier; B) detection of cesium ions by nonmorphological transition mechanism where a stable complex between Cs+ ions and Prussian blue precursor (PBP) is formed due to their strong affinity and AuNPs remained dispersed and red in color showing the presence of Cs+ ions whereas the AuNPs color and morphology was changed in the absence of Cs+ ions due to the formation of PB nanoshells on AuNPs surface by the reduction of Fe3+ into Fe2+ in acidic condition[Citation191]; (C) a label-free detection strategy for mercury ions based on Hg2+ and DNA adsorption on AuNPs which affected their stability. The C(i) represents unwashed AuNPs having free citrate molecules which make an amalgam with Hg2+ and promoted DNA adsorption on AuNPs which make the system stable against salt-induced aggregation hence, showing red in color whereas in C(ii) no free citrate molecules were present in a system so a low concentration of Hg2+ was adsorb on AuNPs which promoted higher DNA adsorption, giving more stability to the particles. Hg2+ adsorption was dominated when its concentration was >5 μM which favored less DNA adsorption hence producing blue color in the detection system. Reproduced with permission from Ref. [Citation192] with minor modifications. Copyright 2021 American Chemical Society; (D) detection of cadmium ions using guanidine thiocyanate modified AuNPs by crosslinking aggregation mechanism due to coordinate bonding interaction between Cd2+ and functionalized nanoparticles. Reproduced with permission from Ref. [Citation193] with some modifications. Copyright 2021 Elsevier; (E) detection of inorganic bromide ions using anti-aggregation mechanism of citrate-AuNPs assisted by Cr3+ ions. Reproduced with permission from Ref. [Citation153] with minor modifications. Copyright 2018 Elsevier.](/cms/asset/7921741f-c866-4085-8da3-dd07cb381b87/batc_a_2162331_f0008_c.jpg)
Conclusively, aggregation and anti-aggregation mechanisms have been reported for alkali and alkaline metal ions detection utilizing aptamers binding and ligand’s affinity approach. Aptamers have gained much interest due to their, high stability, and selectivity compared to antibodies with the same functionalities. Nonetheless, developing aptamers against special analytes is usually done through SELEX procedure which is time-consuming and labor-intensive. Importantly, cationic dye has been used as an efficient AuNPs aggregator instead of conventional inorganic salts. This replacement could be implemented in future to enhance the sensitivity of already established sensors. The affinity of Prussian blue toward Cs+ has been explored using citric acid as a reducing agent which took 20 minutes of incubation. Other reducing agents could be tested to reduce the assay time.
Transition metal ions
Transition metals are the d-block elements that belong to group 3–12 of the periodic table, where a few of them are popularly-known as heavy metals, while others are called coinage metals that have had historical importance. The d-block elements of periodic table are the most common analytes detected in water and food matrices using AuNPs-based colorimetric approaches.
Heavy metal ions
Developing an efficient and sensitive AuNPs-related SPR-based colorimetric sensors and peroxidase activity-based colorimetric sensor for heavy metals such as Ni2+, Hg2+, Cd2+, As3+, Cr3+, Cr6+, and Pb2+ requires specific chemical or biological surface modifications of nanoparticles to prepare a colorimetric probe.[Citation39,Citation195] In a label-free AuNPs-based biosensor, specific DNA sequence was used to build thymine-Hg2+-thymine complex which is used to detect Hg2+ on paper platform.[Citation196] In such a system, conformational shift produced by interactions between DNA and heavy metal are important.[Citation192] However, recently Hu et al. modified this approach by utilizing washed and unwashed AuNPs where interactions between Hg2+ and AuNPs were considered instead of DNA/Hg2+ binding. In this detection system, a blue-to-red color change was observed with increasing concentration of Hg2+ while red-to-blue color produced for the washed AuNPs as shown in .[Citation192] Another example of SPR-based colorimetric sensors is based on analyte aptamer affinity approach for the quantitative detection of Cd2+ using AuNPs.[Citation197] In general, aptamers provide the stability to gold nano-plasmon and keep them well dispersed in the solution. The availability of free aptamers around AuNPs become less in the presence of Cd2+ ions due to Cd2+ aptamer specific interactions which reduces the overall stability of AuNPs system. As a result, AuNPs aggregate in high-salt solutions, which leads to the color change of the solution. No salt-induced aggregation was applied in a colorimetric system in the absence of Cd2+ due to the higher stability of aptamer-AuNPs. In a cross-linking aggregation approach, the quantification of Cd2+ was achieved using guanidine thiocyanate (GT) modified AuNPs.[Citation193] A stable complex was formed between Cd2+ and GT-AuNPs because Cd2+ stimulated the chelation crosslinking of GT-AuNPs through coordinate bonding resulting in a new peak at 682 nm of blue color agglomeration of AuNPs as shown in . This assay showed a linear range of 0.025–50 μM with a LOD of 10 nM.
Qi et al. have reported the nanozyme sensor using oxidase mimetic activity of unmodified AuNPs, where the negative surface of AuNPs contributes toward oxidation of TMB in the presence of Cr6+ resulting in a blue color solution.[Citation198] The strong electrostatic interactions between AuNPs and TMB cations improved the sensitivity of the Cr6+ detection at pH 3. The LOD for this sensor was 93 µg L−1 for water sample which was improved to 0.1 µg L−1 by Mohamed et al. in the same year using SPR-based colorimetric sensor that relied on aggregation-induced color change of maleic acid-functionalized AuNPs.[Citation199] Recently, colorimetric approach was coupled with electrochemical detection to fabricate a dual sensors system for Cr3+ sensing using 3-mercaptopropionic acid ligand adsorbed on AuNPs.[Citation200] This dual sensing system had LOD in uM which was improved to nM by replacing the ligand with L-glutamic acid.[Citation201]
In short, heavy metal ions are the most studied analytes among other metals due to their easy complexation with AuNPs and abundant existence as pollutants. It is common to build aggregation-based detection sensors because of fast chelation reactions between AuNPs and metal ions. Also, researchers have developed multiplex colorimetric systems for simultaneous detection of groups of ions, including Fe3+, Cr3+, Co2+, Mg2+, Pb2+, Ca2+, Zn2+, Ti4+, and Sn4+,[Citation202] Hg2+, Cd2+, Pb2+ and Cu2+,[Citation203] Ti4+, Cr3+, Mn2+, Fe3+, Pb2+, and Sn4+,[Citation204] Pb2+ and Cr3+,[Citation205] Hg2+ and As3+.[Citation206] As a principle, AuNPs were decorated with “suitable” multiple colorimetric chelators and used under optimized conditions to achieve the detection of the target analytes.
Coinage metal ions
Copper, silver, and gold are considered coinage metals in addition to being plasmonic nanomaterials, and hence, their nanoparticles can be used to develop analytical devices for applications in environmental and food monitoring.[Citation207,Citation208] Lu et al. have reported an interesting approach for the detection of Cu2+ ions in nM level using Ag coated AuNPs.[Citation209] This non-aggregation method is based on catalytic leaching of silver around AuNPs by the action of Cu2+ in the presence of thiosulfate; hence, the size of AuNPs decreased which affected the SPR properties of nanoparticles. Also, anti-aggregation/non-aggregation of AuNPs using polyvinylpyrrolidone/2-mercaptobenzimidazole, D-penicillamine[Citation151], and catalytic etching approach via hexadecyltrimethylammonium bromide[Citation210] have been reported for Cu2+ detection previously. In another report, the addition of Cu2+ to histidine modified AuNCs impaired peroxidase mimetic activity of AuNCs because the His/Cu2+ complex was more stable due to the presence of the imidazole ring in histidine. However, this change was fully reversible by the addition of free histidine as ambidentate nature of amino acids was helpful for the selective recognition of Cu2+ with LOD 0.1 nM. In this method, histidine as a ligand and TMB/H2O2 as a substrate was used.[Citation211]
Utilizing the induced hybridization concept between two different ssDNAs adsorbed on AuNPs was employed for the colorimetric detection of Ag+ in dark field microscopy.[Citation212,Citation213] This imaging assay depended on the specific binding affinity between Ag+ and cytosine-bases of ssDNAs that plays a dominant role in the production of C-Ag+-C complex via aggregation of AuNPs. The color change of imaging happened at a single AuNP level from green to yellow and finally red which quantified the amount of silver ions. In a recent report, anti-aggregation mechanism of unmodified AuNPs made Ag+ detection possible in the presence of thiamazole.[Citation152] Anti-aggregation based detection of Ag+ ions with LOD of 0.41 µM was reported employing tris(hydroxymethyl) aminomethane (tris) that inhibited AuNPs aggregation resulting in hypsochromic spectral shift with a color change from blue to red.[Citation214]
On the other hand, aggregation-based mechanism was explored using peptide-AuNPs with high sensitivity as 7.4 nM.[Citation215] The 4-coordinated complexes of Ag+ with peptide was developed through the oxygen of carbonyl group and the nitrogen of α-amino group that induced folding in peptides leading to the aggregation of peptide-AuNPs. Metallization-induced aggregation of chitosan-AuNPs also responsible for Ag+ ions detection with LOD of 130 nM where Ag+ interacted with the Au core and establish a metallic bond which was reduced by chitosan and deposited on AuNPs. This deposition produced Au–Ag core–shell NPs with a distinct color change from pink to orange.[Citation216] Another sensitive Ag+ ions detection in 2.05 nM was done with gallotannin-capped AuNPs that interacted with Ag+ resulting in a color change from pink to yellowish brown hence applicable for quantitative determination of Ag+ ions in real water samples.[Citation217] According to our best understanding, there is no method available for the detection of Au+ using colorimetric approaches; however, this ion has been detected using absorption and fluorescence methods.[Citation218] As AuNP itself act as a transducer for the detection of a wide range of analytes and must be present in the detection system, so its own colorimetric detection would be quite difficult.
In conclusion, AuNPs-based colorimetric approach for the detection of Cu2+ is less explored in recent years due to its easy deposition on electrodes which favors the construction of electrochemical biosensors.[Citation219] However, ligand modified AuNPs have been used for Cu2+ detection employing anti-aggregation, etching, and nanozyme mechanisms. Anti-aggregation approach benefits from the chelation reaction, where Cu2+ acts as a central metal toward ligands and hence keeps the AuNPs dispersed. Though the etching process is efficient and quick, but it mainly depends on the presence of thiosulfate which could be a potential interference for another matrix. Nanozyme-based approach is majorly pH-dependent which works well at pH 3 and hence limits Cu2+ detection in neutral and alkaline medium. Colorimetric-based determination of Ag+ has happened with aggregation and anti-aggregation mechanism. Aggregation-based detection is advantageous due to its superior simplicity, time-saving, and high sensitivity in nM as compared to anti-aggregation mechanism which offers multistep detection with detection limit in µM. The aggregation-based assay should be investigated on solid portable system for an easy on-spot Ag+ determination.
Rare earth metal ions
Rare earth elements belong to f-block of periodic table which include inner transition metals as lanthanide and actinide series. These metals have many applications in technological fields such as sustainable energy construction, electric automobiles, and microchip technology.[Citation220] These metal ions may continually circulate and alter in the environment and accumulate in biological tissues, where their potential adverse effects to ecology and humans become obvious.[Citation221] For example lanthanide (Ln3+) competes with calcium ions and keep depositing in bone tissue of human body,[Citation222] while cerium ions (Ce3+) accumulate in human tissues and liver,[Citation223] and causing cancer.[Citation224,Citation225] For these reasons, many efforts have been done to detect these metal ions using AuNPs.[Citation221]
Malonamide modified AuNPs have been used to detect Ln3+ ions such as Eu3+ and Sm3+ with LOD of 50 nM using aggregation-based colorimetric assays.[Citation226] In recent years, hydroxypyridinonate chelator was used as an indirect approach to sense a few lanthanides such as La3+, Gd3+, and Tb3+ while quenching AuNPs.[Citation227] This sensing principle involved the inhibition of particle growth due to lanthanides and offered a LOD of 3 μM to quantify a targeted analyte in industrial waste. Moreover, this assay offered a tunable dynamic range by varying Au3+ concentrations and octadentate chelator. In another report, biogenic AuNPs were used to detect Ce3+ through fast aggregation of particles due to the formation of coordinate complex between Ce3+ and –COO/–NH surface functionalities of AuNPs.[Citation220] This agglomeration was reversible by the addition of EDTA which leads to chelation with Ce3+ ions, leading to the regeneration of the dispersed AuNPs in the solution as red color colloids. A dual colorimetric sensor was built for the simultaneous detection of Eu3+ and Cr3+ ions in aqueous media utilizing 1,2,3-triazole-4,5-dicarboxylic acid adsorbed on AuNPs at fairly good pH range 4-10.[Citation228] This ligand offered high selectivity as a range of heavy metals and other lanthanide ions have been tested as potential interference. Free carboxylic groups around AuNPs shifted an SPR peak from 527 to 633 nm in case of Cr3+ ions and from 527 to 671 nm for Eu3+ ions resulting in the color change from red to blue. To conclude, a few lanthanides such as Eu3+, Sm3+, Ce3+, and Gd3+ have been detected using instrument dependent colorimetric approach of AuNPs. There is no report for on-site detection methods of these trivalent metal ions except for Eu3+.[Citation228] Ce3+ has been detected using biogenic AuNPs which make a synthetic method environment-friendly due to extracellular culture of fungus instead of harsh chemicals, but it requires careful handling so there is a need to explore Ce3+ detection using chemical synthetic methods.
Anions
Anions are negatively charged ionic species that include halide ions (F−, Cl−, Br−, and I−), oxoanions (HSO4−, H2PO4−, HPO42−, CH3COO−, and NO3−), cyanide (CN− and SCN−), sulfide (SO42−, SO32−, and S2−) and other anions (N3−). Anions have the affinity to bind with biological materials such as protein, DNA, and RNA causing chronic diseases,[Citation229] and hence their timely detection is important. However their colorimetric detection using AuNPs is more challenging as compared to cationic species due to their lower charge to radius ratio, wide range of geometries such as linear, spherical, trigonal, tetrahedral, pH sensitivity, and solvation dependent binding strength and selectivity.[Citation230] Recently, Sepahvand et al. documented the role of nano-plasmonic materials for the detection of nitrate and nitrite ions through aggregation, anti-aggregation, and etching-based detection mechanisms.[Citation231] Recently, nitrite ions were detected (LOD 0.012 mg L−1) as a degradation product of ammonium dinitramide using AuNPs coupled with azo dye ingredients necessary for the Griess assay, which is a common method for nitrite detection.[Citation232] In another study, nitrite ions were detected by iodide accelerated etching mechanism using 20 nm Au nanospheres, nanorods and nanotriangles which induced a plasmonic shift toward longer wavelength.[Citation233] Nitrite being oxidant oxidizes iodide ion and facilitates the etching of AuNPs by iodide ions. The LOD for etching method was 4.5 μM[Citation233] comparable to the LOD of nanozyme method which was 4.6 μM.[Citation234] Nitrite ions besides being an analyte were used as an electrophilic oxidant to oxidize TMB substrate in the presence of AuNP-cerium oxide (CeO2) NP-adsorbed on graphene oxide (GO) sheets. The oxidized TMB produced green color as signal read-out. This study reported a colorimetric recognition for nitrite in aqueous solution based on peroxidase-mimic role of hybrid AuNPs.[Citation234]
An important anion coexisting with nitrate is the phosphate ion which was detected using mercaptoacetic acid (MA) adsorbed on AuNPs with europium (Eu3+) as an aggregating agent.[Citation235] Underlaying detection principle is based on anti-aggregation phenomena of MA-AuNPs in the presence of Pi. The coordination of carboxylate groups of MA with Eu3+ was significantly interrupted due to the higher binding affinity of Eu3+ for Pi, and hence MA-AuNPs remain dispersed. The LOD for this method was 76 nM which was significantly improved by our research group to 3 nM utilizing the Eu3+ encapsulated dextran tablets.[Citation118] Similarly, anti-aggregation mechanism was explored for the detection of bromide ions Br− with and a LOD of 0.04 mM using citrate-AuNPs as a colorimetric probe and Cr3+ as a cross-linking aggregating agent.[Citation153] The coordination of Cr3+ with carboxylate groups of citrate ions resulted in a color change from red to blue without Br−, whereas the solution color remained red in the presence of Br− ions as shown in .
Conclusively, anions detection is more difficult than cations recognition due to different chemical nature of these ionic entities. Nitrite and nitrate ions have been detected with AuNPs via different mechanisms including aggregation, anti-aggregation, nanozyme and etching, but all of them have involved acidic environment because of underlaying chemistry. Griess reaction involved in nitrite detection is acid catalyzed; hence, no detection is recorded in alkaline media which needs exploration. Also, phosphate ions have been reported by colorimetric sensors which can be converted to in-field applications in future.
Detection of organic pollutants in environment and food
Organic pollutants refer to biodegradable contaminants in environment, which can cause serious toxicological effects such as carcinogenicity and neurotoxicity.[Citation236,Citation237] Persistent organic pollutants in environment include polychlorinated biphenyls, polybrominated diphenyl ethers, and polycyclic aromatic hydrocarbons as a result of industrial and household waste.[Citation238–243] Other well-documented pollutants are pesticides, drugs, allergens, mycotoxins, and a variety of small toxic substances such as melamine. The routine use of these organic substances in agricultural and livestock production leads to their heavy accumulation in atmosphere, soil, water, and eventually human body through the food chain, raising threats to the humans. That is why the detection of these contaminants at earliest is crucial, and efforts are ongoing to develop efficient AuNPs-related SPR-based sensors using colorimetric methods to detect and monitor the amounts of organic pollutants in environment and food matrices to prevent their devastating effects. In this connection, several recently published literature reviews have specifically covered the detection of organic pollutants from different aspects.[Citation244–246] In the following sections, we will focus on the recent advancements on the AuNPs-based colorimetric detection of such pollutants.
Detection of pesticides
Being widely used, pesticides have been detected in water, fruit, and vegetable samples using colorimetric probe of AuNPs. Most of the pesticides are rich in active functionalities such as amino, thio, cyano, hydroxyl, carbonyl, and sulfide groups which has binding attraction toward the negatively charged surface of gold particles, and hence distance-induced optical changes in nanoparticles can be seen by naked eye besides UV–vis spectroscopy measurements. Prothioconazole,[Citation247] acephate, phenthoate, profenofos, acetamiprid, chlorothalonil, and cartap pesticides[Citation248] have been detected in µM to nM range with citrate-capped AuNPs. Citrate ions were eliminated after pesticides adsorption on AuNPs, which reduced the electrostatic repulsion force among particles. Van der Waals attraction and hydrogen bonding formation between pesticide molecules facilitated the aggregation of AuNPs which turned solution from red to blue. On the other hand, ligand modified approach worked for some of the pesticides like pencycuron with 6-aza-2-thiothymine,[Citation249] carbendazim with aptamer/poly-diallyldimethylammonium chloride,[Citation250] paraquat, dipterex, cartap, dursban, and methyl thiophanate with tetrakis(N-methyl-4-pyridiniumyl) porphyrin,[Citation251] paraquat with aptamer 77F[Citation252] or sodium 3-mercapto-1-propanesulfonate.[Citation253] In ligand functionalized AuNPs, not only the selectivity but also the sensitivity of the probe was improved. The quantity of pesticides controls an aggregated or dispersed state of AuNPs.
Acetylcholinesterase (AChE) enzyme-mediated detection of organophosphate pesticides (OP) is common and widely used in many applications of water and food safety because the active site of the AChE is permanently blocked in the presence of pesticide, which can be used in pesticide detection with high selectivity. Using this principle, core–shell MnO2-decorated AuNPs were used beside the enzyme to detect OP.[Citation254] Enzyme AChE hydrolyzed acetylthiocholine iodide (ATCh) to thiocholine (TCh) which served as a reducing agent to decompose the MnO2 shell, forming Mn2+ around AuNPs in the absence of OP. This etching process induces a sharp decrease in the extinction coefficient and a blue shift in the SPR peak in the sensing system which could be seen in dark-field microscopy at a single particle level.[Citation254] However, with OPs, AChE enzyme activity was inhibited, and no TCh was produced, and in turn, no etching process occurred. As the whole detection mechanism worked at single particle level, a very low LOD of 0.006 pg mL−1 was achieved.
Other than the etching mechanism, AChE has also been used to indicate nanozyme behavior of AuNPs where peroxidase-like activity was explored for the detection of OP. Without OP, AChE was functional that catalyzed the acetylcholine (ACh) into choline and acetate, but in the presence of OPs such as parathion ethyl pesticide, the AChE became inactive, and resultantly no choline was produced.[Citation255] As choline was absent in the sensing solution, dispersed cysteamine-AuNPs behaved as nanozyme and oxidized TMB in the presence of H2O2 which generated intense blue color solution in the presence of the OP. However, cysteamine-AuNPs were aggregated in the presence of choline and lost their peroxidase-like catalytic activity, resulting in no oxidized TMB production indicated by the colorless solution. Similar peroxidase-mimicking activity of AuNPs has been investigated for the detection of OPs such as dimethoate without AChE assay.[Citation256] In this approach, o-phenylenediamine was used as a substrate instead of TMB which was oxidized to yellow color 2,3-diaminophenazine product in the presence of H2O2. The peroxidase-like catalytic performance of AuNPs was inhibited in the presence of dimethoate pesticide resulting in colorless to light yellow solutions as shown in . This method offered LOD of 4.7 μg L−1.
Figure 9. A representation of different detection strategies for a variety of organic pollutants using AuNPs-based colorimetric sensors. (A) Detection of dimethoate pesticides based on inhibitory effect of peroxidase-like catalytic activity of AuNPs using o-phenylenediamine as chromogenic agent which did not oxidize to a yellow 2,3-diaminophenazine product in the presence of analyte[Citation256]; (B) a label-free biosensor for the detection of Kanamycin antibiotic using AuNPs and arbitrary sequenced DNA where kanamycin adsorbed on AuNPs and responsible for colorimetric signal instead of DNA adsorption on Au surface. Reproduced with permission from Ref. [Citation257] with some modifications. Copyright 2020 American Chemical Society; C) (i) a pollen allergen Cry j 2 recycling, (ii) in the presence of Cry j 2 analyte, P1 and P2 DNA probes were detached from AuNPs constructing two DNA three-way junctions whereas the bare AuNPs showed salt-induced aggregation, (iii) in the absence of target Cry j 2, the P1 and P2 DNA probes were remained adsorbed on AuNPs surface and responsible for interparticle electrostatic and steric repulsion among particles leading no color change after salt addition. Reproduced with permission from Ref. [258] with some modifications. Copyright 2019 American Chemical Society; (D) detection of ochratoxin A by target-responsive aptasensor where ascorbic acid 2-phosphate was hydrolyzed by alkaline phosphatase (ALP) to produce ascorbic acid (AA) which reduced MnO2 to Mn2+ that caused aggregation of AuNPs. Reproduced with permission from Ref. [Citation259] with some modifications. Copyright 2020 Elsevier; (E) an aptasensor-based ochratoxin A (OTA) detection utilizing AuNRs where aptamer-OTA complex released ALP-labelled complementary DNA which catalyzed the ascorbic acid 2-phosphate to ascorbic acid (AA). AA reduced Ag+ ions which deposited on AuNRs resulting multicolor read-out. Reproduced with permission from Ref. [Citation260] with some modifications. Copyright 2020 Elsevier.
![Figure 9. A representation of different detection strategies for a variety of organic pollutants using AuNPs-based colorimetric sensors. (A) Detection of dimethoate pesticides based on inhibitory effect of peroxidase-like catalytic activity of AuNPs using o-phenylenediamine as chromogenic agent which did not oxidize to a yellow 2,3-diaminophenazine product in the presence of analyte[Citation256]; (B) a label-free biosensor for the detection of Kanamycin antibiotic using AuNPs and arbitrary sequenced DNA where kanamycin adsorbed on AuNPs and responsible for colorimetric signal instead of DNA adsorption on Au surface. Reproduced with permission from Ref. [Citation257] with some modifications. Copyright 2020 American Chemical Society; C) (i) a pollen allergen Cry j 2 recycling, (ii) in the presence of Cry j 2 analyte, P1 and P2 DNA probes were detached from AuNPs constructing two DNA three-way junctions whereas the bare AuNPs showed salt-induced aggregation, (iii) in the absence of target Cry j 2, the P1 and P2 DNA probes were remained adsorbed on AuNPs surface and responsible for interparticle electrostatic and steric repulsion among particles leading no color change after salt addition. Reproduced with permission from Ref. [258] with some modifications. Copyright 2019 American Chemical Society; (D) detection of ochratoxin A by target-responsive aptasensor where ascorbic acid 2-phosphate was hydrolyzed by alkaline phosphatase (ALP) to produce ascorbic acid (AA) which reduced MnO2 to Mn2+ that caused aggregation of AuNPs. Reproduced with permission from Ref. [Citation259] with some modifications. Copyright 2020 Elsevier; (E) an aptasensor-based ochratoxin A (OTA) detection utilizing AuNRs where aptamer-OTA complex released ALP-labelled complementary DNA which catalyzed the ascorbic acid 2-phosphate to ascorbic acid (AA). AA reduced Ag+ ions which deposited on AuNRs resulting multicolor read-out. Reproduced with permission from Ref. [Citation260] with some modifications. Copyright 2020 Elsevier.](/cms/asset/ef6c6d15-6bb0-4424-952a-31bb333488e4/batc_a_2162331_f0009_c.jpg)
In summary, a range of pesticides have been detected using bare as well as modified AuNPs employing different mechanistic approaches such as aggregation, etching, and nanozyme. Among all, aggregation-based detection method is frequently studied due to its operational simplicity, but this approach offers a high LOD as compared to ligand modified AuNPs probe. In case of etching-based detection system, AChE assay is frequently employed for the detection of OPs with instrument-dependent read-out like dark-field microscopy. However, AChE assay can also be used for in-field colorimetric detection of OPs when nanozyme role of AuNPs is considered, but in this case, the overall procedure becomes cumbersome which takes longer detection time. Moreover, peroxidase-like catalytic behavior of AuNPs is only functional under acidic environment which limits its application in alkaline systems.
Detection of drugs
So far, various drugs such as neurotransmitters, anticancer, and polyionic drug molecules have been detected in pharmaceutical and biological fluids using AuNPs.[Citation261] However, antibiotic drugs are the main concern in case of environmental and food samples which is the scope of this review article. Different antibiotics such as oxytetracycline, tetracycline, kanamycin, ofloxacin, chloramphenicol, and ceftriaxone have been detected by colorimetric approach of AuNPs.[Citation257,Citation262–265] Their detection is quick and label-free that mainly involves the use of citrate stabilized AuNPs or specific aptamers according to antibiotic compatibility with the assistance of sodium chloride for the visual read-out due to the aggregation of AuNPs.[Citation262,Citation266,Citation267] The aptasensors follow two kinds of mechanisms depending upon the reagent addition sequence. In the most common way, antibiotics cannot induce aggregation in AuNPs directly, but salt can do. That is why, free aptamers are introduced in the detection system as the initial step which are adsorbed onto the surface of AuNPs and provide resistance to aggregation against salt. Regarding the reasons behind aptamer adsorption onto AuNPs surface, there has been inconsistency in the literature. While some researchers have stated that the electrostatic interactions between positively charged nucleobases with negatively charged AuNPs is responsible for adsorption,[Citation266] others have argued that all four nucleobases are charge neutral at neutral pH and the reason for adsorption is coordination.[Citation113] Wu et al. detected multiplex antibiotics using aptamer functionalized AuNPs and smart phone read-out.[Citation266] According to the authors, the recognition part of aptamer bounded with analyte such as chloramphenicol or tetracycline was dissociated from AuNPs as a folded rigid structure. Meanwhile, it was hard for non-recognition fragment of aptamer to maintain AuNPs in a stable dispersed state so aggregation took place upon addition of salt.[Citation266] This method offered LODs of 32.9 and 7.0 nM for tetracycline and chloramphenicol, respectively. Instead of salt, poly dimethyl diallyl ammonium chloride can be used as cationic species which induce aggregation.[Citation268]
In the second mechanism, colorimetric detection was mainly dependent upon antibiotic adsorption on AuNPs instead of aptamer binding which was confirmed by the color change of solution when kanamycin was added to AuNPs as shown in .[Citation257] Moreover, kanamycin did not induce specific desorption of aptamers from the AuNPs as mutated aptamers, and a random DNA sequence were used which were not bound to kanamycin. A comparative study concluded that the inhibited aptamer adsorption on AuNPs surface was due to kanamycin-AuNP interactions and not by the kanamycin-aptamer binding. This assay showed a LOD of 90 nM. The detection procedure takes a relatively longer time (10 minutes) when lateral flow assay was involved[Citation269] but on a paper-based microfluidic device, it took only 2 minutes for the detection of gentamicin.[Citation270] To enhance the signal output, magnetic beads were used as a separator and TMB or OPD as chromogenic substances generated the color in the presence of antibiotic.[Citation169] In short, a wide range of antibiotics have been detected using different aptasensors.[Citation257,Citation266,Citation268,Citation270]
Another approach uses the polymer-stabilized AuNPs for the colorimetric determination of antibiotics such as ceftriaxone and cephradine by aggregation mechanism.[Citation264,Citation271,Citation272] In this scenario, antibiotic reacts with polymer functionalities present at the surface of nanogold. Polymers being rich in oxygen, nitrogen, or sulfur moieties can stabilize AuNPs as well as developing interactions with functional groups of antibiotics to induce aggregation. Specificity and sensitivity of chemical linkages play dominant role in the construction of a selective chemosensor. For example, poly(vinyl alcohol) stabilized AuNPs hydrogel were employed for colorimetric determination of ceftriaxone where borax molecules were used as cross-linker inside the main chain of polymer.[Citation271] The absorbance peak at 517 nm due to AuNPs was decreased in the presence of ceftriaxone, resulting in the size increase of particles with a color change from red to colorless. This assay showed a linear range in 1–90 μg mL−1 ceftriaxone concentration with a LOD of 0.33 μg mL−1.
In summary, aptasensor and chemosensor-based colorimetric detection has been reported for a variety of antibiotics. In case of aptasensors, citrate capped AuNPs without any ligand modification works well with high specificity and sensitivity. High-affinity aptamers can measure lower concentrations of antibiotics. For chemosensors, polymer was used as stabilizing agent and sodium borohydride was used as reducing agent, but this colorimetric probe was less sensitive than aptasensors. However, both approaches have strong potential to fabricate paper-based devices for in-field applications due to strong affinity of these sensors with cellulose matrix. These portable devices would have many applications in food industries and clinical settings.
Detection of allergens
Allergen a sort of antigen proteins or genes that triggers an aggressive immune response that the immune system defends the body against a perceived danger.[Citation273] Allergens significantly impact the quality of life and, hence, remain a big problem for susceptible people especially children.[Citation274] Thus, rapid detection of food allergens utilizing colorimetric response of AuNPs are extremely important to detect.[Citation275] Cross-linking and non-crosslinking aggregation behaviors have been studied in detail to detect a range of allergens in a variety of food samples such as shrimp, fish, eggs, peanuts soybeans, barley, sesame seeds, walnuts, almonds, pistachios[Citation274,Citation276,Citation277] as well as allergenic fungal spore.[Citation278]
In case of cross-linker mediated aggregation, biotinylated antibody as a switchable linker is used to induce aggregation in a detection system. The device follows lateral flow assay where antigen-antibody interactions are the key detection reaction. For example, for the detection of Ara h 1 antigen, it specifically reacted with the biotinylated anti-Ara h1 antibody in the target recognition step which was visualized with the addition of streptavidin-AuNPs.[Citation276] The concentration of cross-linker is important to quantitatively detect the target. On the other side, non-cross-linking mediated aggregation follows hybridization chain reaction between the complementary and target DNA without any enzymes and sophisticated read-out machine.[Citation277] No target DNA was available for the reaction in the absence of allergen gene, so single-stranded portion of hairpin probe remained adsorbed on AuNPs as red dispersed colloids which were resistant to ionic strength. However, in the presence of the allergen as low as 0.5 nM, long double-stranded DNA was formed which could not stabilize the AuNPs and hence released from the gold surface making nanoparticles prone to aggregation after adding NaCl. In a similar way, pollen allergen Cry j 2 was detected at 0.2 ng mL−1 which was 130-times improved due to signal amplification based on catalytic DNA hairpin self-assembly.[Citation258] The bare AuNPs showed purple aggregation after surface detachment of P1 and P2 (DNA probes) that have built two DNA three-way junctions in the presence of Cry j 2. However, in the absence of target Cry j 2, no color change happened in the colloidal solution because of adsorption of ssDNA which was responsible for interparticle electrostatic and steric repulsion among particles as shown in . Overall, allergen genes and proteins can be detected using biomolecules modified AuNPs probe which are highly specific and sensitive. Hybridization chain reaction happened between compatible DNA strands which would allow AuNPs accumulation under high salt concentration. This approach has successful standing in developing biosensors for a variety of different allergen detection.[Citation258,Citation277]
Detection of mycotoxins
Mycotoxins are broad range of toxic secondary fungal metabolites with low molecular weight (300–700 Da) that are harmful for humans and livestock.[Citation279] Out of >300 different types, common examples of mycotoxins are aflatoxins, ochratoxins, zearalenone, deoxynivalenol, fumonisins, patulin, and T-2 toxin, which have been detected using colorimetric sensors. Among them AuNPs-based immunosensors have been used extensively to detect a variety of mycotoxins including fumonisin B1, aflatoxin B1, zearalenone, and ochratoxin A.[Citation280–283] This approach shows multicolor response on lateral flow test strips based on the concentration and type of tested mycotoxin which serves as rapid diagnostic platform for in-field detection. Sample matrix interferences are avoided by simply dilution method.[Citation284]
Second, widely used approach is AuNPs-based aptasensors that specifically bind to the targeted mycotoxin and detach from AuNPs surface making these particles prone to salt-induced aggregation. Through this approach, T-2 toxin in wheat and corn samples were detected at 57.8 pg mL−1 using label-free T-2 specific aptamer.[Citation285] Fumonisin B1 was also detected with aptamers as ssDNA using 40 nt and 96 nt sequence in the presence of Tris-HCl and MgCl2, respectively.[Citation286] Similarly, aflatoxin M1 was detected with LOD of 0.002 ng mL−1 considering 72-mers ssDNA by Lerdsri and coworkers.[Citation287] In another study, instead of salt, charged molecules like cationic perylene was used to detect aflatoxin B1 at 0.18 ng mL−1.[Citation288] However, the detection sensitivity was improved to 35 pg mL−1 by utilizing magnetic bead (MB) associated aptasensor, where hybridization reaction between aptamer and complementary DNA (cDNA) was the key to get higher sensitivity, and the catalytic reaction was responsible for colorimetric detection. In this study, aptamer surrounded Fe3O4@Au magnetic beads and cDNA-AuNPs made a bioconjugate which released the cDNA-AuNPs as signal probe in the presence of aflatoxin B1 due to higher affinity of cDNA with aflatoxin B1 as compared to its affinity with magnetic bead aptamer. The signal probe underwent colorimetric detection utilizing peroxidase-like behavior of AuNPs with TMB-H2O2 system to detect aflatoxin B1.[Citation289] Similar magnetic bead separation strategy has been used for ochratoxin A (OTA) detection with some modifications.[Citation259] Here, alkaline phosphatase (ALP) was used as an external enzyme to facilitate colorimetric response by hydrolyzing ascorbic acid 2-phosphate to the ascorbic acid (AA) which reduced MnO2 nanosheets to Mn2+ that promoted AuNPs aggregation as shown in . The DNA-ALP-immobilized MBs contained OTA aptamer, biotinylated cDNA, and streptavidin–alkaline phosphatase (SA-ALP), where biotinylated cDNA hybridized with OTA aptamer to form an aptamer–cDNA duplex. In the presence of an analyte OTA, an aptamer–cDNA duplex switched to an aptamer-OTA complex which liberated ALP-cDNA from DNA-ALP-immobilized MBs. This magnetic separation is followed by ALP-induced aggregation of AuNPs through Mn2+ ions.[Citation259] The enzyme ALP response was directly related to the OTA concentration through aptamer shape switching on MBs and could detect as low as 5 nM of ochratoxin A. Instead of MnO2 nanosheet, Tian et al. have utilized AuNRs for the detection of OTA where Ag+ ions were reduced to Ag shell around nanorods by AA to generate a blue-shift of the longitudinal SPR peak which showed visible multicolor change as shown in .[Citation260] Another useful probe consists of Fe3O4@Au which has been used for the detection of ochratoxin A at 0.5–80 ng mL−1 detection range by catalyzing TMB under acidic condition.[Citation290]
Besides recognition abilities of AuNPs, these particles have been used as signal amplifier due to their higher surface area which favors the adsorption of larger number of ligands. For example, AuNPs enhanced horseradish peroxidase-linked aptamer assay that was used for the detection of zearalenone as low as 0.08 ng mL−1 in spiked oil sample.[Citation291] Also, three kinds of mycotoxins (i.e. aflatoxins, ochratoxins, and zearalenone) were detected in human food and animal products using three distinct reducing or capping agents on the paper platform.[Citation292] The detection mechanism was based on the sort of bonds that each capping agent created with AuNPs such as hydrogen bonding, and capping agents like caffeic acid and glucose which shared the protons of their hydroxyl groups with analytes. Nevertheless, the interaction between sensor and analyte arose from the acid-base dissociation of these chemical compounds at appropriate pH which caused the aggregation and changing the color of AuNPs from red to blue.[Citation292]
Overall, different shapes of AuNPs such as nanospheres, nanocacti, nanoflowers, and hyperbranched blackbodies have been involved to build immunosensors for the detection of mycotoxins as the common colorimetric strategy, which is simple, portable and timesaving.[Citation282] Selection of AuNPs shape is highly dependant on target of interest which would generate variable color response accordingly and would help to construct multiplex nanosensor. On the other hand, aptasensors for the detection of mycotoxins have been widely used due to its specificity and sensitivity. Aptasensor-based magnetic bead separation followed by enzyme-induced detection would enhance the method’s sensitivity.
Detection of small organic toxins
Physical and chemical interactions of targeted analytes with AuNPs have been used for the detection of a range of small molecules including illegal food additives like melamine,[Citation293] endocrine-disrupting compounds like bisphenol A (BPA),[Citation294] iodinated X-ray contrast chemicals like iohexol,[Citation295] toxic chemicals like nerve agent VX (O-ethyl-S-2-(N,N-diisopropylamino)ethyl methyl-phosphonothioate),[Citation296] disinfectants such as 5-chloro-2-methylisothiazol-3(2H)-one/2-methyl-4-isothiazolin-3-one (CMIT/MIT), and many reducing sugars. Target-AuNPs interactions have been used to study the competitive binding behavior of analyte either with AuNPs or aptamer which would help to build a sensor for a specific analyte. For example, dopamine detection was made possible due to its strong adsorption onto AuNPs, and hence, dopamine was specifically detected in this system.[Citation297] On the other hand, aptasensor was used to detect BPA at pM level with truncated 63-mer BPA-specific aptamer which were 12-mer and 38-mer.[Citation294] The use of truncated aptamer improved the sensitivity of sensor approximately 265-fold with 38-mer and 140-fold with 12-mer as compared to 63-mer aptamer.
Taking the advantages of aggregation mechanism, AuNPs probe commonly utilizes specific molecular recognition approach to detect a wide range of compounds either with bare or modified AuNPs. For example, a fatal chemical compound VX was detected along with its hydrolyzed product 2-(diisopropylamino)ethanethiol (DAET) using citrate capped AuNPs through aggregation mechanism with LOD of 100 nM.[Citation296] Their colorimetric system was highly pH-dependent which favored the VX detection under an acidic environment and DAET detection in alkaline condition. On the other hand, 3-aminophenylboronic acid modified AuNPs have been used for colorimetric sensing of iohexol in environmental samples.[Citation295]
The growth-based detection mechanism of AuNPs was used for the detection of small sugar molecules. Reducing sugars were detected through synthetic approach where room temperature production of AuNPs was dependent on the concentration of reducing sugars involving cetyltrimethylammonium bromide as a surfactant.[Citation298] The participation of sugar in oxidation-reduction reactions of gold salt was responsible for the production of AuNPs and hence, these sugar molecules can be detected by estimating the rate of reaction as higher rate was observed with fructose and lowest with mannose due to their structural chemistry. The variable concentration of sugar leads to different reactions time and yield of nanoparticles. LOD values for monosaccharides such as fructose, glucose, and mannose were 0.067, 0.081, and 0.106 mM respectively while for disaccharide such as lactose was 0.087 mM.
Detection of melamine using SPR properties of AuNPs is a hot topic in colorimetric sensing due to easy aggregation of nanoparticles induced by exocyclic amino groups of melamine.[Citation299,Citation300] Moreover, AuNPs synthesized either by chemical reduction or plant extracts suit well in detection. The LOD of 8 nM was observed with 3-mercapto-1-propanesulfonate ligand which was improved to 1 nM when bare AuNPs were used.[Citation293,Citation301] Usually, electrostatic interactions between AuNPs and melamine trigger the aggregation responsible for the color change, but recently, Xie et al. have explained the dehydrating condensation phenomena responsible to detect melamine.[Citation302]
In summary, a range of small organic toxic substances can be detected using modified or bare AuNPs. The detection based on target-AuNPs interactions are simple to follow but require case-by-case exploration because each analyte has different attractions with AuNPs, so this approach is case-specific. Reducing sugars can be detected with direct synthetic approach, but the detection time was as high as 60 minutes with a LOD in mM which needs an improvement. Moreover, this was a base-assisted synthesis which limits its applications under acidic conditions. In case of melamine detection, many colorimetric sensors have been established but there is a need to make this assay portable due to high demand of melamine detection in food on-spot.
Detection of biological analytes in environment and food
Biological pollutants are mainly living organisms that can pose serious health dangers to humans when present in aquatic and terrestrial environments.[Citation303] These bio-pollutants include bacteria, viruses, molds, and animal dander that cause many diseases such as indoor allergies, foodborne diseases, and bacterial and viral infections.[Citation304] These bio-analytes can be detected as a whole microorganism or specific nucleic acid sequences of a pathogen.[Citation305,Citation306] Of note, detection of different amino acid, peptides, proteins/enzymes, nucleic acids/oligonucleotides fall into the category of biomarkers which are important regulator to know the health conditions. AuNPs-based biosensors and immunosensors for the detection of different disease biomarkers have achieved many important milestones which is not the concern of this document and details can be found elsewhere.[Citation307,Citation308] Detection of different pathogens in water and food samples is important for environmental monitoring and food quality inspection.
Foodborne and waterborne pathogens have emerged as contagious species endangering human health from skin rash to life-threatening diseases including central nervous disorders, intestinal infections, pneumonia, endocarditis, osteomyelitis, sepsis, scalded skin syndrome, and toxic shock syndrome.[Citation309,Citation310] These pathogens found in contaminated poultry, meat, vegetables, fruits, eggs, and dairy products.[Citation311] AuNPs have been widely used for the detection of pathogens in water, food and environment.[Citation310] Mostly oligonucleotide functionalized AuNPs play a significant role in developing biosensors for microbial detection. The working principle of DNA-based nanosensors employing non-cross-linking mechanism of aggregation in modified AuNPs has been explained by He and coworkers.[Citation312] Also, Marin et al. have compiled a comprehensive review on point-of-need bacterial and their toxins detection present in food matrices using AuNPs.[Citation313]
The common recognition units for microbial detection are ssDNA probes, oligonucleotide, peptides, antibodies, bacteriophages, and aptamers. In DNA-based recognition system, the ssDNA adsorbs onto AuNPs through non-covalent interactions which make these particles stable against salt-induced aggregation in the absence of the target. However, salt-mediated aggregation of AuNPs happens with the target due to the formation of dsDNA which has little or no affinity toward AuNPs. Interestingly, Jiao et al. investigated the opposite behavior of DNA-based detection platform while assessing L-histidine.[Citation314] In this assay without histidine, DNA1 and DNA2 as histidine aptamers were partially hybridized to form a swing-like unique structure which helped the DNA duplex absorption on AuNPs and make them dispersed. L-histidine was determined with a LOD of 3.6 nM when sessile phosphodiester of the DNA1 was cleaved into two parts via self-cleavage due to catalytic action of histidine which disturbed the swing structure of duplex and created ssDNA segments. These cleaved ssDNA parts did not provide sufficient stability to AuNPs in concentrated salt media, and hence, the particles were aggregated resulting in blue color development.
Detection of microorganisms/pathogens
Pathogen’s detection is important to control foodborne and waterborne intoxication, resulting in continual advancements in colorimetric detection technology which offers easy-to-perform devices that intend for rapid point-of-need testing. Different shapes of AuNPs such as sphere, cluster, triangle, and hexagonal are involved in microbial detection which work through either LSPR phenomena or peroxidase-like catalytic behavior of AuNPs. Mostly, different biorecognition elements are adsorbed on AuNPs such as ssDNA, aptamers, antibodies, peptides, and bacteriophages to improve the sensitivity and specificity of bacterial detection system.[Citation313] Besides these biomolecular receptors, graphene oxide,[Citation315] iron oxide,[Citation316] citrate,[Citation317] and antibiotic-modified AuNPs[Citation318] have been employed for microorganisms detection.
It is well known that surface of bacteria is unique and considered negatively charged under physiological conditions.[Citation317] The surface electronic properties of bacterial cells have different interactions with AuNPs at different pH. Electrostatic repulsion develops between negatively charged bacterial surface and negative surface of AuNPs which creates a steric hindrance on the surface of bacteria, and resultantly, AuNPs remain dispersed in neutral or alkaline medium. Conversely, in acidic conditions, the surface of bacteria becomes positively charged which attracts negative AuNPs quickly via electrostatic attractive forces, and aggregation of nanoparticles can be observed by naked-eye as blue color. These pH-dependent interactions generate trigger-based aggregation in colorimetric aptasensors and would be responsible for selective bacterial detection. For example, Escherichia coli O157:H7 has been detected with thiolated-aptasensor HS-Apt@AuNPs probe using acidic to alkaline pH range as shown in [Citation144]. The detection limits of 40.46 cfu mL−1 with HCl, 94.08 cfu mL−1 with NaOH and 147.6 cfu mL−1 with MgCl2 were achieved in this assay. However, when simple citrate-AuNPs were used instead of aptamer bounded AuNPs, both the sensitivity and specificity were compromised. For example, seven different kinds of foodborne pathogens named Staphylococcus aureus, Vibrio parahaemolyticus, Shigella flexneri, Bacillus subtilis, Pseudomonas aeruginosa, E. coli O157:H7, and Salmonella typhimurium were detected with citrate capped AuNPs in LODs of 2.8 × 105 to 4.4 × 107 cfu mL−1 at a low pH ∼3.2 within 5 min.[Citation317]
Figure 10. Detection of different biological pollutants using AuNPs-based colorimetric sensors. (A) A pH-mediated detection of E. coli O157:H7 using thiol-aptamer functionalized AuNPs where acidic condition induced aggregation in AuNPs indicating the presence of bacteria whereas dispersion in AuNPs was observed in basic and neutral condition. Reproduced with permission from Ref. [Citation144] with some modifications. Copyright 2021 Elsevier; (B) detection of S. typhimurium using aptamer-gold nanoclusters (Apt-AuNCs) which showed an enhanced peroxidase-mimicking activity in the presence of bacteria as indicated by intense blue color. Reproduced with permission from Ref. [Citation319] with some modifications. Copyright 2021 Elsevier; C) detection of S. typhimurium using aptamer-thiolated polystyrene-cysteamine conjugates which reacted with Salmonella and aggregated AuNPs, resulting a colorimetric read-out whereas complementary DNA magnetic nanoparticle conjugates reacted with the free aptamer-PS-cysteamine conjugates in case of negative results. Reproduced with permission from Ref. [Citation311] with slight modifications. Copyright 2021 Elsevier; (D) detection of V. parahemolyticus using magnetic bead assisted sandwich immunoassay where polyclonal IgG antibodies coated MnO2 particles recognized the targeted bacteria liberating Mn2+ in the presence of ascorbic acid followed by AuNPs aggregation. Reproduced with permission from Ref.[Citation320] with slight modifications. Copyright 2018 American Chemical Society; (E) detection of S. aureus using AuNPs which were aggregated by Cu+ mediated alkyne-azide cycloaddition reaction. The aptamer-ALP-Fe3O4NPs (IMB) were used to separate targeted analyte. Blue color was observed in the absence of analyte because ascorbate-2-phosphate (AP) was hydrolyzed to ascorbic acid (AA) by ALP followed by reduction of Cu2+ to Cu1+ which triggered click reaction.[Citation321]
![Figure 10. Detection of different biological pollutants using AuNPs-based colorimetric sensors. (A) A pH-mediated detection of E. coli O157:H7 using thiol-aptamer functionalized AuNPs where acidic condition induced aggregation in AuNPs indicating the presence of bacteria whereas dispersion in AuNPs was observed in basic and neutral condition. Reproduced with permission from Ref. [Citation144] with some modifications. Copyright 2021 Elsevier; (B) detection of S. typhimurium using aptamer-gold nanoclusters (Apt-AuNCs) which showed an enhanced peroxidase-mimicking activity in the presence of bacteria as indicated by intense blue color. Reproduced with permission from Ref. [Citation319] with some modifications. Copyright 2021 Elsevier; C) detection of S. typhimurium using aptamer-thiolated polystyrene-cysteamine conjugates which reacted with Salmonella and aggregated AuNPs, resulting a colorimetric read-out whereas complementary DNA magnetic nanoparticle conjugates reacted with the free aptamer-PS-cysteamine conjugates in case of negative results. Reproduced with permission from Ref. [Citation311] with slight modifications. Copyright 2021 Elsevier; (D) detection of V. parahemolyticus using magnetic bead assisted sandwich immunoassay where polyclonal IgG antibodies coated MnO2 particles recognized the targeted bacteria liberating Mn2+ in the presence of ascorbic acid followed by AuNPs aggregation. Reproduced with permission from Ref.[Citation320] with slight modifications. Copyright 2018 American Chemical Society; (E) detection of S. aureus using AuNPs which were aggregated by Cu+ mediated alkyne-azide cycloaddition reaction. The aptamer-ALP-Fe3O4NPs (IMB) were used to separate targeted analyte. Blue color was observed in the absence of analyte because ascorbate-2-phosphate (AP) was hydrolyzed to ascorbic acid (AA) by ALP followed by reduction of Cu2+ to Cu1+ which triggered click reaction.[Citation321]](/cms/asset/45bca51b-428e-4337-b7ac-4aedbb652205/batc_a_2162331_f0010_c.jpg)
Instead of a direct trigger-mediated response, the detection of bacteria can also be achieved by indirect method which includes the enhancement of the peroxidase-like catalytic activity of nanogold in the presence of a target. For example, Chen et al. detected S. typhimurium recently through TMB-H2O2 system utilizing bovine serum albumin surrounded gold nanoclusters (BSA-AuNCs) as shown in .[Citation319] Basically, it was the enhancement of peroxidase-like activity of AuNPs after the addition of targeted bacteria resulting deeper blue color due to the formation of diamine and diimine charged complex. The surface of BSA-AuNCs was modified with dual aptamers via Au–S bonds of thiolated aptamers which made this detection system as sensitive as 1 cfu mL−1 which was comparable to an electrochemical sensor. This method was advantageous due to dual aptamer modified probe which occupied different binding sites on the surface of bacteria cell resulting higher sensitivity.
In another approach, aptamers were used in the detection system considering their high binding affinity toward a target. In this method, detection probe was consisted of aptamer-thiolated polystyrene-cysteamine conjugates that reacted with S. typhimurium target if present in the sample otherwise with complementary DNA-magnetic nanoparticle (cDNA-MNP) conjugates. AuNPs were introduced in the detection system after magnetic separation of nanoconjugates, where AuNPs were aggregated by reacting with sulfhydryl groups of cysteamine, giving a visible color change from red to blue in a positive sample with a LOD of 6.0 × 101 cfu mL−1. However, in case of negative sample, complementary DNA-aptamer conjugate had already been separated from the detection platform, so there was no thiolated polystyrene nanoconjugates in the solution, and hence the red color of AuNPs remained red as shown in .[Citation311] Complementary DNA approach without magnetic separation has also been used for K. pneumoniae detection with the help of thiolated oligonucleotide probes where DNA hybridization was the key principle of the detection.[Citation322] In another study, simultaneous detection of nineteen different Salmonella species was achieved by single stranded oligonucleotides 30-mer modified AuNPs through sandwich hybridization with 192-bases of ttrRSBCA of Salmonella strains with and LOD of <10 cfu mL−1.[Citation323]
As another AuNPs-based aptasensor strategy, etching process is widely used to detect a specific analyte and to enhance the method sensitivity. In case of detection, usually MnO2 nanoparticles serve the purpose while iron oxide nanoparticles are used when signal amplification is required to enhance the activity via etching process. However, in both scenario, microorganism detection requires immunomagnetic separation technology, where the binding of recognition antibodies and target bacteria leads to sandwich-type immunocomplex. The MnO2 nanoparticles on this immunocomplex is etched with a reducing agent to generate Mn2+ ions that would cause aggregation in AuNPs. For example, detection of Vibrio parahemolyticus was achieved by IgY-MBs and IgG-MnO2 NPs that bound to different specific binding sites of bacteria through antigen − antibody interactions. After a magnetic separation, the bacteria-attached MnO2 NPs were etched by ascorbic acid to produce excess Mn2+ ions which led to aggregation in AuNPs through metal-ligand interaction as shown in .[Citation320] The amount of Mn2+ ions generated was directly proportional to the bacterial concentration, which gave a LOD of 10 cfu mL−1 for this method. In case of etching-enhanced peroxidase activity of aptamer-AuNPs, Staphylococcus aureus was detected with and LOD of 10 cfu mL−1.[Citation316] In this system, IgY antibodies modified Fe3O4/Au nanocomposite were used to separate target bacteria, and thiol-aptamer-AuNPs showed peroxidase-like catalytic activity which was revealed by the TMB-H2O2 reporting agent. Deep yellow color changed to pale yellow with the increased concentrations of S. aureus. In short, etching process can be used either to reveal or enhance the colorimetric detection of pathogens in AuNPs-based immunoseparation assay.
In addition to aptasensors, Gram-positive and Gram-negative bacteria can be detected with chemosensors utilizing the interactions of antibiotics with bacterial cell wall; hence antibiotic modified AuNPs probe has been synthesized. Recently, polypeptide and β-lactam structural features of antibiotics have been used to reduce gold salt to AuNPs in addition to their sensing properties for different bacterial species like S. aureus, P. aeruginosa, and E. coli.[Citation324] Polymyxin, bacitracin, penicillin, and cephalexin antibiotics have produced spherical, hexagonal and triangle shaped AuNPs in different sizes and population. The detection was based on the interaction between specific binding sites of bacterial membrane and variable functional groups of antibiotics. The LOD was not determined for any bacterial species which needs further exploration. Similarly, vancomycin-AuNPs probe was used to detect S. aureus, M. luteus, and B. subtilis at 1 × 109 cells mL−1 on a test strip which differentiated Gram-positive bacteria from Gram-negative strains.[Citation318] Vancomycin-AuNPs and Gram-positive bacterial cell suspension showed the red color on a nitrocellulose strip which became deepened with increasing bacterial concentrations. Silver treatment for the signal enhancement was applied which changed red color to black due to reduction of silver ions on the Au surface. The bare eyes detection limit for S. aureus was ∼2 × 109 without treatment and ∼1 × 109 cells mL−1 after Ag enhancement. No significant change in LOD was observed for M. luteus, and B. subtilis. Liu et al. reported the detection of a food born pathogen S. aureus with a LOD of 2.4 cfu mL−1 using a click reaction between azides-AuNPs and alkyne-AuNPs.[Citation321] The aptamer and ALP dual functionalized Fe3O4NPs were used as recognition as well as signal transduction agent. The ascorbate-2-phosphate (AP) was catalyzed by ALP to generate ascorbic acid (AA). The AA reduced Cu2+ present in the solution to Cu+ which triggered a click reaction for azide- and alkyne AuNPs, resulting in the aggregation of AuNPs with the development of blue color as shown in . In the presence of S. aureus, the binding of Fe3O4NPs to the bacterial surface inhibited the substrate to show the peroxidase-like catalytic performance, hence, stopping the colorimetric sensing of bacteria resulting in red color of the solution.
Conclusively, AuNPs-based biosensors have been used for the detection of pathogenic bacteria through target-stimulated approach, enhancement of peroxidase activity, thiolated polystyrene-analyte interactions, DNA hybridization and antibody-antigen interactions. Among all, target-mediated approach is simple and inexpensive but very sensitive to pH changes which requires a careful assay handling. Detection through enhancement of peroxidase-activity is a more sensitive approach as compared to all other methods due to involvement of peroxidase-like catalytic role of AuNPs which makes detection system highly sensitive and efficient. While in case of thiolated polystyrene-analyte interactions and antibody-antigen interactions, immunomagnetic separation is involved which is time consuming (∼45–60 min) and makes detection protocols cumbersome. These AuNPs-based aptasensors are highly specific toward bacterial strains as compared to chemosensors based on antibiotic-AuNPs. AuNPs-based antibiotic assisted bacterial detection system is complex due to involvement of multiple amine, amide and carboxylic groups in structure which are acid and base sensitive moieties and require a good control over pH. However, this approach is equally suitable for a variety of Gram-positive and Gram-negative bacteria due to bacterial cell wall interactions with compatible antibiotics. Moreover, silver enhancement protocols in these chemosensors help to increase the color intensity of results which make this procedure favorable over other detection systems.
Summary
The prevalence of AuNPs in scientific research and nanotechnology has been admitted without any debate. The unique SPR and peroxidase-like catalytic properties of AuNPs varies with particle size, geometric shape, and surface chemistry. This comprehensive review presents the main characteristics and synthetic approaches of AuNPs as well as a detailed comparative discussion on different sensing mechanisms of colorimetric detection. Analytes involved in environmental monitoring and food quality control have been broadly categorized into inorganic, organic, and biological sections. Of note, physical properties of AuNPs are controlled by their method of preparation, while the detection method is dependent on the nature of functional groups adhered to AuNPs surface. It is noteworthy to mention that the focus of this review paper is on analytics, device performance and key findings of assay including the nature of functional groups around AuNPs, chemical forces involved, experimental conditions of method, assay time, and limit of detection for the real samples. This review paper is different from other published work on application to environmental and food analysis.
Among AuNPs-related SPR-based colorimetric sensors, the non-crosslinking aggregation method is sensitive, quick, and simple as analyte-triggered destabilization of AuNPs involves only a few steps. However, this method is prone to interference with non-targeted agents and thus has relatively low selectivity. In comparison, the cross-linking aggregation method is less sensitive, and more time-consuming due to the involvement of true chemical bonds by chemical reactions, but it has a higher selectivity. Moreover, salt-induced signal amplification is successful in case of non-crosslinking aggregation, whereas cross-linking aggregation requires additional chelating agents or enzymes to achieve lower limit of detection. Etching-based non-aggregation methods are more sensitive compared to aggregation methods but require harsh conditions such as the use of oxidizing agents or corrosion chemicals. Moreover, etching being a slow process takes longer detection time.
AuNPs-related peroxidase activity-based colorimetric sensors are more sensitive as compared to SPR-based colorimetric sensors due to involvement of peroxidase-like catalytic properties of AuNPs which offers detection limits usually in nM and pM level. However, nanozyme sensors require lengthy detection protocols. In this regard, expression of peroxidase activity method is more efficient and is better studied than the method relying on inhibition of peroxidase activity given to less prevalence of inhibiting agents. Bimetallic nanocomposites offer higher peroxidase-like catalytic activity as compared to ligand modified AuNPs. Although ligand attachment on the surface of particles alters the enzyme mimicking activity of AuNPs, this modification usually detects single analyte, and hence multiplexed identification of analytes is highly challenging in nanozyme sensors as multiplexing capabilities of AuNPs is difficult to achieve. Nanoscale size <10 nm of AuNPs shows better peroxidase-like catalytic activity which is further improved when particle size drops below ∼3 nm, while detection systems work well in terms of sensitivity when AuNPs >10 nm are used in SPR-based colorimetric sensors. Overall, AuNPs-colorimetric sensors are less explored as compared to electrochemical sensors in the area of inorganic ions detection. Chemosensors are widely used for the detection of organic analytes, whereas biosensors are frequently employed for the detection of bio-analytes.
Future perspectives
Worldwide research on AuNPs to construct colorimetric sensors highlights a few points to consider in future. Besides distinct advancement in sensors and detection field, only a few commercial devices using AuNPs are available for a limited variety of analytes. With rapid increase in pollution crises and food frauds, it is crucial to develop colorimetric sensors for on-spot inexpensive testing having rapid results without the need of any instrument, especially in resource-limited areas. Thus, considerable efforts have been made to explore new strategies for the detection of pollutants and toxic substances, as well as to tackle the current challenges associated with existing sensing approaches. Of note, the prevalence of AuNPs in environmental monitoring and food safety has been admitted since decades but further exploration is required to implement signal amplification approaches to improve the sensitivity by naked eye readout. In addition to this, it is necessary to identify more aptamers for designing new aptasensors as aptamers are highly specific to targeted analyte and have higher stability compared to antibodies.
Currently, nanozyme sensors work at a narrow pH range which needs to be broadened to enhance the versatility of nanozyme platform. The disadvantage of nanozyme sensors is the longer detection time because of slow enzyme kinetics which needs to be improved by introducing new functionalities on AuNPs surface. Moreover, it is important to work on less-explored corner of catalytic properties of AuNPs such as catalase, oxidase, and reductase. Besides, analytes in complex food matrix are challenging for on-spot colorimetric detection which require the establishment of in-field sample separation techniques. Moreover, colorimetric reactions usually happen in the solution phase which are susceptible to external environment, and hence, reagent encapsulation or lyophilization strategies should be emphasized for portable detection systems.
Sample matrix interference on the performance of AuNPs-based assays should be considered since AuNPs are vulnerable to aggregation in the presence of sample matrix. In some cases, sample digestion is required to eliminate the effect of a complex sample matrix, but this step increases the acidity or alkalinity of the media which may cause unwanted aggregation of unprotected AuNPs. In another approach, pre-separation of the target analyte from sample via column-based pre-concentration or solvent extraction can alleviate matrix interferences, but such steps are tedious and add complexity in the operation. Alternatively, simple dilution can be used to limit the matrix effect. However, dilution can be applied to selected samples and will decrease the concentration of the analyte in the sample, making it difficult to detect the analyte in low concentrations. Till today, the common approach to avoid interferences is to prepare AuNPs probe with appropriate functionalization which is being used by many researchers.
Overall, colorimetric detection is sensitive to colloidal stability which needs to be improved for better quantification purposes. Morphology of particles govern the analyte sensing capacity, so reproducible synthetic approaches for AuNPs are encouraged as homogeneity and stability of large batch of AuNPs is difficult to achieve. Single AuNP can be functionalized with multiple ligands to fabricate multiplex detection devices. Altogether, AuNPs are considered smart materials in sensors technology due to strong potential applications in environmental monitoring and food applications. AuNPs-based colorimetric sensors could lead to tackle upcoming challenges regarding pollution removal, wastewater treatment, and food safety. The development of state-of-the-art microfluidic devices such as paper-based microfluidic devices using AuNPs, and gold nanocomposites may improve the applicability and sensitivity of established sensors. Active collaboration among researchers is encouraged in the field of sensors, detection, nanomaterials, and computational to advance the AuNPs research. Moreover, improving the stability and reproducibility profile of AuNPs will maximize its utilities in food quality monitoring and pollution detection toward commercialization.
Supplemental Material
Download MS Word (146.3 KB)Acknowledgements
This research received no specific financial support except funding from the Natural Sciences and Engineering Research Council of Canada (NSERC) through a discovery grant, CURC university research chair fund, and the Concordia FRDP grant for development of point-of-care analytical devices for medical diagnostic and environmental application. The funders had no role in data collection, interpretation, or the decision to submit the work for publication.
Disclosure statement
There are no conflicts to declare.
References
- Louis, C.; Pluchery, O. Gold Nanoparticles for Physics, Chemistry and Biology; Imperial College Press: London, 2012. DOI: 10.1142/p815.
- Dekker, F.; Kool, L.; Bunschoten, A.; Velders, A. H.; Saggiomo, V. Syntheses of Gold and Silver Dichroic Nanoparticles; Looking at the Lycurgus Cup Colors. Chem. Teach. Int. 2021, 3, 1–6. DOI: 10.1515/cti-2019-0011.
- Faraday, M. Experimental Relations o f Gold (and Other Metals) to Light. Philos. Trans. R. Soc. London 1857, 147, 145–181. DOI: 10.1098/rstl.1857.0011.
- Letchumanan, I.; Gopinath, S. C. B.; Md Arshad, M. K.; Mohamed Saheed, M. S.; Perumal, V.; Voon, C. H.; Hashim, U. Gold-Nanohybrid Biosensors for Analyzing Blood Circulating Clinical Biomacromolecules: Current Trend toward Future Remote Digital Monitoring. Crit. Rev. Anal. Chem. 2022, 52, 577–592. DOI: 10.1080/10408347.2020.1812373.
- De, A.; Kalita, D. Bio-Fabricated Gold and Silver Nanoparticle Based Plasmonic Sensors for Detection of Environmental Pollutants: An Overview. Crit. Rev. Anal. Chem. 2021. DOI: 10.1080/10408347.2021.1970507.
- Daruich De Souza, C.; Ribeiro Nogueira, B.; Rostelato, M. E. C. M. Review of the Methodologies Used in the Synthesis Gold Nanoparticles by Chemical Reduction. J. Alloy. Compd. 2019, 798, 714–740. DOI: 10.1016/j.jallcom.2019.05.153.
- Qin, L.; Zeng, G.; Lai, C.; Huang, D.; Xu, P.; Zhang, C.; Cheng, M.; Liu, X.; Liu, S.; Li, B.; et al. “Gold Rush” in Modern Science: Fabrication Strategies and Typical Advanced Applications of Gold Nanoparticles in Sensing. Coord. Chem. Rev. 2018, 359, 1–31. DOI: 10.1016/j.ccr.2018.01.006.
- Giljohann, D. A.; Seferos, D. S.; Daniel, W. L.; Massich, M. D.; Patel, P. C.; Mirkin, C. A. Gold Nanoparticles for Biology and Medicine. Angew. Chem. Int. Ed. Engl. 2010, 49, 3280–3294. DOI: 10.1002/anie.200904359.
- Li, J. y.; Zhu, J.; Weng, G. j.; Li, J. j.; Zhao, J. w Multiplex Sensing Based on Plasmonic Optics of Noble Metallic Nanostructures. Crit. Rev. Anal. Chem. 2022. DOI: 10.1080/10408347.2022.2122692.
- Venugopalan, P. L.; Esteban-Fernández De Ávila, B.; Pal, M.; Ghosh, A.; Wang, J. Fantastic Voyage of Nanomotors into the Cell. ACS Nano 2020, 14, 9423–9439. DOI: 10.1021/acsnano.0c05217.
- Ameku, W. A.; De Araujo, W. R.; Rangel, C. J.; Ando, R. A.; Paixão, T. R. L. C. Gold Nanoparticle Paper-Based Dual-Detection Device for Forensics Applications. ACS Appl. Nano Mater. 2019, 2, 5460–5468. DOI: 10.1021/acsanm.9b01057.
- Hua, Z.; Yu, T.; Liu, D.; Xianyu, Y. Recent Advances in Gold Nanoparticles-Based Biosensors for Food Safety Detection. Biosens. Bioelectron. 2021, 179, 113076. DOI: 10.1016/j.bios.2021.113076.
- Wilson, R. The Use of Gold Nanoparticles in Diagnostics and Detection. Chem. Soc. Rev. 2008, 37, 2028–2045. DOI: 10.1039/b712179m.
- Draz, M. S.; Shafiee, H. Applications of Gold Nanoparticles in Virus Detection. Theranostics 2018, 8, 1985–2017. DOI: 10.7150/thno.23856.
- Mie, G. Beitrage Zur Optik Truber Medien Speziell Kolloidaler Goldlosungen. Ann. Phys. 1908, 330, 377–445. DOI: 10.1002/andp.19083300302.
- Horvath, H. Gustav Mie and the Scattering and Absorption of Light by Particles: Historic Developments and Basics. J. Quant. Spectrosc. Radiat. Transf. 2009, 110, 787–799. DOI: 10.1016/j.jqsrt.2009.02.022.
- Turkevich, J.; Stevenson, P. C.; Hillier, J. A Study of the Nucleation and Growth Processes in the Synthesis of Colloidal Gold. Discuss. Faraday Soc. 1951, 11, 55. DOI: 10.1039/df9511100055.
- Feynman, R. P. There’s Plenty of Room at the Bottom (Reprint from Speech Given at Annual Meeting of the American Physical Society). Eng. Sci. 1960, 23, 22–36.
- Faulk, W. P.; Taylor, G. M. An Immunocolloid Method for the Electron Microscope. Immunochemistry 1971, 8, 1081–1083. DOI: 10.1016/0019-2791(71)90496-4.
- Frens, G. Controlled Nucleation for the Regulation of the Particle Size in Monodisperse Gold Suspensions. Nat. Phys. Sci. 1973, 241, 20–22. DOI: 10.1038/physci241020a0.
- Brust, M.; Walker, M.; Bethell, D.; Schiffrin, D. J.; Whyman, R. Synthesis of Thiol-Derivatised Gold Nanoparticles in a Two-Phase Liquid-Liquid System. J. Chem. Soc., Chem. Commun. 1994, 0, 801–802. DOI: 10.1039/C39940000801.
- Alivisatos, A. P.; Johnsson, K. P.; Peng, X.; Wilson, T. E.; Loweth, C. J.; Bruchez, M. P.; Schultz, P. G. Organization of “Nanocrystal Molecules” Using DNA. Nature 1996, 382, 609–611. DOI: 10.1038/382609a0.
- Mirkin, C. A.; Letsinger, R. L.; Mucic, R. C.; Storhoff, J. J. A DNA-Based Method for Rationally Assembling Nanoparticles into Macroscopic Materials. Nature 1996, 382, 607–609. DOI: 10.1038/382607a0.
- Li, H.; Rothberg, L. J. Label-Free Colorimetric Detection of Specific Sequences in Genomic DNA Amplified by the Polymerase Chain Reaction. J. Am. Chem. Soc. 2004, 126, 10958–10961. DOI: 10.1021/ja048749n.
- Velusamy, P.; Su, C. H.; Ramasamy, P.; Arun, V.; Rajnish, N.; Raman, P.; Baskaralingam, V.; Senthil Kumar, S. M.; Gopinath, S. C. B. Volatile Organic Compounds as Potential Biomarkers for Noninvasive Disease Detection by Nanosensors: A Comprehensive Review. Crit. Rev. Anal. Chem. 2022. DOI: 10.1080/10408347.2022.2043145.
- Rawtani, D.; Tharmavaram, M.; Pandey, G.; Hussain, C. M. Functionalized Nanomaterial for Forensic Sample Analysis. TrAC – Trends Anal. Chem. 2019, 120, 115661. DOI: 10.1016/j.trac.2019.115661.
- Oyewunmi, O. D.; Safiabadi-Tali, S. H.; Jahanshahi-Anbuhi, S. Dual-Modal Assay Kit for the Qualitative and Quantitative Determination of the Total Water Hardness Using a Permanent Marker Fabricated Microfluidic Paper-Based Analytical Device. Chemosensors 2020, 8, 97. DOI: 10.3390/chemosensors8040097.
- Safiabadi Tali, S. H.; LeBlanc, J. J.; Sadiq, Z.; Oyewunmi, O. D.; Camargo, C.; Nikpour, B.; Armanfard, N.; Sagan, S. M.; Jahanshahi-Anbuhi, S. Tools and Techniques for Severe Acute Respiratory Syndrome Coronavirus 2 (SARS-CoV-2)/COVID-19 Detection. Clin. Microbiol. Rev. 2021, 34, 1–63. DOI: 10.1128/CMR.00228-20.
- Li, Y.; Wang, Z.; Sun, L.; Liu, L.; Xu, C.; Kuang, H. Nanoparticle-Based Sensors for Food Contaminants. TrAC – Trends Anal. Chem. 2019, 113, 74–83. DOI: 10.1016/j.trac.2019.01.012.
- Saha, K.; Agasti, S. S.; Kim, C.; Li, X.; Rotello, V. M. Gold Nanoparticles in Chemical and Biological Sensing. Chem. Rev. 2012, 112, 2739–2779. DOI: 10.1021/cr2001178.
- Elahi, N.; Kamali, M.; Baghersad, M. H. Recent Biomedical Applications of Gold Nanoparticles: A Review. Talanta 2018, 184, 537–556. DOI: 10.1016/j.talanta.2018.02.088.
- Hong, X.; Bai, J.; Peng, Y.; Zhang, X.; Gao, Z.; Ning, B.; Li, M.; Pu, Y.; Ying, Y.; Su, F.; et al. Au-Doped Photonic Crystal Allows Naked-Eye Determination of Small Organic Molecules. Sens. Actuators B Chem. 2020, 321, 128493. DOI: 10.1016/j.snb.2020.128493.
- Xiao, W.; Deng, Z.; Huang, J.; Huang, Z.; Zhuang, M.; Yuan, Y.; Nie, J.; Zhang, Y. Highly Sensitive Colorimetric Detection of a Variety of Analytes via the Tyndall Effect. Anal. Chem. 2019, 91, 15114–15122. DOI: 10.1021/acs.analchem.9b03824.
- Sánchez-Visedo, A.; Gallego, B.; Royo, L. J.; Soldado, A.; Valledor, M.; Ferrero, F. J.; Campo, J. C.; Costa-Fernández, J. M.; Fernández-Argüelles, M. T. Visual Detection of MicroRNA146a by Using RNA-Functionalized Gold Nanoparticles. Mikrochim. Acta 2020, 187, 192. DOI: 10.1007/s00604-020-4148-4.
- Dong, T.; Ma, X.; Sheng, N.; Qi, X.; Chu, Y.; Song, Q.; Zou, B.; Zhou, G. Point-of-Care DNA Testing by Automatically and Sequentially Performing Extraction, Amplification and Identification in a Closed-Type Cassette. Sens. Actuators B Chem. 2021, 327, 128919. DOI: 10.1016/j.snb.2020.128919.
- Li, L.; Liang, Y.; Zhao, Y.; Chen, Z. Target Binding and DNA Hybridization-Induced Gold Nanoparticle Aggregation for Colorimetric Detection of Thrombin. Sens. Actuators B Chem. 2018, 262, 733–738. DOI: 10.1016/j.snb.2018.02.061.
- Giorgi-Coll, S.; Marín, M. J.; Sule, O.; Hutchinson, P. J.; Carpenter, K. L. H. Aptamer-Modified Gold Nanoparticles for Rapid Aggregation-Based Detection of Inflammation: An Optical Assay for Interleukin-6. Microchim. Acta 2020, 187. DOI: 10.1007/s00604-019-3975-7.
- Gul, Z.; Ullah, S.; Khan, S.; Ullah, H.; Khan, M. U.; Ullah, M.; Ali, S.; Altaf, A. A. Recent Progress in Nanoparticles Based Sensors for the Detection of Mercury (II) Ions in Environmental and Biological Samples. Crit. Rev. Anal. Chem. 2022. DOI: 10.1080/10408347.2022.2049676.
- Ali, S.; Chen, X.; Shi, W.; Huang, G.; Yuan, L. m.; Meng, L.; Chen, S.; Zhonghao, X.; Chen, X. Recent Advances in Silver and Gold Nanoparticles-Based Colorimetric Sensors for Heavy Metal Ions Detection: A Review. Crit. Rev. Anal. Chem. 2021. DOI: 10.1080/10408347.2021.1973886.
- Wang, F.; Lu, Y.; Chen, Y.; Sun, J.; Liu, Y. Colorimetric Nanosensor Based on the Aggregation of AuNP Triggered by Carbon Quantum Dots for Detection of Ag+ Ions. ACS Sustain. Chem. Eng. 2018, 6, 3706–3713. DOI: 10.1021/acssuschemeng.7b04067.
- Cordeiro, M.; Carlos, F. F.; Pedrosa, P.; Lopez, A.; Baptista, P. V. Gold Nanoparticles for Diagnostics: Advances towards Points of Care. Diagnostics 2016, 6, 43. DOI: 10.3390/diagnostics6040043.
- Almeida, M. I. G. S.; Jayawardane, B. M.; Kolev, S. D.; McKelvie, I. D. Developments of Microfluidic Paper-Based Analytical Devices (ΜPADs) for Water Analysis: A Review. Talanta 2018, 177, 176–190. DOI: 10.1016/j.talanta.2017.08.072.
- Kung, C.; Te; Hou, C. Y.; Wang, Y. N.; Fu, L. M. Microfluidic Paper-Based Analytical Devices for Environmental Analysis of Soil, Air, Ecology and River Water. Sens. Actuators B Chem. 2019, 301, 126855. DOI: 10.1016/j.snb.2019.126855.
- Lapresta-Fernández, A.; Fernández, A.; Blasco, J. Nanoecotoxicity Effects of Engineered Silver and Gold Nanoparticles in Aquatic Organisms. TrAC - Trends Anal. Chem. 2012, 32, 40–59. DOI: 10.1016/j.trac.2011.09.007.
- Rinaldi, S.; Tarpani, L.; Latterini, L. UV Treatment of the Stabilizing Shell for Improving the Photostability of Silver Nanoparticles. J. Nanomater. 2016, 2016, 1–7. DOI: 10.1155/2016/7510563.
- Lin, X.; Zhu, Z.; Zhao, C.; Li, S.; Liu, Q.; Liu, A.; Lin, L.; Lin, X. Robust Oxidase Mimicking Activity of Protamine-Stabilized Platinum Nanoparticles Units and Applied for Colorimetric Sensor of Trypsin and Inhibitor. Sens. Actuators B Chem. 2019, 284, 346–353. DOI: 10.1016/j.snb.2018.12.109.
- Sun, L.; Fu, Z.; Ma, E.; Guo, J.; Zhang, Z.; Li, W.; Li, L.; Liu, Z.; Guo, X. Ultrasmall Pt Nanozymes Immobilized on Spherical Polyelectrolyte Brushes with Robust Peroxidase-like Activity for Highly Sensitive Detection of Cysteine. Langmuir 2022, 38, 12915–12923. DOI: 10.1021/acs.langmuir.2c02056.
- Sriram, G.; Bhat, M. P.; Patil, P.; Uthappa, U. T.; Jung, H.-Y.; Altalhi, T.; Kumeria, T.; Aminabhavi, T. M.; Pai, R. K.; Kurkuri, M. D.; et al. Paper-Based Microfluidic Analytical Devices for Colorimetric Detection of Toxic Ions: A Review. TrAC – Trends Anal. Chem. 2017, 93, 212–227. DOI: 10.1016/j.trac.2017.06.005.
- Shah, M.; Badwaik, V.; Kherde, Y.; Waghwani, H. K.; Modi, T.; Aguilar, Z. P.; Rodgers, H.; Hamilton, W.; Marutharaj, T.; Webb, C.; et al. Gold Nanoparticles: Various Methods of Synthesis and Antibacterial Applications. Front. Biosci. (Landmark Ed))2014, 19, 1320–1344. DOI: 10.2741/4284.
- Sardar, R.; Funston, A. M.; Mulvaney, P.; Murray, R. W. Gold Nanoparticles: Past, Present, and Future. Langmuir 2009, 25, 13840–13851. DOI: 10.1021/la9019475.
- Sun, S.; Mendes, P.; Critchley, K.; Diegoli, S.; Hanwell, M.; Evans, S. D.; Leggett, G. J.; Preece, J. A.; Richardson, T. H. Fabrication of Gold Micro- and Nanostructures by Photolithographic Exposure of Thiol-Stabilized Gold Nanoparticles. Nano Lett. 2006, 6, 345–350. DOI: 10.1021/nl052130h.
- Tangeysh, B.; Moore Tibbetts, K.; Odhner, J. H.; Wayland, B. B.; Levis, R. J. Gold Nanoparticle Synthesis Using Spatially and Temporally Shaped Femtosecond Laser Pulses: Post-Irradiation Auto-Reduction of Aqueous [AuCl4]. J. Phys. Chem. C 2013, 117, 18719–18727. DOI: 10.1021/jp4056494.
- Birtcher, R. C.; Kirk, M. A.; Furuya, K.; Lumpkin, G. R.; Ruault, M.-O. In Situ Transmission Electron Microscopy Investigation of Radiation Effects. J. Mater. Res. 2005, 20, 1654–1683. DOI: 10.1557/JMR.2005.0242.
- Wuithschick, M.; Birnbaum, A.; Witte, S.; Sztucki, M.; Vainio, U.; Pinna, N.; Rademann, K.; Emmerling, F.; Kraehnert, R.; Polte, J. Turkevich in New Robes: Key Questions Answered for the Most Common Gold Nanoparticle Synthesis. ACS Nano 2015, 9, 7052–7071. DOI: 10.1021/acsnano.5b01579.
- Yang, X.; Yang, M.; Pang, B.; Vara, M.; Xia, Y. Gold Nanomaterials at Work in Biomedicine. Chem. Rev. 2015, 115, 10410–10488. DOI: 10.1021/acs.chemrev.5b00193.
- Wieckowska, A.; Dzwonek, M. Ultrasmall Au Nanoparticles Coated with Hexanethiol and Anthraquinone/Hexanethiol for Enzyme-Catalyzed Oxygen Reduction. Sens. Actuators B Chem. 2016, 224, 514–520. DOI: 10.1016/j.snb.2015.10.073.
- Sardar, R.; Shumaker-Parry, J. S. 9-BBN Induced Synthesis of Nearly Monodisperse ω-Functionalized Alkylthiol Stabilized Gold Nanoparticles. Chem. Mater. 2009, 21, 1167–1169. DOI: 10.1021/cm802942x.
- Gupta, R. K.; Srinivasan, M. P.; Dharmarajan, R. Synthesis of Short Chain Thiol Capped Gold Nanoparticles, Their Stabilization and Immobilization on Silicon Surface. Colloids Surf. A Physicochem. Eng. Asp 2011, 390, 149–156. DOI: 10.1016/j.colsurfa.2011.09.019.
- Leontowich, A. F. G.; Calver, C. F.; Dasog, M.; Scott, R. W. J. Surface Properties of Water-Soluble Glycine-Cysteamine-Protected Gold Clusters. Langmuir 2010, 26, 1285–1290. DOI: 10.1021/la902465b.
- Zaluzhna, O.; Li, Y.; Zangmeister, C.; Allison, T. C.; Tong, Y. Y. J. Mechanistic Insights on One-Phase vs. Two-Phase Brust-Schiffrin Method Synthesis of Au Nanoparticles with Dioctyl-Diselenides. Chem. Commun. (Camb) 2012, 48, 362–364. DOI: 10.1039/c1cc15955k.
- Wiesner, J.; Wokaun, A. Anisometric Gold Colloids. Preparation, Characterization, and Optical Properties. Chem. Phys. Lett. 1989, 157, 569–575. DOI: 10.1016/S0009-2614(89)87413-5.
- Yang, Y. C.; Wang, C. H.; Hwu, Y. K.; Je, J. H. Synchrotron X-Ray Synthesis of Colloidal Gold Particles for Drug Delivery. Mater. Chem. Phys. 2006, 100, 72–76. DOI: 10.1016/j.matchemphys.2005.12.007.
- Akar, B.; Pushpavanam, K.; Narayanan, E.; Rege, K.; Heys, J. J. Mechanistic Investigation of Radiolysis-Induced Gold Nanoparticle Formation for Radiation Dose Prediction. Biomed. Phys. Eng. Express 2018, 4, 065011. DOI: 10.1088/2057-1976/aac280.
- Abdelghany, A. M.; Abdelrazek, E. M.; Badr, S. I.; Abdel-Aziz, M. S.; Morsi, M. A. Effect of Gamma-Irradiation on Biosynthesized Gold Nanoparticles Using Chenopodium Murale Leaf Extract. J. Saudi Chem. Soc. 2017, 21, 528–537. DOI: 10.1016/j.jscs.2015.10.002.
- Hanžić, N.; Jurkin, T.; Maksimović, A.; Gotić, M. The Synthesis of Gold Nanoparticles by a Citrate-Radiolytical Method. Radiat. Phys. Chem. 2015, 106, 77–82. DOI: 10.1016/j.radphyschem.2014.07.006.
- Chen, S.; Liu, Y.; Wu, G. Stabilized and Size-Tunable Gold Nanoparticles Formed in a Quaternary Ammonium-Based Room-Temperature Ionic Liquid under γ-Irradiation. Nanotechnology 2005, 16, 2360–2364. DOI: 10.1088/0957-4484/16/10/061.
- Li, X.; Liu, L.; Xu, Z.; Wang, W.; Shi, J.; Liu, L.; Jing, M.; Li, F.; Zhang, X. Gamma Irradiation and Microemulsion Assisted Synthesis of Monodisperse Flower-like Platinum-Gold Nanoparticles/Reduced Graphene Oxide Nanocomposites for Ultrasensitive Detection of Carcinoembryonic Antigen. Sens. Actuators B Chem. 2019, 287, 267–277. DOI: 10.1016/j.snb.2019.02.026.
- Fan, C.; Li, W.; Zhao, S.; Chen, J.; Li, X. Efficient One Pot Synthesis of Chitosan-Induced Gold Nanoparticles by Microwave Irradiation. Mater. Lett. 2008, 62, 3518–3520. DOI: 10.1016/j.matlet.2008.03.040.
- Ngo, V. K. T.; Nguyen, D. G.; Huynh, T. P.; Lam, Q. V. A Low Cost Technique for Synthesis of Gold Nanoparticles Using Microwave Heating and Its Application in Signal Amplification for Detecting Escherichia coli O157:H7 Bacteria. Adv. Nat. Sci. Nanosci. Nanotechnol. 2016, 7, 035016. DOI: 10.1088/2043-6262/7/3/035016.
- El-Naggar, M. E.; Shaheen, T. I.; Fouda, M. M. G.; Hebeish, A. A. Eco-Friendly Microwave-Assisted Green and Rapid Synthesis of Well-Stabilized Gold and Core–Shell Silver–Gold Nanoparticles. Carbohydr. Polym. 2016, 136, 1128–1136. DOI: 10.1016/j.carbpol.2015.10.003.
- Morad, M.; Karim, M. A.; Altass, H. M.; Khder, A. E. R. S. Microwave-Assisted Synthesis of Gold Nanoparticles Supported on Mn3O4 Catalyst for Low Temperature CO Oxidation. Environ. Technol. 2021, 42, 2680–2689. DOI: 10.1080/09593330.2019.1709988.
- Alle, M.; Lee, S. H.; Kim, J. C. Ultrafast Synthesis of Gold Nanoparticles on Cellulose Nanocrystals via Microwave Irradiation and Their Dyes-Degradation Catalytic Activity. J. Mater. Sci. Technol. 2020, 41, 168–177. DOI: 10.1016/j.jmst.2019.11.003.
- Chin, C. D. W.; Treadwell, L. J.; Wiley, J. B. Microwave Synthetic Routes for Shape-Controlled Catalyst Nanoparticles and Nanocomposites. Molecules 2021, 26, 3647–3616. DOI: 10.3390/molecules26123647.
- Rodríguez-Torres, M. D. P.; Diaz-Torres, L. A.; Olmos-López, M.; Salas, P.; Gutiérrez, C. UVA Mediated Synthesis of Gold Nanoparticles in Pharmaceutical-Grade Heparin Sodium Solutions. Plasmonics: Metallic Nanostruct. Their Opt. Prop. XI 2013, 8809, 88092R. DOI: 10.1117/12.2024441.
- Braguer, D.; Correard, F.; Maximova, K.; Villard, C.; Roy, M.; Al-Kattan, A.; Sentis, M.; Gingras, M.; Kabashin, A.; Esteve, M.-A. Gold Nanoparticles Prepared by Laser Ablation in Aqueous Biocompatible Solutions: Assessment of Safety and Biological Identity for Nanomedicine Applications. Int. J. Nanomed. 2014, 9, 5415–5430. DOI: 10.2147/IJN.S65817.
- Tiyyagura, H. R.; Majerič, P.; Anžel, I.; Rudolf, R. Low-Cost Synthesis of AuNPs through Ultrasonic Spray Pyrolysis. Mater. Res. Express 2020, 7, 055017. DOI: 10.1088/2053-1591/ab80ea.
- Gao, L.; Mei, S.; Ma, H.; Chen, X. Ultrasound-Assisted Green Synthesis of Gold Nanoparticles Using Citrus Peel Extract and Their Enhanced anti-Inflammatory Activity. Ultrason. Sonochem. 2022, 83, 105940. DOI: 10.1016/j.ultsonch.2022.105940.
- Vo, K. D. N.; Kowandy, C.; Dupont, L.; Coqueret, X.; Hien, N. Q. Radiation Synthesis of Chitosan Stabilized Gold Nanoparticles Comparison between E- Beam and γ Irradiation. Radiat. Phys. Chem. 2014, 94, 84–87. DOI: 10.1016/j.radphyschem.2013.04.015.
- Kumar, A.; Bhatt, M.; Vyas, G.; Bhatt, S.; Paul, P. Sunlight Induced Preparation of Functionalized Gold Nanoparticles as Recyclable Colorimetric Dual Sensor for Aluminum and Fluoride in Water. ACS Appl. Mater. Interfaces 2017, 9, 17359–17368. DOI: 10.1021/acsami.7b02742.
- Qiao, J.; Qi, L. Recent Progress in Plant-Gold Nanoparticles Fabrication Methods and Bio-Applications. Talanta 2021, 223, 121396. DOI: 10.1016/j.talanta.2020.121396.
- Nguyen, T. H. A.; Nguyen, V. C.; Phan, T. N. H.; Le, V. T.; Vasseghian, Y.; Trubitsyn, M. A.; Nguyen, A. T.; Chau, T. P.; Doan, V. D. Novel Biogenic Silver and Gold Nanoparticles for Multifunctional Applications: Green Synthesis, Catalytic and Antibacterial Activity, and Colorimetric Detection of Fe(III) Ions. Chemosphere 2022, 287, 132271. DOI: 10.1016/j.chemosphere.2021.132271.
- Badwaik, V. D.; Bartonojo, J. J.; Evans, J. W.; Sahi, S. V.; Willis, C. B.; Dakshinamurthy, R. Single-Step Biofriendly Synthesis of Surface Modifiable, near-Spherical Gold Nanoparticles for Applications in Biological Detection and Catalysis. Langmuir 2011, 27, 5549–5554. DOI: 10.1021/la105041d.
- Gholami-Shabani, M.; Shams-Ghahfarokhi, M.; Gholami-Shabani, Z.; Akbarzadeh, A.; Riazi, G.; Ajdari, S.; Amani, A.; Razzaghi-Abyaneh, M. Enzymatic Synthesis of Gold Nanoparticles Using Sulfite Reductase Purified from Escherichia coli: A Green Eco-Friendly Approach. Process Biochem. 2015, 50, 1076–1085. DOI: 10.1016/j.procbio.2015.04.004.
- Maruyama, T.; Fujimoto, Y.; Maekawa, T. Synthesis of Gold Nanoparticles Using Various Amino Acids. J. Colloid Interface Sci. 2015, 447, 254–257. DOI: 10.1016/j.jcis.2014.12.046.
- Roy, S.; Das, T. K.; Maiti, G. P.; Basu, U. Microbial Biosynthesis of Nontoxic Gold Nanoparticles. Mater. Sci. Eng. B 2016, 203, 41–51. DOI: 10.1016/j.mseb.2015.10.008.
- Ying, S.; Guan, Z.; Ofoegbu, P. C.; Clubb, P.; Rico, C.; He, F.; Hong, J. Green Synthesis of Nanoparticles: Current Developments and Limitations. Environ. Technol. Innov. 2022, 26, 102336. DOI: 10.1016/j.eti.2022.102336.
- Dumur, F.; Dumas, E.; Mayer, C. R. Functionalization of Gold Nanoparticles by Inorganic Entities. Nanomaterials 2020, 10, 548. DOI: 10.3390/nano10030548.
- Nicol, J. R.; Dixon, D.; Coulter, J. A. Gold Nanoparticle Surface Functionalization: A Necessary Requirement in the Development of Novel Nanotherapeutics. Nanomedicine (Lond) 2015, 10, 1315–1326. DOI: 10.2217/nnm.14.219.
- Wang, Z.; Lee, J. H.; Lu, Y. Label-Free Colorimetric Detection of Lead Ions with a Nanomolar Detection Limit and Tunable Dynamic Range by Using Gold Nanoparticles and DNAzyme. Adv. Mater. 2008, 20, 3263–3267. DOI: 10.1002/adma.200703181.
- Fellows, T.; Ho, L.; Flanagan, S.; Fogel, R.; Ojo, D.; Limson, J. Gold Nanoparticle-Streptavidin Conjugates for Rapid and Efficient Screening of Aptamer Function in Lateral Flow Sensors Using Novel CD4-Binding Aptamers Identified through Crossover-SELEX. Analyst 2020, 145, 5180–5193. DOI: 10.1039/d0an00634c.
- Shih, Y. C.; Ke, C. Y.; Yu, C. J.; Lu, C. Y.; Tseng, W. L. Combined Tween 20-Stabilized Gold Nanoparticles and Reduced Graphite Oxide-Fe3O4 Nanoparticle Composites for Rapid and Efficient Removal of Mercury Species from a Complex Matrix. ACS Appl. Mater. Interfaces 2014, 6, 17437–17445. DOI: 10.1021/am5033988.
- Zhao, Y.; Wang, Z.; Zhang, W.; Jiang, X. Adsorbed Tween 80 is Unique in Its Ability to Improve the Stability of Gold Nanoparticles in Solutions of Biomolecules. Nanoscale 2010, 2, 2114–2119. DOI: 10.1039/c0nr00309c.
- Wang, Y.; Quinsaat, J. E. Q.; Ono, T.; Maeki, M.; Tokeshi, M.; Isono, T.; Tajima, K.; Satoh, T.; Sato, S. i.; Miura, Y.; et al. Enhanced Dispersion Stability of Gold Nanoparticles by the Physisorption of Cyclic Poly(Ethylene Glycol). Nat. Commun. 2020, 11, 1–12. DOI: 10.1038/s41467-020-19947-8.
- Chai, F.; Wang, C.; Wang, T.; Li, L.; Su, Z. Colorimetric Detection of Pb2+ Using Glutathione Functionalized Gold Nanoparticles. ACS Appl. Mater. Interfaces 2010, 2, 1466–1470. DOI: 10.1021/am100107k.
- Hermes, J. P.; Sander, F.; Peterle, T.; Urbani, R.; Pfohl, T.; Thompson, D.; Mayor, M. Gold Nanoparticles Stabilized by Thioether Dendrimers. Chemistry 2011, 17, 13473–13481. DOI: 10.1002/chem.201101837.
- Yonezawa, T.; Yasui, K.; Kimizuka, N. Controlled Formation of Smaller Gold Nanoparticles by the Use of Four-Chained Disulfide Stabilizer. Langmuir 2001, 17, 271–273. DOI: 10.1021/la001247c.
- Tzhayik, O.; Sawant, P.; Efrima, S.; Kovalev, E.; Klug, J. T. Xanthate Capping of Silver, Copper, and Gold Colloids. Langmuir 2002, 18, 3364–3369. DOI: 10.1021/la015653n.
- Zhang, X.; Servos, M. R.; Liu, J. Instantaneous and Quantitative Functionalization of Gold Nanoparticles with Thiolated DNA Using a PH-Assisted and Surfactant-Free Route. J. Am. Chem. Soc. 2012, 134, 7266–7269. DOI: 10.1021/ja3014055.
- Luo, C.; Wen, W.; Lin, F.; Zhang, X.; Gu, H.; Wang, S. Simplified Aptamer-Based Colorimetric Method Using Unmodified Gold Nanoparticles for the Detection of Carcinoma Embryonic Antigen. RSC Adv. 2015, 5, 10994–10999. DOI: 10.1039/C4RA14833A.
- Chirra, H. D.; Sexton, T.; Biswal, D.; Hersh, L. B.; Hilt, J. Z. Catalase-Coupled Gold Nanoparticles: Comparison between the Carbodiimide and Biotin–Streptavidin Methods. Acta Biomater. 2011, 7, 2865–2872. DOI: 10.1016/j.actbio.2011.01.003.
- Martínez, Á.; Scrimin, P. Gold Nanoparticles Crosslinking by Peptides and Amino Acids: A Tool for the Colorimetric Identification of Amino Acids. Biopolymers 2018, 109, e23111. DOI: 10.1002/bip.23111.
- Lin, X.; Chen, X. Gold Nanoparticles Stabilized with Four Kinds of Amino Acid-Derived Carbon Dots for Colorimetric and Visual Discrimination of Proteins and Microorganisms. Microchim. Acta 2019, 186, 1–9. DOI: 10.1007/s00604-019-3602-7.
- Zohora, N.; Kumar, D.; Yazdani, M.; Rotello, V. M.; Ramanathan, R.; Bansal, V. Rapid Colorimetric Detection of Mercury Using Biosynthesized Gold Nanoparticles. Colloids Surf. A Physicochem. Eng. Asp 2017, 532, 451–457. DOI: 10.1016/j.colsurfa.2017.04.036.
- Harrison, E.; Coulter, J. A.; Dixon, D. Gold Nanoparticle Surface Functionalization: Mixed Monolayer versus Hetero Bifunctional Peg Linker. Nanomedicine (Lond) 2016, 11, 851–865. DOI: 10.2217/nnm.16.28.
- Poonthiyil, V.; Lindhorst, T. K.; Golovko, V. B.; Fairbanks, A. J. Recent Applications of Click Chemistry for the Functionalization of Gold Nanoparticles and Their Conversion to Glyco-Gold Nanoparticles. Beilstein J. Org. Chem. 2018, 14, 11–24. DOI: 10.3762/bjoc.14.2.
- Mandal, T. K.; Fleming, M. S.; Walt, D. R. Preparation of Polymer Coated Gold Nanoparticles by Surface-Confined Living Radical Polymerization at Ambient Temperature. Nano Lett. 2002, 2, 3–7. DOI: 10.1021/nl015582c.
- Yoon, K. R.; Lee, S. M.; Ramaraj, B.; Kim, D.-P. Surface Initiated-Atom Transfer Radical Polymerization of a Sugar Methacrylate on Gold Nanoparticles. Surf. Interface Anal. 2008, 40, 1139–1143. DOI: 10.1002/sia.2847.
- Kim, Y. H.; Jeon, J.; Hong, S. H.; Rhim, W. K.; Lee, Y. S.; Youn, H.; Chung, J. K.; Lee, M. C.; Lee, D. S.; Kang, K. W.; et al. Tumor Targeting and Imaging Using Cyclic RGD-PEGylated Gold Nanoparticle Probes with Directly Conjugated Iodine-125. Small 2011, 7, 2052–2060. DOI: 10.1002/smll.201100927.
- Mu, C. J.; LaVan, D. A.; Langer, R. S.; Zetter, B. R. Self-Assembled Gold Nanoparticle Molecular Probes for Detecting Proteolytic Activity in Vivo. ACS Nano 2010, 4, 1511–1520. DOI: 10.1021/nn9017334.
- Chiu, T. C.; Huang, C. C. Aptamer-Functionalized Nano-Biosensors. Sensors (Basel) 2009, 9, 10356–10388. DOI: 10.3390/s91210356.
- Ding, B.; Deng, Z.; Yan, H.; Cabrini, S.; Zuckermann, R. N.; Bokor, J. Gold Nanoparticle Self-Similar Chain Structure Organized by DNA Origami. J. Am. Chem. Soc. 2010, 132, 3248–3249. DOI: 10.1021/ja9101198.
- Choi, D. Y.; Kim, S.; Oh, J. W.; Nam, J. M. Conjugation Strategies of DNA to Gold Nanoparticles. Bull. Korean Chem. Soc. 2022. DOI: 10.1002/bkcs.12621.
- Liu, B.; Liu, J. Interface-Driven Hybrid Materials Based on DNA-Functionalized Gold Nanoparticles. Matter 2019, 1, 825–847. DOI: 10.1016/j.matt.2019.08.008.
- Hurst, S. J.; Lytton-Jean, A. K. R.; Mirkin, C. A. Maximizing DNA Loading on a Range of Gold Nanoparticle Sizes. Anal. Chem. 2006, 78, 8313–8318. DOI: 10.1021/ac0613582.
- Mthembu, S. N.; Sharma, A.; Albericio, F.; de la Torre, B. G. Breaking a Couple: Disulfide Reducing Agents. ChemBioChem. 2020, 21, 1947–1954. DOI: 10.1002/cbic.202000092.
- Liu, B.; Liu, J. Methods for Preparing DNA-Functionalized Gold Nanoparticles, a Key Reagent of Bioanalytical Chemistry. Anal. Methods 2017, 9, 2633–2643. DOI: 10.1039/C7AY00368D.
- Moreira-Alvarez, B.; Cid-Barrio, L.; Ferreira, H. S.; Costa-Fernández, J. M.; Encinar, J. R. Integrated Analytical Platforms for the Comprehensive Characterization of Bioconjugated Inorganic Nanomaterials Aiming at Biological Applications. J. Anal. At. Spectrom. 2020, 35, 1518–1529. DOI: 10.1039/D0JA00147C.
- Esfahani, A. R.; Sadiq, Z.; Oyewunmi, O. D.; Safiabadi Tali, S. H.; Usen, N.; Boffito, D. C. C.; Jahanshahi-Anbuhi, S.; Safiabadi-Tali, S. H.; Usen, N.; Boffito, D. C. C.; et al. Portable, Stable, and Sensitive Assay to Detect Phosphate in Water with Gold Nanoparticles (AuNPs) and Dextran Tablet. Analyst 2021, 146, 3697–3708. DOI: 10.1039/d0an02063j.
- Buhr, E.; Senftleben, N.; Klein, T.; Bergmann, D.; Gnieser, D.; Frase, C. G.; Bosse, H. Characterization of Nanoparticles by Scanning Electron Microscopy in Transmission Mode. Meas. Sci. Technol. 2009, 20, 084025. DOI: 10.1088/0957-0233/20/8/084025.
- Liu, J.; Papadakis, R.; Li, H. Experimental Observation of Size-Dependent Behavior in Surface Energy of Gold Nanoparticles through Atomic Force Microscope. Appl. Phys. Lett. 2018, 113, 083108. DOI: 10.1063/1.5046736.
- Ramanaviciene, A.; Voronovic, J.; Popov, A.; Drevinskas, R.; Kausaite-Minkstimiene, A.; Ramanavicius, A. Investigation of Biocatalytic Enlargement of Gold Nanoparticles Using Dynamic Light Scattering and Atomic Force Microscopy. Sens. Actuators B Chem. 2016, 510, 183–189. DOI: 10.1016/j.colsurfa.2016.07.078.
- Zheng, T.; Bott, S.; Huo, Q. Techniques for Accurate Sizing of Gold Nanoparticles Using Dynamic Light Scattering with Particular Application to Chemical and Biological Sensing Based on Aggregate Formation. ACS Appl. Mater. Interfaces 2016, 8, 21585–21594. DOI: 10.1021/acsami.6b06903.
- Nehl, C. L.; Hafner, J. H. Shape-Dependent Plasmon Resonances of Gold Nanoparticles. J. Mater. Chem 2008, 18, 2415. DOI: 10.1039/b714950f.
- Pellegrino, T.; Sperling, R. A.; Alivisatos, A. P.; Parak, W. J. Gel Electrophoresis of Gold-DNA Nanoconjugates. J. Biomed. Biotechnol. 2007, 2007, 26796. DOI: 10.1155/2007/26796.
- Harimech, P. K.; Gerrard, S. R.; El-Sagheer, A. H.; Brown, T.; Kanaras, A. G. Reversible Ligation of Programmed DNA-Gold Nanoparticle Assemblies. J. Am. Chem. Soc. 2015, 137, 9242–9245. DOI: 10.1021/jacs.5b05683.
- Abbasgholi N. Asbaghi, B.; Alsadig, A.; Casalis, L.; Parisse, P.; Niemela, J.; Bellucci, S.; Cabrera, H. An Electrophoresis Approach with Online Thermal Lens Detection to Monitoring DNA Surface Coatings on Gold Nanoparticles. Microchem. J. 2022, 173, 106961. DOI: 10.1016/j.microc.2021.106961.
- Nara, S.; Tripathi, V.; Singh, H.; Shrivastav, T. G. Colloidal Gold Probe Based Rapid Immunochromatographic Strip Assay for Cortisol. Anal. Chim. Acta 2010, 682, 66–71. DOI: 10.1016/j.aca.2010.09.041.
- Akintelu, S. A.; Olugbeko, S. C.; Folorunso, A. S. A Review on Synthesis, Optimization, Characterization and Antibacterial Application of Gold Nanoparticles Synthesized from Plants. Int. Nano Lett. 2020, 10, 237–248. DOI: 10.1007/s40089-020-00317-7.
- Arockiya Aarthi Rajathi, F.; Parthiban, C.; Ganesh Kumar, V.; Anantharaman, P. Biosynthesis of Antibacterial Gold Nanoparticles Using Brown Alga, Stoechospermum Marginatum (Kützing). Spectrochim. Acta A Mol Biomol. Spectrosc. 2012, 99, 166–173. DOI: 10.1016/j.saa.2012.08.081.
- von Bohlen, A.; Brücher, M.; Holland, B.; Wagner, R.; Hergenröder, R. X-Ray Standing Waves and Scanning Electron Microscopy—Energy Dispersive X-Ray Emission Spectroscopy Study of Gold Nanoparticles. Spectrochim. Acta B Spectrosc. 2010, 65, 409–414. DOI: 10.1016/j.sab.2010.04.017.
- Chan, M. Y.; Leng, W.; Vikesland, P. J. Surface-Enhanced Raman Spectroscopy Characterization of Salt-Induced Aggregation of Gold Nanoparticles. ChemPhysChem. 2018, 19, 24–28. DOI: 10.1002/cphc.201700798.
- Mozhayeva, D.; Engelhard, C. A Critical Review of Single Particle Inductively Coupled Plasma Mass Spectrometry-A Step towards an Ideal Method for Nanomaterial Characterization. J. Anal. At. Spectrom. 2020, 35, 1740–1783. DOI: 10.1039/C9JA00206E.
- Pengo, P.; Polizzi, S.; Battagliarin, M.; Pasquato, L.; Scrimin, P. Synthesis, Characterization and Properties of Water-Soluble Gold Nanoparticles with Tunable Core Size. J. Mater. Chem. 2003, 13, 2471–2478. DOI: 10.1039/B306366F.
- Nicolardi, S.; van der Burgt, Y. E. M.; Codée, J. D. C.; Wuhrer, M.; Hokke, C. H.; Chiodo, F. Structural Characterization of Biofunctionalized Gold Nanoparticles by Ultrahigh-Resolution Mass Spectrometry. ACS Nano 2017, 11, 8257–8264. DOI: 10.1021/acsnano.7b03402.
- Link, S.; El-Sayed, M. A. Size and Temperature Dependence of the Plasmon Absorption of Colloidal Gold Nanoparticles. J. Phys. Chem. B 1999, 103, 4212–4217. DOI: 10.1021/jp984796o.
- Njoki, P. N.; Lim, I.-I. S.; Mott, D.; Park, H.-Y.; Khan, B.; Mishra, S.; Sujakumar, R.; Luo, J.; Zhong, C.-J. Size Correlation of Optical and Spectroscopic Properties for Gold Nanoparticles. J. Phys. Chem. C 2007, 111, 14664–14669. DOI: 10.1021/jp074902z.
- Jans, H.; Huo, Q. Gold Nanoparticle-Enabled Biological and Chemical Detection and Analysis. Chem. Soc. Rev. 2012, 41, 2849–2866. DOI: 10.1039/c1cs15280g.
- Zeng, S.; Yong, K. T.; Roy, I.; Dinh, X. Q.; Yu, X.; Luan, F. A Review on Functionalized Gold Nanoparticles for Biosensing Applications. Plasmonics 2011, 6, 491–506. DOI: 10.1007/s11468-011-9228-1.
- Zhao, W.; Brook, M. A.; Li, Y. Design of Gold Nanoparticle-Based Colorimetric Biosensing Assays. ChemBioChem. 2008, 9, 2363–2371. DOI: 10.1002/cbic.200800282.
- Frens, G. Particle Size and Sol Stability in Metal Colloids. Kolloid-Z.u.Z. Polym. 1972, 250, 736–741. DOI: 10.1007/BF01498565.
- Zhang, F.; Wang, S.; Liu, J. Gold Nanoparticles Adsorb DNA and Aptamer Probes Too Strongly and a Comparison with Graphene Oxide for Biosensing. Anal. Chem. 2019, 91, 14743–14750. DOI: 10.1021/acs.analchem.9b04142.
- Zhang, F.; Liu, J. Label‐Free Colorimetric Biosensors Based on Aptamers and Gold Nanoparticles: A Critical Review. Anal. Sens. 2021, 1, 30–43. DOI: 10.1002/anse.202000023.
- Zhou, Y.; Wang, S.; Zhang, K.; Jiang, X. Visual Detection of Copper(II) by Azide- and Alkyne-Functionalized Gold Nanoparticles Using Click Chemistry. Angew. Chem. Int. Ed. Engl. 2008, 47, 7454–7456. DOI: 10.1002/anie.200802317.
- Xie, Y.; Huang, Y.; Li, J.; Wu, J. A Trigger-Based Aggregation of Aptamer-Functionalized Gold Nanoparticles for Colorimetry: An Example on Detection of Escherichia coli O157:H7. Sens. Actuators B Chem. 2021, 339, 129865. DOI: 10.1016/j.snb.2021.129865.
- Pamies, R.; Cifre, J. G. H.; Espín, V. F.; Collado-González, M.; Baños, F. G. D.; De La Torre, J. G. Aggregation Behaviour of Gold Nanoparticles in Saline Aqueous Media. J. Nanoparticle Res. 2014, 16, 1–11. DOI: 10.1007/s11051-014-2376-4.
- Tiwari, N. R.; Rathore, A.; Prabhune, A.; Kulkarni, S. K. Gold Nanoparticles for Colorimetric Detection of Hydrolysis of Antibiotics by Penicillin G Acylase. ABB 2010, 01, 322–329. DOI: 10.4236/abb.2010.14042.
- Zhang, J.; Wang, Y.; Yang, X.; Xu, X.; Yang, X. Specifically Colorimetric Recognition of Calcium, Strontium, and Barium Ions Using 2-Mercaptosuccinic Acid-Functionalized Gold Nanoparticles and Its Use in Reliable Detection of Calcium Ion in Water. Analyst 2011, 136, 3865–3868. DOI: 10.1039/c1an15175d.
- Kim, K.; Nam, Y. S.; Lee, Y.; Lee, K. B. Highly Sensitive Colorimetric Assay for Determining Fe3+ Based on Gold Nanoparticles Conjugated with Glycol Chitosan. J. Anal. Methods Chem. 2017, 2017, 3648564. DOI: 10.1155/2017/3648564.
- Reyes, M.; Piotrowski, M.; Ang, S. K.; Chan, J.; He, S.; Chu, J. J. H.; Kah, J. C. Y. Exploiting the anti-Aggregation of Gold Nanostars for Rapid Detection of Hand, Foot, and Mouth Disease Causing Enterovirus 71 Using Surface-Enhanced Raman Spectroscopy. Anal. Chem. 2017, 89, 5373–5381. DOI: 10.1021/acs.analchem.7b00066.
- Wan, Y.; Zhao, J.; He, J.; Lou, X. Nano-Affi: A Solution-Phase, Label-Free, Colorimetric Aptamer Affinity Assay Based on Binding-Inhibited Aggregation of Gold Nanoparticles. Analyst 2020, 145, 4276–4282. DOI: 10.1039/d0an00827c.
- Hormozi-Nezhad, M. R.; Abbasi-Moayed, S. A Sensitive and Selective Colorimetric Method for Detection of Copper Ions Based on anti-Aggregation of Unmodified Gold Nanoparticles. Talanta 2014, 129, 227–232. DOI: 10.1016/j.talanta.2014.05.022.
- Mao, L.; Wang, Q.; Luo, Y.; Gao, Y. Detection of Ag + Ions via an anti-Aggregation Mechanism Using Unmodified Gold Nanoparticles in the Presence of Thiamazole. Talanta 2021, 222, 121506. DOI: 10.1016/j.talanta.2020.121506.
- Plaisen, S.; Cheewasedtham, W.; Rujiralai, T. Robust Colorimetric Detection Based on the anti-Aggregation of Gold Nanoparticles for Bromide in Rice Samples. RSC Adv. 2018, 8, 21566–21576. DOI: 10.1039/C8RA03497D.
- Song, J.; Huang, P. C.; Wan, Y. Q.; Wu, F. Y. Colorimetric Detection of Thiocyanate Based on anti-Aggregation of Gold Nanoparticles in the Presence of Cetyltrimethyl Ammonium Bromide. Sens. Actuators B Chem. 2016, 222, 790–796. DOI: 10.1016/j.snb.2015.09.006.
- Huang, P. C.; Gao, N.; Li, J. F.; Wu, F. Y. Colorimetric Detection of Methionine Based on anti-Aggregation of Gold Nanoparticles in the Presence of Melamine. Sens. Actuators B Chem. 2018, 255, 2779–2784. DOI: 10.1016/j.snb.2017.09.092.
- Xianyu, Y.; Lin, Y.; Chen, Q.; Belessiotis-Richards, A.; Stevens, M. M.; Thomas, M. R. Iodide-Mediated Rapid and Sensitive Surface Etching of Gold Nanostars for Biosensing. Angew. Chem. Int. Ed. Engl. 2021, 60, 9891–9896. DOI: 10.1002/anie.202017317.
- Pérez-Juste, J.; Pastoriza-Santos, I.; Liz-Marzán, L. M.; Mulvaney, P. Gold Nanorods: Synthesis, Characterization and Applications. Coord. Chem. Rev. 2005, 249, 1870–1901. DOI: 10.1016/j.ccr.2005.01.030.
- Zhang, Z.; Wang, H.; Chen, Z.; Wang, X.; Choo, J.; Chen, L. Plasmonic Colorimetric Sensors Based on Etching and Growth of Noble Metal Nanoparticles: Strategies and Applications. Biosens. Bioelectron. 2018, 114, 52–65. DOI: 10.1016/j.bios.2018.05.015.
- Lone, S. A.; Sadhu, K. K. Time-Dependent Growth of Gold Nanoparticles: Experimental Correlation of Van Der Waals Contact between DNA and Amino Acids with Polar Uncharged Side Chains. J. Phys. Chem. C 2019, 123, 20319–20324. DOI: 10.1021/acs.jpcc.9b04911.
- Compton, O. C.; Osterloh, F. E. Evolution of Size and Shape in the Colloidal Crystallization of Gold Nanoparticles. J Am Chem Soc 2007, 129, 7793–7798. DOI: 10.1021/ja069033q.
- Jayeoye, T. J.; Sirimahachai, U.; Rujiralai, T. Sensitive Colorimetric Detection of Ascorbic Acid Based on Seed Mediated Growth of Sodium Alginate Reduced/Stabilized Gold Nanoparticles. Carbohydr. Polym. 2021, 255, 117376. DOI: 10.1016/j.carbpol.2020.117376.
- Zhao, Y.; Gui, L.; Chen, Z. Colorimetric Detection of Hg2+ Based on Target-Mediated Growth of Gold Nanoparticles. Sens. Actuators B Chem. 2017, 241, 262–267. DOI: 10.1016/j.snb.2016.10.084.
- Liu, B.; Tang, Y.; Yang, Y.; Wu, Y. Design an Aptamer-Based Sensitive Lateral Flow Biosensor for Rapid Determination of Isocarbophos Pesticide in Foods. Food Control 2021, 129, 108208. DOI: 10.1016/j.foodcont.2021.108208.
- Zhao, M.; Yao, X.; Liu, S.; Zhang, H.; Wang, L.; Yin, X.; Su, L.; Xu, B.; Wang, J.; Lan, Q.; et al. Antibiotic and Mammal IgG Based Lateral Flow Assay for Simple and Sensitive Detection of Staphylococcus Aureus. Food Chem. 2021, 339, 127955. DOI: 10.1016/j.foodchem.2020.127955.
- Khlebtsov, B. N.; Tumskiy, R. S.; Burov, A. M.; Pylaev, T. E.; Khlebtsov, N. G. Quantifying the Numbers of Gold Nanoparticles in the Test Zone of Lateral Flow Immunoassay Strips. ACS Appl. Nano Mater. 2019, 2, 5020–5028. DOI: 10.1021/acsanm.9b00956.
- Liu, W.; Tian, L.; Du, J.; Wu, J.; Liu, Y.; Wu, G.; Lu, X. Triggered Peroxidase-like Activity of Au Decorated Carbon Dots for Colorimetric Monitoring of Hg2 + Enrichment in: Chlorella Vulgaris. Analyst 2020, 145, 5500–5507. DOI: 10.1039/d0an00930j.
- Das, R.; Dhiman, A.; Kapil, A.; Bansal, V.; Sharma, T. K. Aptamer-Mediated Colorimetric and Electrochemical Detection of Pseudomonas aeruginosa Utilizing Peroxidase-Mimic Activity of Gold NanoZyme. Anal Bioanal Chem 2019, 411, 1229–1238. DOI: 10.1007/s00216-018-1555-z.
- Franco, J.; Lou; Das, B.; Elliott, C.; Cao, C. Gold Nanozymes : From Concept to Biomedical Applications; Springer Singapore, 2021. DOI: 10.1007/s40820-020-00532-z.
- Xu, Y.; Lu, C.; Sun, Y.; Shao, Y.; Cai, Y.; Zhang, Y.; Miao, J.; Miao, P. A Colorimetric Aptasensor for the Antibiotics Oxytetracycline and Kanamycin Based on the Use of Magnetic Beads and Gold Nanoparticles. Microchim Acta 2018, 185, 1–8. DOI: 10.1007/s00604-018-3077-y.
- Liu, C. P.; Chen, K. C.; Su, C. F.; Yu, P. Y.; Lee, P. W. Revealing the Active Site of Gold Nanoparticles for the Peroxidase-like Activity: The Determination of Surface Accessibility. Catalysts 2019, 9, 517. DOI: 10.3390/catal9060517.
- Liu, Y.; Xiang, Y.; Zhen, Y.; Guo, R. Halide Ion-Induced Switching of Gold Nanozyme Activity Based on Au-X Interactions. Langmuir 2017, 33, 6372–6381. DOI: 10.1021/acs.langmuir.7b00798.
- Zhao, D.; Chen, C.; Lu, L.; Yang, F.; Yang, X. A Label-Free Colorimetric Sensor for Sulfate Based on the Inhibition of Peroxidase-like Activity of Cysteamine-Modified Gold Nanoparticles. Sens. Actuators B Chem. 2015, 215, 437–444. DOI: 10.1016/j.snb.2015.04.010.
- Chen, Z.; Qian, S.; Chen, J.; Cai, J.; Wu, S.; Cai, Z. Protein-Templated Gold Nanoclusters Based Sensor for off-on Detection of Ciprofloxacin with a High Selectivity. Talanta 2012, 94, 240–245. DOI: 10.1016/j.talanta.2012.03.033.
- Briffa, J.; Sinagra, E.; Blundell, R. Heavy Metal Pollution in the Environment and Their Toxicological Effects on Humans. Heliyon 2020, 6, e04691. DOI: 10.1016/j.heliyon.2020.e04691.
- Wang, M.; Chen, Z.; Jing, X.; Zhou, H.; Wang, Y.; Ye, J.; Chu, Q. Tween 20-Capped Gold Nanoparticles for Selective Extraction of Free Low-Molecular-Weight Thiols in Saliva Followed by Capillary Electrophoresis with Contactless Conductivity Detection. J. Chromatogr. B Analyt Technol. Biomed. Life Sci. 2021, 1176, 122756. DOI: 10.1016/j.jchromb.2021.122756.
- Chen, F.; Li, G.; Liu, H.; Leung, C. H.; Ma, D. L. G-Quadruplex-Based Detection of Glyphosate in Complex Biological Systems by a Time-Resolved Luminescent Assay. Sens. Actuators B Chem. 2020, 320, 128393. DOI: 10.1016/j.snb.2020.128393.
- Zhang, J. T.; Kang, T. S.; Wong, S. Y.; Pei, R. J.; Ma, D. L.; Leung, C. H. An Iridium(III) Complex/G-Quadruplex Ensemble for Detection of Ochratoxin a Based on Long-Lifetime Luminescent. Anal. Biochem. 2019, 580, 49–55. DOI: 10.1016/j.ab.2019.06.005.
- Ma, D. L.; Wu, C.; Dong, Z. Z.; Tam, W. S.; Wong, S. W.; Yang, C.; Li, G.; Leung, C. H. The Development of G-Quadruplex-Based Assays for the Detection of Small Molecules and Toxic Substances. Chem. Asian J. 2017, 12, 1851–1860. DOI: 10.1002/asia.201700533.
- Escarpa, A. Lights and Shadows on Food Microfluidics. Lab. Chip 2014, 14, 3213–3224. DOI: 10.1039/c4lc00172a.
- Della Gaspera, E.; Guglielmi, M.; Agnoli, S.; Granozzi, G.; Post, M. L.; Bello, V.; Mattei, G.; Martucci, A. Au Nanoparticles in Nanocrystalline TiO2-NiO Films for SPR-Based, Selective H2S Gas Sensing. Chem. Mater. 2010, 22, 3407–3417. DOI: 10.1021/cm100297q.
- Zhang, Z.; Chen, Z.; Wang, S.; Qu, C.; Chen, L. On-Site Visual Detection of Hydrogen Sulfide in Air Based on Enhancing the Stability of Gold Nanoparticles. ACS Appl. Mater. Interfaces 2014, 6, 6300–6307. DOI: 10.1021/am500564w.
- Martí, A.; Costero, A. M.; Gaviña, P.; Parra, M. Selective Colorimetric NO(g) Detection Based on the Use of Modified Gold Nanoparticles Using Click Chemistry. Chem. Commun. (Camb) 2015, 51, 3077–3079. DOI: 10.1039/c4cc10149a.
- Feng, D.; Zhang, Y.; Shi, W.; Li, X.; Ma, H. A Simple and Sensitive Method for Visual Detection of Phosgene Based on the Aggregation of Gold Nanoparticles. Chem. Commun. (Camb) 2010, 46, 9203–9205. DOI: 10.1039/c0cc02703k.
- Martínez-Aquino, C.; Costero, A. M.; Gil, S.; Gaviña, P. Resorcinol Functionalized Gold Nanoparticles for Formaldehyde Colorimetric Detection. Nanomaterials 2019, 9, 302. DOI: 10.3390/nano9020302.
- Harada, M. Minamata Disease: Methylmercury Poisoning in Japan Caused by Environmental Pollution. Crit. Rev. Toxicol. 1995, 25, 1–24. DOI: 10.3109/10408449509089885.
- Lin, C. Y.; Yu, C. J.; Lin, Y. H.; Tseng, W. L. Colorimetric Sensing of Silver(I) and Mercury(II) Ions Based on an Assembly of Tween 20-Stabilized Gold Nanoparticles. Anal Chem 2010, 82, 6830–6837. DOI: 10.1021/ac1007909.
- Wang, X.; Shen, C.; Zhou, C.; Bu, Y.; Yan, X. Methods, Principles and Applications of Optical Detection of Metal Ios. Chem. Eng. J 2021, 417, 129125. DOI: 10.1016/j.cej.2021.129125.
- Berlina, A. N.; Sotnikov, D. V.; Komova, N. S.; Zherdev, A. V.; Dzantiev, B. B. Limitations for Colorimetric Aggregation Assay of Metal Ions and Ways of Their Overcoming. Anal. Methods 2021, 13, 250–257. DOI: 10.1039/d0ay02068k.
- Hu, S.; Huang, P.-J. J. J.; Wang, J.; Liu, J. Dissecting the Effect of Salt for More Sensitive Label-Free Colorimetric Detection of DNA Using Gold Nanoparticles. Anal. Chem. 2020, 92, 13354–13360. DOI: 10.1021/acs.analchem.0c02688.
- Naderi, M.; Hosseini, M.; Ganjali, M. R. Naked-Eye Detection of Potassium Ions in a Novel Gold Nanoparticle Aggregation-Based Aptasensor. Spectrochim. Acta A Mol. Biomol. Spectrosc. 2018, 195, 75–83. DOI: 10.1016/j.saa.2018.01.051.
- Qiu, J.; Fu, L.; Wang, H.; Zou, R.; Zhang, Y.; Li, X.; Wu, A. Colorimetric Detection of Cs + Based on the Nonmorphological Transition Mechanism of Gold Nanoparticles in the Presence of Prussian Blue. New J. Chem. 2020, 44, 2241–2246. DOI: 10.1039/C9NJ05301H.
- Hu, Y.; Huang, Z.; Liu, B.; Liu, J. Hg(II) Adsorption on Gold Nanoparticles Dominates DNA-Based Label-Free Colorimetric Sensing. ACS Appl. Nano Mater. 2021, 4, 1377–1384. DOI: 10.1021/acsanm.0c02923.
- Bhamore, J. R.; Gul, A. R.; Kailasa, S. K.; Kim, K. W.; Lee, J. S.; Park, H.; Park, T. J. Functionalization of Gold Nanoparticles Using Guanidine Thiocyanate for Sensitive and Selective Visual Detection of Cd2+. Sens. Actuators B Chem. 2021, 334, 129685. DOI: 10.1016/j.snb.2021.129685.
- Duenchay, P.; Chailapakul, O.; Siangproh, W. A Transparency Sheet-Based Colorimetric Device for Simple Determination of Calcium Ions Using Induced Aggregation of Modified Gold Nanoparticles. IJMS 2019, 20, 2954. DOI: 10.3390/ijms20122954.
- Zhang, L.; Huang, D.; Yue, G.; Zhu, J.; Yang, L.; Yang, L.; Dan, W.; Zhao, P. Effective Colorimetric Detection of Ni2+ Using Gold Nanoparticles Functionalized with Phytate. Chem. Phys. Lett. 2021, 784, 139101. DOI: 10.1016/j.cplett.2021.139101.
- Chen, G. H.; Chen, W. Y.; Yen, Y. C.; Wang, C. W.; Chang, H. T.; Chen, C. F. Detection of Mercury(II) Ions Using Colorimetric Gold Nanoparticles on Paper-Based Analytical Devices. Anal. Chem. 2014, 86, 6843–6849. DOI: 10.1021/ac5008688.
- Gan, Y.; Liang, T.; Hu, Q.; Zhong, L.; Wang, X.; Wan, H.; Wang, P. In-Situ Detection of Cadmium with Aptamer Functionalized Gold Nanoparticles Based on Smartphone-Based Colorimetric System. Talanta 2020, 208, 120231. DOI: 10.1016/j.talanta.2019.120231.
- Qi, Y.; Ma, J.; Xiu, F. R.; Gao, X. Determination of Cr(VI) Based on the Peroxidase Mimetic Catalytic Activity of Citrate-Capped Gold Nanoparticles. Microchim Acta 2021, 188, 1–11. DOI: 10.1007/s00604-021-04942-7.
- Mohamed, A.; Li, X.; Li, C.; Li, X.; Yuan, C.; Barakat, H. Smartphone-Based Colorimetric Detection of Chromium (Vi) by Maleic Acid-Functionalized Gold Nanoparticles. Appl. Sci. 2021, 11, 10894. DOI: 10.3390/app112210894.
- Ejeta, S. Y.; Imae, T. Selective Colorimetric and Electrochemical Detections of Cr(III) Pollutant in Water on 3-Mercaptopropionic Acid-Functionalized Gold Plasmon Nanoparticles. Anal. Chim. Acta 2021, 1152, 338272. DOI: 10.1016/j.aca.2021.338272.
- Shellaiah, M.; Thirumalaivasan, N.; Sun, K. W.; Wu, S. P. A PH Cooperative Strategy for Enhanced Colorimetric Sensing of Cr(III) Ions Using Biocompatible L-Glutamic Acid Stabilized Gold Nanoparticles. Microchem. J. 2021, 160, 105754. DOI: 10.1016/j.microc.2020.105754.
- Jia, F.; Liu, Q.; Chen, Z.; Wei, W. Colorimetric Determination of Nine Metal Ions Based on the De-Aggregation of Papain-Functionalized Gold Nanoparticles and Using Three Chelating Agents. Microchim. Acta 2019, 186, 1–7. DOI: 10.1007/s00604-019-4028-y.
- Yu, L.; Pang, Y.; Mo, Z.; Huang, Y.; Shen, X. Coordination Array for Accurate Colorimetric Sensing of Multiple Heavy Metal Ions. Talanta 2021, 231, 122357. DOI: 10.1016/j.talanta.2021.122357.
- Li, X.; Li, S.; Liu, Q.; Chen, Z. Electronic-Tongue Colorimetric-Sensor Array for Discrimination and Quantitation of Metal Ions Based on Gold-Nanoparticle Aggregation. Anal. Chem. 2019, 91, 6315–6320. DOI: 10.1021/acs.analchem.9b01139.
- Sang, F.; Li, X.; Zhang, Z.; Liu, J.; Chen, G. Recyclable Colorimetric Sensor of Cr3+ and Pb2+ Ions Simultaneously Using a Zwitterionic Amino Acid Modified Gold Nanoparticles. Spectrochim. Acta A Mol. Biomol. Spectrosc. 2018, 193, 109–116. DOI: 10.1016/j.saa.2017.11.048.
- Yoon, S. J.; Nam, Y. S.; Lee, Y.; Oh, I. H.; Lee, K. B. A Dual Colorimetric Probe for Rapid Environmental Monitoring of Hg2+ and As3+ Using Gold Nanoparticles Functionalized Withd-Penicillamine. RSC Adv. 2021, 11, 5456–5465. DOI: 10.1039/d0ra08525a.
- Marquez, S.; Morales-Narváez, E. Nanoplasmonics in Paper-Based Analytical Devices. Front. Bioeng. Biotechnol. 2019, 7, 69–10. DOI: 10.3389/fbioe.2019.00069.
- Hyder, A.; Buledi, J. A.; Nawaz, M.; Rajpar, D. B.; Shah, Z.-H.; Orooji, Y.; Yola, M. L.; Karimi-Maleh, H.; Lin, H.; Solangi, A. R. Identification of Heavy Metal Ions from Aqueous Environment through Gold, Silver and Copper Nanoparticles: An Excellent Colorimetric Approach. Environ. Res. 2022, 205, 112475. DOI: 10.1016/j.envres.2021.112475.
- Lou, T.; Chen, L.; Chen, Z.; Wang, Y.; Chen, L.; Li, J. Colorimetric Detection of Trace Copper Ions Based on Catalytic Leaching of Silver-Coated Gold Nanoparticles. ACS Appl. Mater. Interfaces 2011, 3, 4215–4220. DOI: 10.1021/am2008486.
- Liu, R.; Chen, Z.; Wang, S.; Qu, C.; Chen, L.; Wang, Z. Colorimetric Sensing of Copper(II) Based on Catalytic Etching of Gold Nanoparticles. Talanta 2013, 112, 37–42. DOI: 10.1016/j.talanta.2013.01.065.
- Liu, Y.; Ding, D.; Zhen, Y.; Guo, R. Amino Acid-Mediated ‘Turn-off/Turn-On’ Nanozyme Activity of Gold Nanoclusters for Sensitive and Selective Detection of Copper Ions and Histidine. Biosens. Bioelectron. 2017, 92, 140–146. DOI: 10.1016/j.bios.2017.01.036.
- Xie, Y. F.; Cheng, Y. Y.; Liu, M. L.; Zou, H. Y.; Huang, C. Z. A Single Gold Nanoprobe for Colorimetric Detection of Silver(I) Ions with Dark-Field Microscopy. Analyst 2019, 144, 2011–2016. DOI: 10.1039/c8an02397b.
- Jiang, X.; Xu, W.; Chen, X.; Liang, Y. Colorimetric Assay for Ultrasensitive Detection of Ag(I) Ions Based on the Formation of Gold Nanoparticle Oligomers. Anal Bioanal Chem 2019, 411, 2439–2445. DOI: 10.1007/s00216-019-01685-6.
- Safavi, A.; Ahmadi, R.; Mohammadpour, Z. Colorimetric Sensing of Silver Ion Based on anti Aggregation of Gold Nanoparticles. Sens. Actuators B Chem. 2017, 242, 609–615. DOI: 10.1016/j.snb.2016.11.043.
- Li, X.; Wu, Z.; Zhou, X.; Hu, J. Colorimetric Response of Peptide Modified Gold Nanoparticles: An Original Assay for Ultrasensitive Silver Detection. Biosens. Bioelectron. 2017, 92, 496–501. DOI: 10.1016/j.bios.2016.10.075.
- Zhao, L.; Wang, Y.; Li, Z.; Deng, Y.; Zhao, X.; Xia, Y. Facile Synthesis of Chitosan-Gold Nanocomposite and Its Application for Exclusively Sensitive Detection of Ag + Ions. Carbohydr. Polym. 2019, 226, 115290. DOI: 10.1016/j.carbpol.2019.115290.
- Mahajan, P. G.; Dige, N. C.; Vanjare, B. D.; Hong, S. K.; Lee, K. H.; Hwan, K. Gallotannin Functionalized Gold Nanoparticles for Rapid, Selective and Sensitive Colorimetric Detection of Ag+ in Aqueous Medium: Approach to Eco-Friendly Water Analysis. Sens. Actuators B Chem. 2019, 281, 720–729. DOI: 10.1016/j.snb.2018.10.116.
- Zuber, A.; Purdey, M.; Schartner, E.; Forbes, C.; Van Der Hoek, B.; Giles, D.; Abell, A.; Monro, T.; Ebendorff-Heidepriem, H. Detection of Gold Nanoparticles with Different Sizes Using Absorption and Fluorescence Based Method. Sens. Actuators B Chem. 2016, 227, 117–127. DOI: 10.1016/j.snb.2015.12.044.
- Bagheri, N.; Mazzaracchio, V.; Cinti, S.; Colozza, N.; Di Natale, C.; Netti, P. A.; Saraji, M.; Roggero, S.; Moscone, D.; Arduini, F. Electroanalytical Sensor Based on Gold-Nanoparticle-Decorated Paper for Sensitive Detection of Copper Ions in Sweat and Serum. Anal. Chem. 2021, 93, 5225–5233. DOI: 10.1021/acs.analchem.0c05469.
- Priyadarshini, E.; Pradhan, N.; Panda, P. K.; Mishra, B. K. Biogenic Unmodified Gold Nanoparticles for Selective and Quantitative Detection of Cerium Using UV-Vis Spectroscopy and Photon Correlation Spectroscopy (DLS). Biosens. Bioelectron. 2015, 68, 598–603. DOI: 10.1016/j.bios.2015.01.048.
- Liu, X.; Ouyang, W.; Shu, Y.; Tian, Y.; Feng, Y.; Zhang, T.; Chen, W. Incorporating Bioaccessibility into Health Risk Assessment of Heavy Metals in Particulate Matter Originated from Different Sources of Atmospheric Pollution. Environ. Pollut. 2019, 254, 113113. DOI: 10.1016/j.envpol.2019.113113.
- Rogosnitzky, M.; Branch, S. Gadolinium-Based Contrast Agent Toxicity: A Review of Known and Proposed Mechanisms. Biometals 2016, 29, 365–376. DOI: 10.1007/s10534-016-9931-7.
- Park, K.; Park, J.; Lee, H.; Choi, J.; Yu, W. J.; Lee, J. Toxicity and Tissue Distribution of Cerium Oxide Nanoparticles in Rats by Two Different Routes: Single Intravenous Injection and Single Oral Administration. Arch. Pharm. Res. 2018, 41, 1108–1116. DOI: 10.1007/s12272-018-1074-7.
- He, B.; Wang, J.; Lin, J.; Chen, J.; Zhuang, Z.; Hong, Y.; Yan, L.; Lin, L.; Shi, B.; Qiu, Y.; et al. Association between Rare Earth Element Cerium and the Risk of Oral Cancer: A Case-Control Study in Southeast China. Front. Public Heal 2021, 9, 647120. DOI: 10.3389/fpubh.2021.647120.
- Ma, Y.; Li, P.; Zhao, L.; Liu, J.; Yu, J.; Huang, Y.; Zhu, Y.; Li, Z.; Zhao, R.; Hua, S.; et al. Size-Dependent Cytotoxicity and Reactive Oxygen Species of Cerium Oxide Nanoparticles in Human Retinal Pigment Epithelia Cells. Int. J. Nanomedicine 2021, 16, 5333–5341. DOI: 10.2147/IJN.S305676.
- Lisowski, C. E.; Hutchison, J. E. Malonamide-Functionalized Gold Nanoparticles for Selective, Colorimetric Sensing of Trivalent Lanthanide Ions. Anal. Chem. 2009, 81, 10246–10253. DOI: 10.1021/ac902271t.
- Pallares, R. M.; Carter, K. P.; Zeltmann, S. E.; Tratnjek, T.; Minor, A. M.; Abergel, R. J. Selective Lanthanide Sensing with Gold Nanoparticles and Hydroxypyridinone Chelators. Inorg. Chem. 2020, 59, 2030–2036. DOI: 10.1021/acs.inorgchem.9b03393.
- Mondal, P.; Yarger, J. L. Colorimetric Dual Sensors of Metal Ions Based on 1,2,3-Triazole-4,5-Dicarboxylic Acid-Functionalized Gold Nanoparticles. J. Phys. Chem. C 2019, 123, 20459–20467. DOI: 10.1021/acs.jpcc.9b03721.
- Ducatman, A.; Luster, M.; Fletcher, T. Perfluoroalkyl Substance Excretion: Effects of Organic Anion-Inhibiting and Resin-Binding Drugs in a Community Setting. Environ. Toxicol. Pharmacol. 2021, 85, 103650. DOI: 10.1016/j.etap.2021.103650.
- Beer, P. D.; Gale, P. A. Anion Recognition and Sensing : The State of the Art and Future Perspectives. Angew. Chem. Int. Ed. 2001, 40, 486–516. DOI: 10.1002/1521-3773(20010202)40:3<486::AID-ANIE486>3.0.CO;2-P.
- Sepahvand, M.; Ghasemi, F.; Seyed Hosseini, H. M. Plasmonic Nanoparticles for Colorimetric Detection of Nitrite and Nitrate. Food Chem. Toxicol. 2021, 149, 112025. DOI: 10.1016/j.fct.2021.112025.
- Mamatioğlu, F.; Üzer, A.; Erçağ, E.; Apak, R. Development of a Gold Nanoparticles-Based Colorimetric Sensor for the Indirect Determination of Ammonium Dinitramide and Tetryl. Talanta 2021, 226, 122187. DOI: 10.1016/j.talanta.2021.122187.
- He, Z.; Zhang, L.; Peng, G.; Wang, G.; Liang, X. Chemical Redox-Modulated Etching of Plasmonic Nanoparticles for Nitrite Detection: Comparison among Gold Nanosphere, Nanorod, and Nanotriangle. J. Anal. Test 2021, 5, 350–359. DOI: 10.1007/s41664-021-00153-4.
- Adegoke, O.; Zolotovskaya, S.; Abdolvand, A.; Nic, N. Rapid and Highly Selective Colorimetric Detection of Nitrite Based on the Catalytic-Enhanced Reaction of Mimetic Au Nanoparticle-CeO2 Nanoparticle-Graphene Oxide Hybrid Nanozyme. Talanta 2021, 224, 121875. DOI: 10.1016/j.talanta.2020.121875.
- Liu, W.; Du, Z.; Qian, Y.; Li, F. A Specific Colorimetric Probe for Phosphate Detection Based on anti-Aggregation of Gold Nanoparticles. Sens. Actuators B Chem. 2013, 176, 927–931. DOI: 10.1016/j.snb.2012.10.074.
- Wu, L.; Qiu, X.; Wang, T.; Tao, K.; Bao, L.; Zeng, E. Y. Water Quality and Organic Pollution with Health Risk Assessment in China: A Short Review. ACS Est. Water 2022, 2, 1279–1288. DOI: 10.1021/acsestwater.2c00137.
- Mishra, A.; Kumari, M.; Kumar, R.; Iqbal, K.; Thakur.; I. S.; Swati. Bioresource Technology Reports Persistent Organic Pollutants in the Environment : Risk Assessment, Hazards, and Mitigation Strategies. Bioresour. Technol. Rep. 2022, 19, 101143. DOI: 10.1016/j.biteb.2022.101143.
- Fan, X.; Wang, Z.; Li, Y.; Wang, H.; Fan, W.; Dong, Z. Estimating the Dietary Exposure and Risk of Persistent Organic Pollutants in China: A National Analysis. Environ. Pollut. 2021, 288, 117764. DOI: 10.1016/j.envpol.2021.117764.
- Stratakis, N.; Rock, S.; La Merrill, M. A.; Saez, M.; Robinson, O.; Fecht, D.; Vrijheid, M.; Valvi, D.; Conti, D. V.; McConnell, R. Prenatal Exposure to Persistent Organic Pollutants and Childhood Obesity: A Systematic Review and Meta-Analysis of Human Studies. Obes. Rev. 2022, 23, 1–16. DOI: 10.1111/obr.13383.
- Okoro, H. K.; Pandey, S.; Ogunkunle, C. O.; Ngila, C. J.; Zvinowanda, C.; Jimoh, I.; Lawal, I. A.; Orosun, M. M.; Adeniyi, A. G. Nanomaterial-Based Biosorbents: Adsorbent for Efficient Removal of Selected Organic Pollutants from Industrial Wastewater. Emerg. Contam. 2022, 8, 46–58. DOI: 10.1016/j.emcon.2021.12.005.
- Wagner, M.; Andrew Lin, K. Y.; Oh, W.; Da; Lisak, G. Metal-Organic Frameworks for Pesticidal Persistent Organic Pollutants Detection and Adsorption – A Mini Review. J. Hazard Mater. 2021, 413, 125325. DOI: 10.1016/j.jhazmat.2021.125325.
- Tseng, W. B.; Hsieh, M. M.; Chen, C. H.; Chiu, T. C.; Tseng, W. L. Functionalized Gold Nanoparticles for Sensing of Pesticides: A Review. J. Food Drug Anal. 2020, 28, 521–538. DOI: 10.38212/2224-6614.1092.
- Liu, D. M.; Xu, B.; Dong, C. Recent Advances in Colorimetric Strategies for Acetylcholinesterase Assay and Their Applications. TrAC – Trends Anal. Chem. 2021, 142, 116320. DOI: 10.1016/j.trac.2021.116320.
- Ozcelikay, G.; Cetinkaya, A.; Kaya, S. I.; Yence, M.; Canavar Eroğlu, P. E.; Unal, M. A.; Ozkan, S. A. Novel Sensor Approaches of Aflatoxins Determination in Food and Beverage Samples. Crit. Rev. Anal. Chem. 2022, 1–20. DOI: 10.1080/10408347.2022.2105136.
- Erarpat, S.; Bodur, S.; Bakırdere, S. Nanoparticles Based Extraction Strategies for Accurate and Sensitive Determination of Different Pesticides. Crit. Rev. Anal. Chem. 2022, 52, 1370–1385. DOI: 10.1080/10408347.2021.1876552.
- da Costa Filho, B. M.; Duarte, A. C.; Santos, T. A. P. R. Environmental Monitoring Approaches for the Detection of Organic Contaminants in Marine Environments: A Critical Review. Trends Environ. Anal. Chem. 2022, 33, e00154. DOI: 10.1016/j.teac.2022.e00154.
- Zhou, Y.; Li, C.; Liu, R.; Chen, Z.; Li, L.; Li, W.; He, Y.; Yuan, L. Label-Free Colorimetric Detection of Prothioconazole Using Gold Nanoparticles Based on One-Step Reaction. ACS Biomater. Sci. Eng. 2020, 6, 2805–2811. DOI: 10.1021/acsbiomaterials.0c00208.
- Rana, K.; Bhamore, J. R.; Rohit, J. V.; Park, T. J.; Kailasa, S. K. Ligand Exchange Reactions on Citrate-Gold Nanoparticles for a Parallel Colorimetric Assay of Six Pesticides. New J. Chem. 2018, 42, 9080–9090. DOI: 10.1039/C8NJ01294F.
- Kailasa, S. K.; Nguyen, T. P.; Baek, S. H.; Tu Phan, L. M.; Rafique, R.; Park, T. J. Assembly of 6-Aza-2-Thiothymine on Gold Nanoparticles for Selective and Sensitive Colorimetric Detection of Pencycuron in Water and Food Samples. Talanta 2019, 205, 120087. DOI: 10.1016/j.talanta.2019.06.087.
- Wang, S.; Su, L.; Wang, L.; Zhang, D.; Shen, G.; Ma, Y. Colorimetric Determination of Carbendazim Based on the Specific Recognition of Aptamer and the Poly-Diallyldimethylammonium Chloride Aggregation of Gold Nanoparticles. Spectrochim. Acta A Mol. Biomol. Spectrosc. 2020, 228, 117809. DOI: 10.1016/j.saa.2019.117809.
- Chen, H.; Shi, Q.; Fu, H.; Hu, O.; Fan, Y.; Xu, L.; Zhang, L.; Lan, W.; Sun, D.; Yang, T.; et al. Rapid Detection of Five Pesticide Residues Using Complexes of Gold Nanoparticle and Porphyrin Combined with Ultraviolet Visible Spectrum. J. Sci. Food Agric. 2020, 100, 4464–4473. DOI: 10.1002/jsfa.10487.
- Kuitio, C.; Klangprapan, S.; Chingkitti, N.; Boonthavivudhi, S.; Choowongkomon, K. Aptasensor for Paraquat Detection by Gold Nanoparticle Colorimetric Method. J. Environ. Sci. Health B 2021, 56, 370–377. DOI: 10.1080/03601234.2021.1888615.
- Zhang, Y.; Huang, Y.; Fu, L.; Qiu, J.; Wang, Z.; Wu, A. Colorimetric Detection of Paraquat in Aqueous and Fruit Juice Samples Based on Functionalized Gold Nanoparticles. J. Food Compos. Anal. 2020, 92, 103574. DOI: 10.1016/j.jfca.2020.103574.
- Wang, S.; Ye, Z.; Wang, X.; Xiao, L. Etching of Single-MnO2-Coated Gold Nanoparticles for the Colorimetric Detection of Organophosphorus Pesticides. ACS Appl. Nano Mater. 2019, 2, 6646–6654. DOI: 10.1021/acsanm.9b01517.
- Shah, M. M.; Ren, W.; Irudayaraj, J.; Sajini, A. A.; Ali, M. I.; Ahmad, B. Colorimetric Detection of Organophosphate Pesticides Based on Acetylcholinesterase and Cysteamine Capped Gold Nanoparticles as Nanozyme. Sensors 2021, 21, 8050. DOI: 10.3390/s21238050.
- Hu, Y.; Wang, J.; Wu, Y. A Simple and Rapid Chemosensor for Colorimetric Detection of Dimethoate Pesticide Based on the Peroxidase-Mimicking Catalytic Activity of Gold Nanoparticles. Anal. Methods 2019, 11, 5337–5347. DOI: 10.1039/C9AY01506J.
- Han, H.; Liu, J.; Zhou, J.; Li, Y.; Wang, W.; Lu, Z. Kanamycin Adsorption on Gold Nanoparticles Dominates Its Label-Free Colorimetric Sensing with Its Aptamer. Langmuir 2020, 36, 11490–11498. DOI: 10.1021/acs.langmuir.0c01786.
- Chang, C. C.; Wang, G.; Takarada, T.; Maeda, M. Target-Recycling-Amplified Colorimetric Detection of Pollen Allergen Using Non-Cross-Linking Aggregation of Dna-Modified Gold Nanoparticles. ACS Sens. 2019, 4, 363–369. DOI: 10.1021/acssensors.8b01156.
- He, Y.; Tian, F.; Zhou, J.; Zhao, Q.; Fu, R.; Jiao, B. Colorimetric Aptasensor for Ochratoxin a Detection Based on Enzyme-Induced Gold Nanoparticle Aggregation. J Hazard Mater. 2020, 388, 121758. DOI: 10.1016/j.jhazmat.2019.121758.
- Tian, F.; Zhou, J.; Fu, R.; Cui, Y.; Zhao, Q.; Jiao, B.; He, Y. Multicolor Colorimetric Detection of Ochratoxin a via Structure-Switching Aptamer and Enzyme-Induced Metallization of Gold Nanorods. Food Chem. 2020, 320, 126607. DOI: 10.1016/j.foodchem.2020.126607.
- Kailasa, S. K.; Koduru, J. R.; Desai, M. L.; Park, T. J.; Singhal, R. K.; Basu, H. Recent Progress on Surface Chemistry of Plasmonic Metal Nanoparticles for Colorimetric Assay of Drugs in Pharmaceutical and Biological Samples. TrAC – Trends Anal. Chem. 2018, 105, 106–120. DOI: 10.1016/j.trac.2018.05.004.
- Zhou, X.; Wang, L.; Shen, G.; Zhang, D.; Xie, J.; Mamut, A.; Huang, W.; Zhou, S. Colorimetric Determination of Ofloxacin Using Unmodified Aptamers and the Aggregation of Gold Nanoparticles. Microchim. Acta 2018, 185, 1–9. DOI: 10.1007/s00604-018-2895-2.
- Zhang, Z.; Tian, Y.; Huang, P.; Wu, F. Y. Using Target-Specific Aptamers to Enhance the Peroxidase-like Activity of Gold Nanoclusters for Colorimetric Detection of Tetracycline Antibiotics. Talanta 2020, 208, 120342. DOI: 10.1016/j.talanta.2019.120342.
- Raja, D. A.; Musharraf, S. G.; Shah, M. R.; Jabbar, A.; Bhanger, M. I.; Malik, M. I. Poly(Propylene Glycol) Stabilized Gold Nanoparticles: An Efficient Colorimetric Assay for Ceftriaxone. J. Ind. Eng. Chem. 2020, 87, 180–186. DOI: 10.1016/j.jiec.2020.03.041.
- Khurana, S.; Kukreti, S.; Kaushik, M. Designing a Two-Stage Colorimetric Sensing Strategy Based on Citrate Reduced Gold Nanoparticles: Sequential Detection of Sanguinarine (Anticancer Drug) and Visual Sensing of DNA. Spectrochim. Acta A Mol. Biomol. Spectrosc. 2021, 246, 119039. DOI: 10.1016/j.saa.2020.119039.
- Wu, Y. Y.; Huang, P.; Wu, F. Y. A Label-Free Colorimetric Aptasensor Based on Controllable Aggregation of AuNPs for the Detection of Multiplex Antibiotics. Food Chem. 2020, 304, 125377. DOI: 10.1016/j.foodchem.2019.125377.
- Ma, Q.; Wang, Y.; Jia, J.; Xiang, Y. Colorimetric Aptasensors for Determination of Tobramycin in Milk and Chicken Eggs Based on DNA and Gold Nanoparticles. Food Chem. 2018, 249, 98–103. DOI: 10.1016/j.foodchem.2018.01.022.
- Huang, W.; Wang, Y.; Wang, L.; Pan, C.; Shen, G. Colorimetric Detection of Ciprofloxacin in Aqueous Solution Based on an Unmodified Aptamer and the Aggregation of Gold Nanoparticles. Anal. Methods 2021, 13, 90–98. DOI: 10.1039/d0ay01811b.
- Birader, K.; Kumar, P.; Tammineni, Y.; Barla, J. A.; Reddy, S.; Suman, P. Colorimetric Aptasensor for on-Site Detection of Oxytetracycline Antibiotic in Milk. Food Chem. 2021, 356, 129659. DOI: 10.1016/j.foodchem.2021.129659.
- Ramalingam, S.; Collier, C. M.; Singh, A. A Paper-Based Colorimetric Aptasensor for the Detection of Gentamicin. Biosensors 2021, 11, 29. DOI: 10.3390/bios11020029.
- Pourreza, N.; Ghomi, M. A Network Composed of Gold Nanoparticles and a Poly(Vinyl Alcohol) Hydrogel for Colorimetric Determination of Ceftriaxone. Microchim. Acta 2020, 187, 1–10. DOI: 10.1007/s00604-019-4039-8.
- Raja, D. A.; Munir, F.; Shah, M. R.; Bhanger, M. I.; Malik, M. I. Colorimetric Sensing of Cephradine through Polypropylene Glycol Functionalized Gold Nanoparticles. R Soc Open Sci 2021, 8, 210185. DOI: 10.1098/rsos.210185.
- Turcanu, V.; Brough, H. A.; Du Toit, G.; Foong, R. X.; Marrs, T.; Santos, A. F.; Lack, G. Immune Mechanisms of Food Allergy and Its Prevention by Early Intervention. Curr. Opin. Immunol. 2017, 48, 92–98. DOI: 10.1016/j.coi.2017.08.009.
- Ramesh, T.; Hong, P.; Maqsood, L.; Soomro, A.; Zheng, H.; Li, X.; Wang, K. Visual Detection of Tropomyosin, a Major Shrimp Allergenic Protein Using Gold Nanoparticles (AuNPs) - Assisted Colorimetric Aptasensor. Mar. Life Sci. Technol. 2021, 3, 382–394. DOI: 10.1007/s42995-020-00085-5.
- Xing, K.; Peng, J.; Chen, W.; Fang, B.; Liu, D.; Shan, S.; Zhang, G.; Huang, Y.; Lai, W. Development of a Label-Free Plasmonic Gold Nanoparticles Aggregates Sensor on the Basis of Charge Neutralization for the Detection of Zearalenone. Food Chem. 2022, 370, 131365. DOI: 10.1016/j.foodchem.2021.131365.
- Kim, E.; Hahn, J.; Ban, C.; Jo, Y.; Han, H.; Lim, S.; Choi, Y. J. Visible on-Site Detection of Ara h 1 by the Switchable-Linker-Mediated Precipitation of Gold Nanoparticles. Food Chem. 2021, 352, 129354. DOI: 10.1016/j.foodchem.2021.129354.
- Yuan, D.; Fang, X.; Liu, Y.; Kong, J.; Chen, Q. A Hybridization Chain Reaction Coupled with Gold Nanoparticles for Allergen Gene Detection in Peanut, Soybean and Sesame DNAs. Analyst 2019, 144, 3886–3891. DOI: 10.1039/c9an00394k.
- Lee, J. I.; Jang, S. C.; Chung, J.; Choi, W. K.; Hong, C.; Ahn, G. R.; Kim, S. H.; Lee, B. Y.; Chung, W. J. Colorimetric Allergenic Fungal Spore Detection Using Peptide-Modified Gold Nanoparticles. Sens. Actuators B Chem. 2021, 327, 128894. DOI: 10.1016/j.snb.2020.128894.
- Singh, J.; Mehta, A. Rapid and Sensitive Detection of Mycotoxins by Advanced and Emerging Analytical Methods: A Review. Food Sci. Nutr. 2020, 8, 2183–2204. DOI: 10.1002/fsn3.1474.
- Huang, X.; Huang, T.; Li, X.; Huang, Z. Flower-like Gold Nanoparticles-Based Immunochromatographic Test Strip for Rapid Simultaneous Detection of Fumonisin B1 and Deoxynivalenol in Chinese Traditional Medicine. J. Pharm. Biomed. Anal. 2020, 177, 112895. DOI: 10.1016/j.jpba.2019.112895.
- Bu, T.; Zhang, M.; Sun, X.; Tian, Y.; Bai, F.; Jia, P.; Bai, Y.; Zhe, T.; Wang, L. Gold Nanoparticles-Functionalized Microorganisms Assisted Construction of Immunobiosensor for Sensitive Detection of Ochratoxin a in Food Samples. Sens. Actuators B Chem. 2019, 299, 126969. DOI: 10.1016/j.snb.2019.126969.
- Wu, Y.; Zhou, Y.; Huang, H.; Chen, X.; Leng, Y.; Lai, W.; Huang, X.; Xiong, Y. Engineered Gold Nanoparticles as Multicolor Labels for Simultaneous Multi-Mycotoxin Detection on the Immunochromatographic Test Strip Nanosensor. Sens. Actuators B Chem. 2020, 316, 128107. DOI: 10.1016/j.snb.2020.128107.
- Yu, Y.; Li, Y.; Zhang, Q.; Zha, Y.; Lu, S.; Yang, Y.; Li, P.; Zhou, Y. Colorimetric Immunoassay via Smartphone Based on Mn2+-Mediated Aggregation of AuNPs for Convenient Detection of Fumonisin B1. Food Control 2022, 132, 108481. DOI: 10.1016/j.foodcont.2021.108481.
- Ren, W.; Huang, Z.; Xu, Y.; Li, Y.; Ji, Y.; Su, B. Urchin-like Gold Nanoparticle-Based Immunochromatographic Strip Test for Rapid Detection of Fumonisin B1 in Grains. Anal. Bioanal. Chem. 2015, 407, 7341–7348. DOI: 10.1007/s00216-015-8896-7.
- Zhang, W.; Wang, Y. Y.; Nan, M.; Li, Y.; Yun, J.; Wang, Y. Y.; Bi, Y. Novel Colorimetric Aptasensor Based on Unmodified Gold Nanoparticle and SsDNA for Rapid and Sensitive Detection of T-2 Toxin. Food Chem. 2021, 348, 129128. DOI: 10.1016/j.foodchem.2021.129128.
- Miron-Merida, V. A.; Gonzalez-Espinosa, Y.; Collado-Gonzalez, M.; Gong, Y. Y.; Guo, Y.; Goycoolea, F. M. Aptamer–Target–Gold Nanoparticle Conjugates for the Quantification of Fumonisin B1. Biosensors 2021, 11, 18. DOI: 10.3390/bios11010018.
- Lerdsri, J.; Soongsong, J.; Laolue, P.; Jakmunee, J. Reliable Colorimetric Aptasensor Exploiting 72-Mers SsDNA and Gold Nanoprobes for Highly Sensitive Detection of Aflatoxin M1 in Milk. J. Food Compos. Anal. 2021, 102, 103992. DOI: 10.1016/j.jfca.2021.103992.
- Lerdsri, J.; Chananchana, W.; Upan, J.; Sridara, T.; Jakmunee, J. Label-Free Colorimetric Aptasensor for Rapid Detection of Aflatoxin B1 by Utilizing Cationic Perylene Probe and Localized Surface Plasmon Resonance of Gold Nanoparticles. Sens. Actuators B Chem. 2020, 320, 128356. DOI: 10.1016/j.snb.2020.128356.
- Qian, J.; Ren, C.; Wang, C.; An, K.; Cui, H.; Hao, N.; Wang, K. Gold Nanoparticles Mediated Designing of Versatile Aptasensor for Colorimetric/Electrochemical Dual-Channel Detection of Aflatoxin B1. Biosens. Bioelectron 2020, 166, 112443. DOI: 10.1016/j.bios.2020.112443.
- Zhu, W.; Li, L.; Zhou, Z.; Yang, X.; Hao, N.; Guo, Y.; Wang, K. A Colorimetric Biosensor for Simultaneous Ochratoxin a and Aflatoxins B1 Detection in Agricultural Products. Food Chem. 2020, 319, 126544. DOI: 10.1016/j.foodchem.2020.126544.
- Sun, S.; Xie, Y. An Enhanced Enzyme-Linked Aptamer Assay for the Detection of Zearalenone Based on Gold Nanoparticles. Anal. Methods 2021, 13, 1255–1260. DOI: 10.1039/d0ay02173c.
- Sheini, A. Colorimetric Aggregation Assay Based on Array of Gold and Silver Nanoparticles for Simultaneous Analysis of Aflatoxins, Ochratoxin and Zearalenone by Using Chemometric Analysis and Paper Based Analytical Devices. Microchim. Acta 2020, 187, 1–11. DOI: 10.1007/s00604-020-4147-5.
- Cerra, S.; Salamone, T. A.; Sciubba, F.; Marsotto, M.; Battocchio, C.; Nappini, S.; Scaramuzzo, F. A.; Li Voti, R.; Sibilia, C.; Matassa, R.; et al. Study of the Interaction Mechanism between Hydrophilic Thiol Capped Gold Nanoparticles and Melamine in Aqueous Medium. Colloids Surf. B Biointerfaces 2021, 203, 111727. DOI: 10.1016/j.colsurfb.2021.111727.
- Jia, M.; Sha, J.; Li, Z.; Wang, W.; Zhang, H. High Affinity Truncated Aptamers for Ultra-Sensitive Colorimetric Detection of Bisphenol a with Label-Free Aptasensor. Food Chem. 2020, 317, 126459. DOI: 10.1016/j.foodchem.2020.126459.
- Yang, J.; Sun, Q.; Huang, C.; Qin, S.; Han, S.; Huo, Z.; Li, Y.; Sun, X.; Chen, J. 3-Aminophenylboronic Acid-Mediated Aggregation of Gold Nanoparticles for Colorimetric Sensing of Iohexol in Environmental and Biological Samples. Spectrochim Acta A Mol Biomol Spectrosc 2021, 261, 120004. DOI: 10.1016/j.saa.2021.120004.
- Takahashi, F.; Kazui, Y.; Miyaguchi, H.; Ohmori, T.; Tanaka, R.; Jin, J. Simple Colorimetric Screening of the Nerve Agent VX Using Gold Nanoparticles and a Hand-Powered Extraction Device. Sens. Actuators B Chem. 2021, 327, 128902. DOI: 10.1016/j.snb.2020.128902.
- Liu, X.; He, F.; Zhang, F.; Zhang, Z.; Huang, Z.; Liu, J. Dopamine and Melamine Binding to Gold Nanoparticles Dominates Their Aptamer-Based Label-Free Colorimetric Sensing. Anal. Chem. 2020, 92, 9370–9378. DOI: 10.1021/acs.analchem.0c01773.
- Brasiunas, B.; Popov, A.; Ramanavicius, A.; Ramanaviciene, A. Gold Nanoparticle Based Colorimetric Sensing Strategy for the Determination of Reducing Sugars. Food Chem. 2021, 351, 129238. DOI: 10.1016/j.foodchem.2021.129238.
- Liang, W.; Wei, Y.; Gao, M.; Yan, X.; Zhu, X.; Guo, W. Detection of Melamine Adulteration in Milk Powder by Using Optical Spectroscopy Technologies in the Last Decade—a Review. Food Anal. Methods 2020, 13, 2059–2069. DOI: 10.1007/s12161-020-01822-3.
- Harahap, R. M.; Lutfi Firdaus, M. Nanomaterial-Based Colorimetric Sensors for Melamine and Polymers Analysis: A Review. Proc. Int. Conf. Sci. 2021, 2, 1–9.
- Siddiquee, S.; Saallah, S.; Bohari, N. A.; Ringgit, G.; Roslan, J.; Naher, L.; Nudin, N. F. H. Visual and Optical Absorbance Detection of Melamine in Milk by Melamine-Induced Aggregation of Gold Nanoparticles. Nanomaterials 2021, 11, 1142–1111. DOI: 10.3390/nano11051142.
- Xie, T.; Ma, J.; Zhou, M.; Fu, Y.; Jiao, T. Comparison of Visual Detection of Melamine by AuNPs Sol Prepared in Marine and Terrestrial Plant Extracts. Colloids Surf. A Physicochem. Eng. Asp 2021, 614, 126133. DOI: 10.1016/j.colsurfa.2021.126133.
- Elliott, M. Biological Pollutants and Biological Pollution - An Increasing Cause for Concern. Mar. Pollut. Bull. 2003, 46, 275–280. DOI: 10.1016/S0025-326X(02)00423-X.
- Rosário Filho, N. A.; Urrutia-Pereira, M.; D'Amato, G.; Cecchi, L.; Ansotegui, I. J.; Galán, C.; Pomés, A.; Murrieta-Aguttes, M.; Caraballo, L.; Rouadi, P.; et al. Air Pollution and Indoor Settings. World Allergy Organ J. 2021, 14, 100499. DOI: 10.1016/j.waojou.2020.100499.
- Wang, W.; Gunasekaran, S. Nanozymes-Based Biosensors for Food Quality and Safety. TrAC – Trends Anal. Chem. 2020, 126, 115841. DOI: 10.1016/j.trac.2020.115841.
- Hassan, M. M.; Xu, Y.; Zareef, M.; Li, H.; Chen, Q. Recent Progress in Chemometrics Driven Biosensors for Food Application. TrAC - Trends Anal. Chem 2022, 156, 116707. DOI: 10.1016/j.trac.2022.116707.
- Akshaya, K.; Arthi, C.; Pavithra, A. J.; Poovizhi, P.; Antinate, S. S.; Hikku, G. S.; Jeyasubramanian, K.; Murugesan, R. Bioconjugated Gold Nanoparticles as an Efficient Colorimetric Sensor for Cancer Diagnostics. Photodiagnosis Photodyn. Ther. 2020, 30, 101699. DOI: 10.1016/j.pdpdt.2020.101699.
- Yang, T.; Luo, Z.; Tian, Y.; Qian, C.; Duan, Y. Design Strategies of AuNPs-Based Nucleic Acid Colorimetric Biosensors. TrAC – Trends Anal. Chem. 2020, 124, 115795. DOI: 10.1016/j.trac.2019.115795.
- Gerber, L. R.; McCallum, H.; Lafferty, K. D.; Sabo, J. L.; Dobson, A. Exposing Extinction Risk Analysis to Pathogens: Is Disease Just Another Form of Density Dependence? Ecol. Appl. 2005, 15, 1402–1414. DOI: 10.1890/04-0880.
- Yadav, N.; Chhillar, A. K.; Rana, J. S. Detection of Pathogenic Bacteria with Special Emphasis to Biosensors Integrated with AuNPs. Sensors Int. 2020, 1, 100028. DOI: 10.1016/j.sintl.2020.100028.
- Man, Y.; Ban, M.; Li, A.; Jin, X.; Du, Y.; Pan, L. A Microfluidic Colorimetric Biosensor for in-Field Detection of Salmonella in Fresh-Cut Vegetables Using Thiolated Polystyrene Microspheres, Hose-Based Microvalve and Smartphone Imaging APP. Food Chem. 2021, 354, 129578. DOI: 10.1016/j.foodchem.2021.129578.
- He, Z.; Ding, S.; Wang, L.; Wang, G.; Liang, X.; Takarada, T.; Maeda, M. Introducing DNA Nanosensor to Undergraduate Students: Rapid Non-Cross-Linking Aggregation of DNA-Functionalized Gold Nanoparticles for Colorimetric DNA Assay. J. Chem. Educ. 2021, 98, 3553–3559. DOI: 10.1021/acs.jchemed.1c00605.
- Marin, M.; Nikolic, M. V.; Vidic, J. Rapid Point-of-Need Detection of Bacteria and Their Toxins in Food Using Gold Nanoparticles. Compr. Rev. Food Sci. Food Saf. 2021, 20, 5880–5900. DOI: 10.1111/1541-4337.12839.
- Jiao, Y.; Liu, Q.; Qiang, H.; Chen, Z. Colorimetric Detection of L-Histidine Based on the Target-Triggered Self-Cleavage of Swing-Structured DNA Duplex-Induced Aggregation of Gold Nanoparticles. Microchim. Acta 2018, 185, 1–6. DOI: 10.1007/s00604-018-2987-z.
- Gupta, R.; Kumar, A.; Kumar, S.; Pinnaka, A. K.; Singhal, N. K. Naked Eye Colorimetric Detection of Escherichia coli Using Aptamer Conjugated Graphene Oxide Enclosed Gold Nanoparticles. Sens. Actuators B Chem. 2021, 329, 129100. DOI: 10.1016/j.snb.2020.129100.
- Yao, S.; Li, J.; Pang, B.; Wang, X.; Shi, Y.; Song, X.; Xu, K.; Wang, J.; Zhao, C. Colorimetric Immunoassay for Rapid Detection of Staphylococcus Aureus Based on Etching-Enhanced Peroxidase-like Catalytic Activity of Gold Nanoparticles. Microchim. Acta 2020, 187, 1–8. DOI: 10.1007/s00604-020-04473-7.
- Du, J.; Yu, Z.; Hu, Z.; Chen, J.; Zhao, J.; Bai, Y. A Low PH-Based Rapid and Direct Colorimetric Sensing of Bacteria Using Unmodified Gold Nanoparticles. J. Microbiol. Methods 2021, 180, 106110. DOI: 10.1016/j.mimet.2020.106110.
- You, Q.; Zhang, X.; Wu, F. G.; Chen, Y. Colorimetric and Test Stripe-Based Assay of Bacteria by Using Vancomycin-Modified Gold Nanoparticles. Sens. Actuators B Chem. 2019, 281, 408–414. DOI: 10.1016/j.snb.2018.10.103.
- Chen, Q.; Gao, R.; Jia, L. Enhancement of the Peroxidase-like Activity of Aptamers Modified Gold Nanoclusters by Bacteria for Colorimetric Detection of Salmonella typhimurium. Talanta 2021, 221, 121476. DOI: 10.1016/j.talanta.2020.121476.
- Fu, K.; Zheng, Y.; Li, J.; Liu, Y.; Pang, B.; Song, X.; Xu, K.; Wang, J.; Zhao, C. Colorimetric Immunoassay for Rapid Detection of Vibrio parahemolyticus Based on Mn2+ Mediates the Assembly of Gold Nanoparticles. J. Agric. Food Chem. 2018, 66, 9516–9521. DOI: 10.1021/acs.jafc.8b02494.
- Liu, Y.; Wang, X.; Shi, X.; Sun, M.; Wang, L.; Hu, Z.; Liu, F.; Liu, Q.; Wang, P.; Li, J.; et al. A Colorimetric Sensor for Staphylococcus Aureus Detection Based on Controlled Click Chemical-Induced Aggregation of Gold Nanoparticles and Immunomagnetic Separation. Microchim. Acta 2022, 189, 104. DOI: 10.1007/s00604-022-05211-x.
- Ahmadi, S.; Kamaladini, H.; Haddadi, F.; Sharifmoghadam, M. R. Thiol-Capped Gold Nanoparticle Biosensors for Rapid and Sensitive Visual Colorimetric Detection of Klebsiella Pneumoniae. J. Fluoresc. 2018, 28, 987–998. DOI: 10.1007/s10895-018-2262-z.
- Quintela, I. A.; De Los Reyes, B. G.; Lin, C. S.; Wu, V. C. H. Simultaneous Colorimetric Detection of a Variety of Salmonella Spp. In Food and Environmental Samples by Optical Biosensing Using Oligonucleotide-Gold Nanoparticles. Front. Microbiol. 2019, 10, 1138–1112. DOI: 10.3389/fmicb.2019.01138.
- Elliott, C. N.; Becerra, M. C.; Bennett, J. C.; Graham, L.; Silvero C, M. J.; Hallett-Tapley, G. L. Facile Synthesis of Antibiotic-Functionalized Gold Nanoparticles for Colorimetric Bacterial Detection. RSC Adv. 2021, 11, 14161–14168. DOI: 10.1039/d1ra01316e.