Abstract
As a model organism that has helped revolutionize life sciences, Caenorhabditis elegans has been increasingly used in nutrition research. Here we explore the tradeoffs between pros and cons of its use as a dietary model based primarily on literature review from the past decade. We first provide an overview of its experimental strengths as an animal model, focusing on lifespan and healthspan, behavioral and physiological phenotypes, and conservation of key nutritional pathways. We then summarize recent advances of its use in nutritional studies, e.g. food preference and feeding behavior, sugar status and metabolic reprogramming, lifetime and transgenerational nutrition tracking, and diet–microbiota–host interactions, highlighting cutting-edge technologies originated from or developed in C. elegans. We further review current challenges of using C. elegans as a nutritional model, followed by in-depth discussions on potential solutions. In particular, growth scales and throughputs, food uptake mode, and axenic culture of C. elegans are appraised in the context of food research. We also provide perspectives for future development of chemically defined nematode food (“NemaFood”) for C. elegans, which is now widely accepted as a versatile and affordable in vivo model and has begun to show transformative potential to pioneer nutrition science.
Introduction
Humans and animals require sufficient nutrients to support their life activities, including growth, development, reproduction, movement and survival. A balanced diet ensures the intake of essential nutrients that provide adequate energy and substances for these activities, while either deficiency or excess of nutrients may cause suboptimal functioning of the body, leading to metabolic stress and associated disorders (GBD 2017 Diet Collaborators Citation2019; Lepre et al. Citation2021). In the last few decades, lifestyle routines and eating habits have drastically changed, including substantial shift from home‐made to industrial food, due to the rapid development of food production and the globalization of food supply. This transition is accompanied by significant changes in dietary intake with a modified nutritional pattern thanks to the increased availability of food, especially energy-dense food. The resulting nutritional imbalance and energy surplus, as opposed to evolutionarily adapted mechanisms of food shortage, are particularly alarming as early suboptimal nutrition may have long-term health consequences several decades even generations later (Gentile and Weir Citation2018; Jiménez-Chillarón et al. Citation2012; Robinson Citation2018). Indeed, increasing evidence suggests that nutritional memory from earlier life stages of an individual per se, as well as transgenerational metabolic memory of ancestral food experience, may have important impacts on metabolic physiology, including food consumption and nutritional balancing strategies, which in turn will affect health and disease (Barrès and Zierath Citation2016; Fontana and Partridge Citation2015; Hahn et al. Citation2019; Perez and Lehner Citation2019). However, convenient models for nutrition studies in such contexts still need further development to unravel long-term and cross-generational beneficial as well as adverse effects and underlying molecular mechanisms, including signaling, transcriptional and epigenetic regulations.
The experimentally tractable nematode Caenorhabditis elegans has been widely used as a model animal that has helped revolutionize our understanding in virtually all aspects of life sciences for half a century (C. elegans Sequencing Consortium Citation1998), and has also emerged as a favorable model in food and nutrition studies (Cypser, Kitzenberg, and Park Citation2013; Gottschling and Döring Citation2019; Shen et al. Citation2018; Zečić, Dhondt, and Braeckman Citation2019). As summarized in , C. elegans has been used in recent years to investigate almost every category of nutrients, including carbohydrates, proteins, lipids, vitamins and minerals. For example, wild-type as well as various transgenic C. elegans models are used to assess the bioactivities of polysaccharides, peptides and other extracts derived from food (e.g. Chen et al. Citation2020; Guo et al. Citation2020; Jia et al. Citation2018; Li et al. Citation2011; Lu et al. Citation2021; Wang et al. Citation2016; H. Zhang et al. Citation2012; J. Zhang et al. Citation2016; Zhong et al. Citation2021). Given the data available thus far, it is entirely plausible to foresee the transformative power of C. elegans in pioneering nutrition science, e.g. lifelong monitoring and transgenerational tracking of nutritional status. To appreciate the groundbreaking potential, however, a number of inadvertent compromises must be fully realized to forestall misuses of C. elegans models. Here we focus on the feasibility and advantages as well as limitations and solutions of using C. elegans as a “dietary” model in nutritional studies.
Table 1. Bioactivities of food nutrients and functional components investigated in C. elegans models.a
Experimental strengths of C. elegans as a nutritional model
As a major model organism in life sciences and biomedical research, C. elegans is powerful at both molecular and organismal levels and has a number of advantages such as small size (∼1 mm in length with 959 cells for adult hermaphrodites), rapid life cycle (∼3 days), short lifespan (∼3 weeks), convenience of laboratory maintenance (agar plates or liquid cultures), ease of experimental manipulation (e.g. 96- and 384-well plates), and genetic similarity to human beings (containing potential counterparts of most human disease genes and signaling networks). Despite its small size, this seemingly simple nematode model does have diverse tissues, e.g. nervous, muscle, intestinal, and reproductive systems. In addition, access to vast online resources is publicly available, including specialized knowledge as well as genetic and strain resources such as WormBook (http://www.wormbook.org), WormBase (https://wormbase.org) and CGC (https://cbs.umn.edu/cgc/home; i.e. Caenorhabditis Genetics Center).
Take as an example healthspan, which is becoming a central topic in aging and nutritional studies (Lee et al. Citation2021; Olshansky Citation2018; Scott, Ellison, and Sinclair Citation2021; Seals, Justice, and LaRocca Citation2016; Wickramasinghe et al. Citation2020). Healthspan can be defined as the period of life that an organism is free from major diseases and disabilities. As a leading model in aging research, C. elegans has been increasingly used to help distinguish between lifespan and healthspan (e.g. Bansal et al. Citation2015; Huang, Ma, et al. Citation2022; Möller et al. Citation2020), both of which are essential aspects of aging that can be regulated by dietary manipulation (Lee et al. Citation2021; Wickramasinghe et al. Citation2020). In consensus with the concept in other organisms, three main categories of health indicators have been developed in C. elegans healthspan, i.e. cell and tissue integrity, tissue and organ function, and physiological and stress resilience (Bansal et al. Citation2015; Hahm et al. Citation2015; Keith et al. Citation2014; Koopman et al. Citation2020).
A number of behavioral and physiological phenotypes have been adopted for healthspan studies in C. elegans, including locomotion, body bends, pharyngeal pumping, and body size (Bansal et al. Citation2015; Ewald, Castillo-Quan, and Blackwell Citation2018; Seo et al. Citation2018). The highly coordinated and tightly regulated pharyngeal pumping is an essential action of C. elegans for food intake. Illustrated in is a representative image of C. elegans pharynx. Feeding is accomplished by cycles of contraction and relaxation in the pharynx, where the corpus sucks in and concentrates food while the terminal bulb grinds it up and transports it to the intestine (Avery and Shtonda Citation2003; Avery and You Citation2012). The pharyngeal pumping rate, which is modulated by food availability and thus a reliable measure of nutritional status, can be measured by directly counting the visible pumps under a microscope and used as a reliable and quantitative indicator of C. elegans health (Lemieux et al. Citation2015; Williams et al. Citation2019). Another essential indicator of health is body size and body shape, which is of utmost importance for food and nutrition studies. We have previously shown that paeoniflorin is able to delay progressive paralysis of C. elegans caused by the expression of amyloid-β peptide (Aβ), but it does not affect their body size as analyzed by a COPAS Biosort system using large number of animals, including both time of flight (TOF; equivalent to nematode length) and extinction (EXT; indicative of internal structure of nematodes) (), implying no compromise on the health of C. elegans (Ai et al. Citation2018).
Figure 1. Pharyngeal pump and body size of Caenorhabditis elegans. (A) Microscopy of pharynx indicating pharyngeal pumping and food transport in C. elegans. A representative image of pharynx (about 100 × 20 μm in adult hermaphrodites) is shown to indicate the neuromuscular pump that connects to the mouth at the anterior end via the corpus and to the intestine at the posterior end via the terminal bulb. Scale bar, 40 μm. (B) Effect of paeoniflorin on body size of C. elegans. Wild-type nematodes are treated with paeoniflorin at the indicated concentrations, and their TOF and EXT in a COPAS Biosort system are measured (n > 100 animals per group). Adapted from original data that support the findings in Ai et al. (Citation2018). AU, arbitrary units.
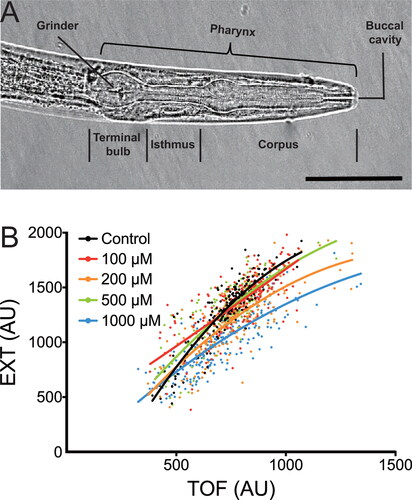
Conservation of key nutritional pathways in C. elegans
To maximize health and survival, animals must maintain a nutritional balance in line with nutrient availability, which may activate a number of signal transduction networks to modulate various biochemical processes of fundamental importance, including metabolism, stress response, aging and disease (Chantranupong, Wolfson, and Sabatini Citation2015; Efeyan, Comb, and Sabatini Citation2015; Templeman and Murphy Citation2018). Many of these signaling cascades are highly conserved across animal kingdom, including the nematode model C. elegans (Rashid et al. Citation2020; Rubio-Aliaga Citation2012). For example, the phylogenetically conserved insulin/insulin-like growth factor (IGF) signaling (IIS) pathway is known to play pivotal roles in dietary response, metabolic control and lifespan determination. The main signaling components of this pathway in C. elegans are DAF-2, AGE-1, AKT and DAF-16, which are homologous to insulin/IGF receptor (IGFR), phosphoinositide 3-kinase (PI3K), protein kinase B (PKB) and forkhead box O (FOXO) transcription factor, respectively, in humans (López-Otín et al. Citation2013; Xiao et al. Citation2022; Zhang et al. Citation2012).
The AMP-activated protein kinase (AMPK), a serine/threonine protein kinase ubiquitously expressed in eukaryotic cells, is a critical sensor of energy status that controls the response of several energy-related pathways to energy depletion, nutritional cues and other signals (Herzig and Shaw Citation2018; López et al. Citation2016). As the energy gauge of eukaryotes, AMPK plays an important role in cellular metabolism and metabolic diseases, including uptake and metabolism of sugars and fatty acids (Day, Ford, and Steinberg Citation2017; Herzig and Shaw Citation2018; Kahn et al. Citation2005). Another serine/threonine protein kinase known as target of rapamycin (TOR) or mammalian/mechanistic TOR (mTOR) is also a major nutrient sensor evolutionarily conserved in eukaryotes (Chantranupong, Wolfson, and Sabatini Citation2015). TOR/mTOR is known to play an integral role in response to signals from IIS and AMPK as well as nutrients (e.g. leucine and arginine), and serves as a master regulator of metabolic homeostasis and cell growth (Blackwell et al. Citation2019; Kim and Guan Citation2019; Saxton and Sabatini Citation2017).
Another well-characterized pathway linked to nutrient signaling involves the mitogen-activated protein kinases (MAPK), including c-Jun N-terminal kinases (JNK), extracellular signal-regulated kinases (ERK) and p38 kinases, which are widespread in eukaryotes from invertebrates to mammals and have diverse physiological functions such as stress and metabolic responses (Darling and Cook Citation2014; Huang and Tunnacliffe Citation2004; Huang, Banton, and Tunnacliffe Citation2010; Nikolic, Leiva, and Sabio Citation2020). Phylogenetic and functional analysis of C. elegans has identified four JNK homologs (JNK-1, KGB-1, KGB-2 and C49C3.10), two ERK homologs (MPK-1 for ERK1/2 and MPK-2 for the rare ERK5) and three p38 MAPK homologs (PMK-1, PMK-2 and PMK-3) (Andrusiak and Jin Citation2016; Heger et al. Citation2010; Lackner et al. Citation1994), providing invaluable models to study MAPK associated nutrition signalings as demonstrated for peptides, polysaccharides and other functional food components (Li et al. Citation2017; Xiao et al. Citation2022; Yoon et al. Citation2019).
Many other signal transduction pathways of nutritional and metabolic importance are also conserved in C. elegans. For example, the lifespan-extending effect of caloric restriction, an extensively studied dietary regimen across species, has been well established in C. elegans. The highly conserved molecular signature of dietary restriction is found not only closely associated with the aforementioned IIS and TOR/mTOR pathways but also related to other conserved signaling cascades such as the NAD-dependent protein deacetylase SIR-2.1 (homologous to human SIRT1) and the stress response transcription factor SKN-1 (homolog of human Nrf2) as well as conserved epigenetic mechanisms (Bishop and Guarente Citation2007; Hernández-Saavedra et al. Citation2019; Kapahi, Kaeberlein, and Hansen Citation2017; Lakowski and Hekimi Citation1998). Taken together, the strong conservation of these signaling principles and the extensive experimental investigations in C. elegans make this invertebrate animal a convenient yet elegant in vivo model for nutrition biology studies and also a highly promising tool to provide further insights into molecular mechanisms of human nutrition.
Perception and consumption of food in C. elegans
Food consumption is essential for animals and humans to maintain physiological integrity and live in a healthy state, but food supplements may have different impacts on their behaviors, including feeding performance. C. elegans has a number of conserved food-intake regulation mechanisms orthologous to humans, and thus has been used as a model system to study feeding behaviors and phenotypes (Gomez-Amaro et al. Citation2015; Rodríguez-Palero et al. Citation2018). When they encounter unfavorable or harmful food, their food intake will be reduced, which may affect their survival and fecundity and result in an altered food clearance rate in a short period of time (Voisine et al. Citation2007). Remarkably, C. elegans has been shown to exhibit dietary choice for “good” food and “bad” food through mechanisms such as pheromones (Greene et al. Citation2016; Shtonda and Avery Citation2006), and is recently found even to discriminate colors to guide their foraging decisions for bacterial food (Ghosh et al. Citation2021).
The perception of food has evolved as one of the essential sensory functions in animals, and has been the subject of considerable studies in animals and humans. An example methodology to study food perception in C. elegans is illustrated in , which shows that either binary choice assays or multiple choice experiments can be used to determine food preference, including differentiation of spiked compounds as well as pathogenic bacteria in food (Zhang, Lu, and Bargmann Citation2005). Both tests can be easily performed in a single assay plate, and food preference can be expressed as choice index () or choice percentage (). Thanks to its sensitivity to a variety of different substances such as food additives, pesticides, heavy metals and toxins, C. elegans has been increasingly used as an alternative model animal to test the efficacy and safety of food ingredients, especially in early and rapid assessments (Ma et al. Citation2019; M. Zhang, Li, et al. Citation2019; Zhang et al. Citation2021). For example, C. elegans has been used as a model in nutrition research to detect various neurodevelopmental toxins (Hunt et al. Citation2018).
Figure 2. Experimental design for food choice assay in Caenorhabditis elegans. (A) Binary choice assay. Food selectivity can be assessed by measuring the olfactory preference of C. elegans. The test plate is divided into two different food zones, e.g. a zone seeded with E. coli OP50 as control food and a zone of E. coli OP50 with supplements as test food. Approximately 100–200 animals are placed at the center of the plate, allowed to move freely for a period of time (e.g. 10 h at 20 °C), and scored in each zone. A choice index for a food is calculated according to the equation shown, where an index of 1.0, -1.0 and 0 indicates complete preference, complete avoidance and no preference of the food, respectively. (B) Multiple choice assay. In multiple choice experiments, the test plate is divided into several zones for different foods. The animals are placed at the center of the plate, roughly equidistant from each zone, allowed to move, and scored in each zone as in (A). Food preference is expressed as a percentage of animals in the food zone relative to total animals.
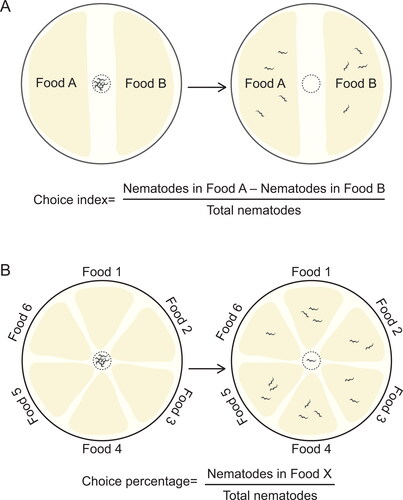
Sugar status and metabolic reprogramming in C. elegans
Animals and humans primarily use sugars to produce energy, but different amounts and types consumed may have profoundly different consequences despite undisputed importance of sugars in health (Clemens et al. Citation2016). For example, high glucose has been found to shorten the lifespan of C. elegans (Lee, Murphy, and Kenyon Citation2009; Schlotterer et al. Citation2009), while glucose restriction (Schulz et al. Citation2007) and trehalose supplementation (Honda, Tanaka, and Honda Citation2010) are shown to extend its lifespan. When fed with high-glucose diets, the biochemical and physiological changes of C. elegans are indeed prominent, including lifespan, stress resistance, reproduction, body size, fat content, and glycogen storage (see examples listed in ).
It has been established that the insulin signaling pathway, i.e. the DAF-2/IGFR–AGE-1/PI3K–AKT/PKB–DAF-16/FOXO axis, plays an important role in the response and adaptation of C. elegans to different type and different amount of sugars, including remodeling of carbohydrate and lipid metabolisms (Depuydt et al. Citation2014; Forsythe et al. Citation2006; Hanover et al. Citation2005; Hibshman et al. Citation2017; Seo et al. Citation2018). For example, triglycerides are the main energy storage in wild-type (N2) C. elegans under normal conditions, while glycogen storage and use are increased in daf-2 mutants (Seo et al. Citation2018). When glycogen is suppressed, trehalose storage is increased. Interestingly, the lifespan and healthspan of C. elegans are increased upon this metabolic shift from glycogen to trehalose (Seo et al. Citation2018). In particular, the metabolic shift between glycogen and trehalose is shown to play a part in development and stress responses, including oxidative stress and starvation, via the DAF-2–DAF-16 insulin pathway (Gusarov et al. Citation2017; Hibshman et al. Citation2017; Penkov et al. Citation2020).
Lifetime and transgenerational nutrition tracking in C. elegans
Due to nutritional memory, early-life dietary intervention may affect the health and fitness outcomes of animals and humans later in life even after resuming normal diet, and this may also have an impact on their progeny (Hahn et al. Citation2019; Jobson et al. Citation2015; Mautz, Lind, and Maklakov Citation2020). With a short life cycle and lifespan, C. elegans can be easily exposed to different dietary conditions at any particular stage of development and monitor its nutritional response for a specific period of time, including lifelong and cross-generational tracking. A notable example is the above-mentioned adverse effects of high glucose, which is the major energy source and also a key regulator of metabolism. Some of these effects are heritable in C. elegans as transgenerational phenotypes are observed in the next generations of progeny despite only the first generation being exposed to high glucose (Alcántar-Fernández et al. Citation2018, Citation2019; Tauffenberger and Parker Citation2014). Another example is the studies on starvation throughout the life cycle of C. elegans as recently reviewed (Baugh and Hu Citation2020). Extended period of food deprivation at larval stages is found to have long-term phenotypic consequences on growth, development, reproduction and survival in later life and also induces transgenerational inheritance (Jobson et al. Citation2015; Jordan et al. Citation2019; Rechavi et al. Citation2014; Webster et al. Citation2018).
While starvation is basically a prolonged food deprivation with adverse consequences, dietary restriction is in essence a measured starvation resulting in low caloric intake but avoiding malnutrition, which is known to promote lifespan extension across diverse species, including C. elegans, Drosophila and mouse. However, this caloric intervention paradigm is recently shown to improve the organismal fitness of C. elegans in later life but reduce the fitness of their progeny, indicating an intergenerational tradeoff of fitness between parent and progeny nematodes (Mautz, Lind, and Maklakov Citation2020). On the other hand, the descendants of severely starved larvae are shown to be more resistant to starvation and stress than that of the nematodes not starved in the first generation (Jobson et al. Citation2015; Jordan et al. Citation2019). The transgenerational inheritance of these starvation phenotypes is found to be mediated by small RNA molecules, which act on genes associated with nutrient-response genes (Rechavi et al. Citation2014), and vitellogenin-mediated intergenerational transmission of IIS-to-IIS signals, which convey adaptation to diet availability (Jordan et al. Citation2019). Interestingly, the link between glucose metabolism and epigenetic signaling has also been established, which is likely to be mediated by the glucose-derived metabolite acetyl-CoA (Wellen et al. Citation2009). Collectively, these studies support transgenerational memory of ancestral dietary conditions and also adaptive modulation of epigenetic machinery by nutrition.
C. elegans as a simplified model for studies of diet–microbiota–host interactions
It is well recognized that health and disease are under the direct influence of microbial communities associated with humans and animals (Fontana and Partridge Citation2015; Leulier et al. Citation2017; Suzuki and Ley Citation2020; The Integrative HMP (iHMP) Research Network Consortium Citation2019). For example, gut microbiota is found to be a critical determinant of animal physiology and as a whole is linked to many aspects of host health. However, the functional interactions between food ingredients, which are the key regulators of host as well as microbial physiology, and gut microbiota (including their metabolites) still remain to be elucidated. In particular, further dissection of the intricate yet robust relationships between gut microbes and host nutrition following a more holistic approach would reveal the dynamic roles of nutrients in balancing gut microbiota and host metabolism, providing a comprehensive insight into microbial management in health and disease.
Until now, most research involving C. elegans has been performed on nematodes fed with standard monoxenic bacterial diet, usually E. coli strain OP50 on solid NGM plates and strain NA22 or HB101 in liquid culture (), essentially in the absence of other bacteria. This type of C. elegans model does have an advantage in testing the direct functions and mechanisms of food ingredients. Since both C. elegans and E. coli are well-characterized model organisms, this monoxenic pattern of host with single-species bacteria can also serve as a simplistic but tractable “microbiota” model, in which the standard OP50 bacteria can be easily substituted by or cocultivated with other bacteria (Cabreiro and Gems Citation2013; Calvo et al. Citation2016; Gerbaba, Green-Harrison, and Buret Citation2017; Ortiz de Ora and Bess Citation2021). For example, C. elegans has shown significant metabolic, defense and transcriptomic response changes when grown at different temperatures (15, 20 and 25 °C), but distinct patterns of response to temperatures are observed when its food is changed from the standard E. coli diet to Bacillus subtilis (Gómez-Orte et al. Citation2018). Interestingly, the lifespan of C. elegans has been reported to be greater when fed on B. subtilis than on E. coli because of the differences in bacterially-derived metabolites such as nitric oxide and coenzyme Q, which may affect longevity-associated signaling pathways such as redox homeostasis, HSF-1 and DAF-16 (Garsin et al. Citation2003; Gusarov et al. Citation2013; Sánchez-Blanco et al. Citation2016).
Figure 3. Growth of Caenorhabditis elegans at different scales and throughputs. (A) Laboratory maintenance and standard culture of C. elegans. Routine culture of C. elegans is maintained on NGM agar plates seeded with a lawn of E. coli OP50 as food source. The nematodes are usually grown at 20 °C unless otherwise specified. (B) Mass culture of C. elegans on peptone-rich or egg yolk-enriched solid NGM. To obtain biochemical or larger quantities, C. elegans can be grown in mass cultures using peptone-rich or chicken egg yolk-enriched NGM trays seeded with a thick layer of E. coli NA22 as food. (C) Large-scale growth of C. elegans in liquid culture. Large quantities of nematodes can be cultured in flasks up to liters and decaliters using S medium with E. coli NA22 (or HB101) as food source or axenic liquid nematode medium. (D) Liquid culture of C. elegans for high-throughput investigations. C. elegans can be grown in 96- or 384-well plates with S medium and bacterial food or with axenic liquid nematode medium, and thus can be conveniently used in high-throughput investigations of nutritionally and pharmacologically important compounds.
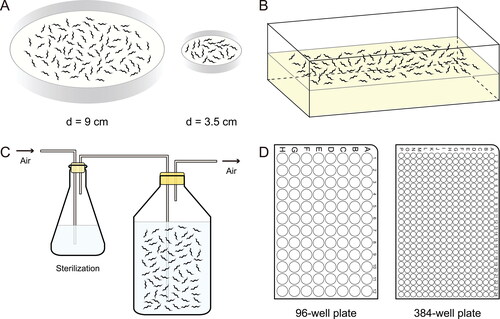
Like in humans, microbial communities associated with C. elegans are also shown to play a critical role in its physiology, making it a promising model for mechanistic studies of gut microbiota (Dirksen et al. Citation2020; Gerbaba, Green-Harrison, and Buret Citation2017; O’Donnell et al. Citation2020; Shapira Citation2017; Zhang, Holdorf, et al. Citation2017). For example, multiple species of commensal Providencia bacteria are found to colonize the gut and alter the olfactory behavior of C. elegans, promoting fitness of both the host and the bacteria (O’Donnell et al. Citation2020). Further investigation suggests that the sensory alteration is caused by the bacterially produced tyramine, which is a neuromodulator that can be used by C. elegans to modulate olfactory response via ASH nociceptive neurons, demonstrating a gut–microbiota–brain signaling in the nematodes (O’Donnell et al. Citation2020). On the other hand, a consortium of foodborne lactic acid bacteria has been used to feed C. elegans and different bacteria are found to have differential gut colonization capacities (Zanni et al. Citation2015). Intriguingly, supplementation of the bacterial consortium is shown to decrease lifespan, reduce brood size, accumulate lipid droplets, and alter expression of obesity-associated genes such as nhr-49, pept-1 and tub-1, as compared to the nematodes fed the standard E. coli diet or probiotic bacterial strain (Zanni et al. Citation2015).
Unlike the monoxenically fed C. elegans, however, their wild counterparts are found to harbor a microbial community of diverse species in the gut. Interestingly, the gut microflora is conserved in wild C. elegans from various natural environments of distinct geographical locations, which appears to serve as a core microbiota beneficial to the nematodes, including fitness and adaptation (Berg et al. Citation2016; Dirksen et al. Citation2016; Samuel et al. Citation2016; Schulenburg and Félix Citation2017). Based on these investigations of natural microflora, CeMbio, a simplified model of C. elegans microbiota, has been recently established and become publicly available (Dirksen et al. Citation2020). CeMbio includes a set of 12 bacteria of different families, representing the core microbiota taxa of natural environment (Dirksen et al. Citation2020). Such a gnotobiotic model of C. elegans with native microbiota will undoubtedly help understand nematode biology, including metabolism, immunity, fertility, aging and gut–brain axis (Dirksen et al. Citation2020; O’Donnell et al. Citation2020; Ortiz de Ora and Bess Citation2021; Shapira Citation2017). Together with the small size and genetic tractability of C. elegans host, this type of simplified model is expected to become commonplace in microbiota studies, including high-throughput investigations of dynamic diet–microbiota–host interactions, which is expected to advance our understanding of how dietary nutrients are integrated by microbiota and host to coordinate nutrient homeostasis and thus affect health and disease.
In summary, many cutting-edge technologies originated from or developed in C. elegans, e.g. genome-wide RNA interference (RNAi), have revolutionized biomedical research in general and are increasingly contributing to nutritional biology in particular. Indeed, as a versatile and affordable in vivo model, C. elegans can be conveniently and effectively used in almost every aspect of nutrition science at molecular, cellular and organismal levels, e.g. integrated investigation on the nutritional modulation of gut-brain axis.
Limitations and solutions of using C. elegans as a food and nutrition model
As exemplified above and reported in an increasing body of literature (e.g. those listed in ), significant progress in food and nutrition studies has been made using C. elegans models. We envision that continued innovation in technology combined with the inherent strengths of C. elegans model, such as genome-editing, genome-wide association study (GWAS), in vivo high-throughput, in vivo microfluidic, and automatic lifetime behavioral tracking technologies, will drive further development in food and nutrition research. However, a number of issues related to nutritional studies need to be adequately addressed before we can bring the well-established knowledge and leading-edge technologies into full play. Pho and MacNeil (Citation2019) have recently discussed the potential causes of experimental variability in C. elegans biology between laboratories and between experiments, including variation of bacterial diet, inconsistency of growth media and genetic background of animal population per se. Here we focus on laboratory cultivation and dietary standardization with an emphasis on feeding mode and growth scales.
Culture of bacteria-fed C. elegans at different scales and throughputs
In wild, C. elegans is a nematode known to prey conservatively but selectively on bacteria as mentioned above. In laboratory, feeding C. elegans with the uracil auxotroph E. coli OP50 on solid NGM plates has become a canonical protocol for maintenance and most experiments ()—growth of OP50 on NGM is limited, allowing easier handling and observation of the nematodes (Brenner Citation1974). This cost-effective cultivation is typically carried out in 9 cm petri dishes, but 3.5 cm and 15 cm plates are also used depending on specific experiments. However, when large quantities of nematodes become necessary, the routine NGM culture plates may not suffice to meet the need. To this end, C. elegans can be grown in big trays using peptone-rich or chicken egg yolk-enriched solid NGM seeded with the E. coli NA22 as food, growing in thick layers () (Miedel et al. Citation2014; Strange, Christensen, and Morrison Citation2007). Alternatively, large-scale growth of C. elegans can be achieved in liquid culture using S medium and E. coli strain NA22 or HB101 as food, which can be performed in flasks at the scale of liters and decaliters. To avoid food and oxygen shortages, the culture is gently but continuously aerated with chemically or filter sterilized ambient air (), and more bacteria can be added before they are depleted to ensure food supply. Axenic liquid nematode medium can also be used in large-scale culture of C. elegans, but further development and practice are needed.
In addition to growing nematodes in large quantities, liquid culture has also become increasingly common in laboratory for better control of dietary supplements, including nutritionally and pharmacologically important compounds (Hibshman, Webster, and Baugh Citation2021; Lev et al. Citation2019). In particular, C. elegans can be grown in liquid culture with S medium in 96- or 384-well plates if high-throughput investigation is to be performed (). Particular attention should be paid to the amount of food, the density of nematodes, and the total volume per well to ensure survival and avoid dietary restriction of the animals (Wang et al. Citation2021). In addition to bacterial feeding, axenic liquid culture of C. elegans is also a promising alternative that can be used in high-throughput investigations.
Uptake of dietary supplements in bacteria-fed C. elegans culture
Caenorhabditis elegans is known to obtain compounds from its surroundings through three routes, i.e. ingestion by the pharynx, absorption via the skin-like cuticle, and uptake through the exposed neuronal endings (Kaletta and Hengartner Citation2006). In practice, supplementation of functional ingredients, including nutrients and therapeutics, to bacteria-fed C. elegans can be done by adding to the medium (e.g. NGM or S Medium), directly spreading on the NGM plates, or mixing with the bacterial food and then spreading on the NGM plates. For convenience, many studies directly add supplements into the NGM agar medium or the liquid S Medium (e.g. Koseki et al. Citation2020). In the case of NGM supplementation, however, it is difficult to know how much of the supplemented compounds is taken by the nematodes. It may end up with very high “doses” to show desired nutritional effects particularly if the supplements are inside the NGM agar, while high concentrations may on the other hand cause osmotic pressure, affecting the outcomes of experiments (Koseki et al. Citation2020; Lublin and Link Citation2013; Zheng et al. Citation2013).
Whilst nutrients added in the liquid medium can be uptaken by ingestion through the pharynx, the supplements added inside NGM agar are likely to be taken by absorption via cuticle and exposed neuronal cilia rather than by direct pharyngeal ingestion, particularly for small-molecule compounds. This is, in the common sense, not “food” intake and ingestion but may nevertheless still be misinterpreted as “dietary” data. In this regard, supplementation of E. coli OP50 food with functional ingredients is a better option. Yet, metabolic interference from the bacteria may preclude direct examination on the nutrients of interest. For this purpose, the live bacterial food can be replaced with dead OP50 E. coli killed by UV irradiation or heat (e.g. Cabreiro et al. Citation2013; Seo et al. Citation2018; Wood et al. Citation2004). However, special attention should be paid to the preparation of UV- and, in particular, heat-killed bacteria so that essential nutrients are retained in the bacterial food; otherwise dietary restriction may arise.
Distinct effects of different bacterial strains as food on C. elegans
As indicated above, wild C. elegans forages for a variety of bacteria as food in its natural habitat, and different bacterial food may have distinct effects on the nematodes, including fitness, lifespan, reproduction and stress response (e.g. Coolon et al. Citation2009). These findings suggest that there may exist differential nutrients and bioactive metabolites in the bacteria. Under laboratory conditions, C. elegans is usually cultured on NGM agar plates seeded with a lawn of standardized E. coli bacteria, whose major macronutrient is proteins. On average, the contents of proteins, lipids and carbohydrates in E. coli cells are 55%, 7–9% and 6% respectively by dry weight, i.e. a macronutrient ratio (%) of approximately 80:10:10 (protein:fat:carbohydrate), and the overall mass levels of proteins and carbohydrates of E. coli food are roughly similar to that of C. elegans as well as mammals (Zečić, Dhondt, and Braeckman Citation2019). The content of micronutrients, including vitamins and minerals, accounts for ∼4% of bacterial dry weight, and different E. coli strains contain different levels of dietary micronutrients (e.g. folate and vitamins) to C. elegans (Zečić, Dhondt, and Braeckman Citation2019).
The macronutrients of E. coli strains commonly used as C. elegans food source in laboratory are roughly similar but with different carbohydrate contents and fatty acid compositions. For example, similar levels of proteins and fatty acids are found in the standard laboratory strain OP50 (a uracil auxotroph derived from the standard B strain), the RNAi feeding strain HT115 (an RNase III-deficient strain derived from K12 strain) and another popular laboratory E. coli strain HB101 (a hybrid of strains B and K12), but the content of total carbohydrates in OP50 is 3–5 times less than that in HT115 and HB101 strains (Brooks, Liang, and Watts Citation2009; MacNeil et al. Citation2013). HB101 can form thicker lawns than OP50 on NGM plates and is recommended for liquid cultures of C. elegans (Brooks, Liang, and Watts Citation2009; Hibshman, Webster, and Baugh Citation2021; MacNeil et al. Citation2013).
Interestingly, almost no impact is found on the lifespan of wild-type C. elegans grown on these E. coli strains (Brooks, Liang, and Watts Citation2009; Pang and Curran Citation2014; Soukas et al. Citation2009). HB101 is, however, considered to be a high-quality food due to its capability to provide better sustenance for growth rate, body size and brood size of C. elegans as compared to OP50 and OP50-derived strains (Avery and Shtonda Citation2003; Shtonda and Avery Citation2006; So, Miyahara, and Ohshima Citation2011; Sowa et al. Citation2015). While the phospholipid levels of C. elegans feeding on these E. coli strains are similar, the triacylglycerol level of OP50-fed nematodes is almost twice the amount of that in nematodes fed with HT115 or HB101 bacteria (Brooks, Liang, and Watts Citation2009), demonstrating potential reprogramming of metabolic pathways leading to metabolic adaptation of different diets. Therefore, control food settings are of paramount importance in any experiments involving bacteria-fed C. elegans.
Chemically defined medium for C. elegans culture
Dietary composition is undoubtedly a major player in animal physiology and health, and C. elegans is known to adapt to a variety of bacterial diets likely through reprogramming of metabolic pathways as aforementioned (e.g. Pang and Curran Citation2014; Watson et al. Citation2013). Therefore, potential metabolism of supplemented nutrients by the bacteria may contribute, for good or for bad, to the nutritional and pharmacological effects of the supplements under investigation, which may well be intended only to study the direct food–animal interactions. To this end and also for convenience, attempts have been made to develop defined axenic media for C. elegans culture (Lu and Goetsch Citation1993; Szewczyk, Kozak, and Conley Citation2003; Vanfleteren Citation1976), e.g. CeMM (C. elegans Maintenance Medium) and CeHR (C. elegans Habitation and Reproduction) media (), excluding bacterial metabolism-associated variables in nutrition study. It is advisable to make the chemically defined media from a series of component preparations, including vitamins and growth factor solutions, salt solutions, amino acids, nucleic acid substituents, other growth factors, and energy source (either D-glucose or potassium acetate) (Szewczyk, Kozak, and Conley Citation2003). Most of these component groups can be prepared in advance and stored frozen for up to six months. Upon use, the medium is compounded with the solutions in proportion as detailed in the supplementary protocol of Szewczyk, Kozak, and Conley (Citation2003).
Table 2. Comparison of commonly used culture methods of C. elegans.
Axenic culture of C. elegans in chemically defined liquid medium
Caenorhabditis elegans is often described as a free-living soil nematode but is typically found in rotting vegetation rather than in “pure” soil, and as a known bacterivore it may also take in plant or animal materials and yeast cells (Félix and Duveau Citation2012; Frézal and Félix Citation2015; Schulenburg and Félix Citation2017; Zečić, Dhondt, and Braeckman Citation2019). Therefore, various extracts, including those of yeast, chick embryo and liver, were included in early attempts of bacteria-free culture of C. elegans to ensure its nutritional requirements. Based on nutritional framework borrowed from E. coli diet (e.g. amino acid ratio), CbMM (Caenorhabditis briggsae Maintenance Medium) was developed as a nematode basal medium, which contains glucose, amino acids, vitamins, minerals and growth factors (Buecher, Hansen, and Yarwood Citation1966; Sayre, Hansen, and Yarwood Citation1963), and later modified as CeMM, a chemically defined axenic medium (), by adding potassium acetate or extra glucose as energy source (Lu and Goetsch Citation1993; Szewczyk, Kozak, and Conley Citation2003). Another bacteria-free medium is CeHR (), which is similar to CeMM but supplemented with either 10% or 20% milk to alleviate growth, development and fecundity of C. elegans altered in axenic liquid culture media (Flavel et al. Citation2018; Rao et al. Citation2005). The addition of milk, however, makes CeHR no longer a chemically defined medium in a strict sense.
Take CeMM as an example of defined medium, every nutrient added to the medium is quantified and each factor can be individually studied without potential interference of bacteria (Szewczyk, Kozak, and Conley Citation2003), e.g. in the discovery of novel bioactive monosaccharides (Sakoguchi et al. Citation2016a, Citation2016b). Furthermore, CeMM has been demonstrated to be better suited than bacterial food for automatic detection and long-term tracking of C. elegans, e.g. in sophisticated worm microfluidics avoiding pipeline blockage caused by bacterial aggregation (Doh et al. Citation2016), in long-term recordings of locomotor activity using infrared microbeam scattering without frequent worm transfer (Simonetta and Golombek Citation2007), or in spaceflight-related experiments (Adenle, Johnsen, and Szewczyk Citation2009; Qiao et al. Citation2013; Tee et al. Citation2017).
Adaptation of C. elegans in chemically defined liquid medium
Normal movement of C. elegans requires a layer of water surrounding its body surface. In natural habitat as well as on the standard NGM agar plates, C. elegans lives near the liquid–air interface, making it rapidly adaptable to the two distinct physical conditions. For example, the pattern of locomotion can switch quickly between crawling and swimming movements driven by S- and C-shaped body kinematics typical for solid and liquid cultures, respectively (Korta et al. Citation2007; Pierce-Shimomura et al. Citation2008). Therefore, one of the major adaptations of C. elegans to axenic liquid medium is the long-term impact of aquatic environment to their movement kinematics. In addition to behavioral changes, morphological, physiological and transcriptional differences may also arise when C. elegans is grown in liquid culture as compared to culture on agar plates (Çelen, Doh, and Sabanayagam Citation2018; Hibshman, Webster, and Baugh Citation2021; Houthoofd et al. Citation2002; Lev et al. Citation2019; Szewczyk, Kozak, and Conley Citation2003; Szewczyk et al. Citation2006). For instance, a number of differentially expressed genes in the axenic liquid media (including CeMM and CeHR) are found to correlate with the observed phenotypes (e.g. body morphology and cuticle development), highlighting the importance to standardize controls when switch between different culture media (Çelen, Doh, and Sabanayagam Citation2018; Pierce-Shimomura et al. Citation2008).
Sensory perception of food is essential for C. elegans to survive in microbe-rich natural habitats. As a powerful model organism in genetics and neurobiology, the functions of olfactory neurons of C. elegans are well established, including food search and aversive responses (e.g. avoidance of pathogens), and the sensory transduction pathways (e.g. Ras-MAPK) are conserved with higher animals (Hirotsu and Iino Citation2005). Interestingly, the olfactory neurons have been found to respond to a number of chemical cues produced specifically by bacteria (e.g. Bargmann, Hartwieg, and Horvitz Citation1993; O’Donnell et al. Citation2020; Zhang, Lu, and Bargmann Citation2005). Therefore, another adaptation of C. elegans to axenic media is the lack of potentially influential chemicals produced by the bacterial food source, e.g. the absence of bacteria may decrease the expression of sensory signaling-associated genes in nematodes grown in CeMM (Mukhopadhyay et al. Citation2008). Taken together, C. elegans shows a biological instinct to trigger molecular control mechanism underlying its robust phenotypic plasticity in response to changes in environment and nutrient.
Impact of particles on C. elegans in axenic liquid culture
It is well-known that the feeding of C. elegans depends on the rhythmic contraction of the pharynx, a neuromuscular organ that consists of 20 neurons and 20 muscle cells and functions as a pump connecting the mouth to the intestine (). Of the neurons, the MC cholinergic motoneurons are controlled by food sensation (mechanosensation) and serotonin signal and directly linked to food pumping dynamics (Avery and You Citation2012; Franks et al. Citation2006). The pharyngeal pumping rate is found to vary in response to food availability, at ∼1 pump/sec without food but ∼4 pumps/sec in the presence of bacterial food, which is considered to arise from the stimulation of the pharynx by increased bacterial particles (Franks et al. Citation2006; Lee et al. Citation2017). In wild-type C. elegans, MC neurons are key cholinergic neurons of the pharynx, which are associated with response to nutrient changes and pumping rate through acetylcholine secretion (Ishita, Chihara, and Okumura Citation2020; Zhang et al. Citation2015). Interestingly, signalings from the pharyngeal MC neurons and body wall muscles, including eat-2 (encoding an acetylcholine receptor) and cha-1 (encoding an acetylcholine synthetic enzyme), are shown to regulate the growth and development of nematodes grown in CeMM medium, suggesting the involvement of mechanical intake of nutrients by pharynx (Zhang et al. Citation2015).
CeMM is a full nematode medium with an excess of basic nutrients. Intriguingly, however, C. elegans cultured in this medium still shows suboptimal growth, a state between standard E. coli OP50 diet and dietary restriction-like phenotypes, suggesting poor nutrient intake or utilization, despite the fact that the nematodes are “healthy” in term of full growth cycle (Castelein et al. Citation2008; Lenaerts et al. Citation2008; Szewczyk et al. Citation2006; Zhang et al. Citation2015). On the other hand, apart from nutritional effects, the added milk in CeHR is also considered to provide the medium with particulate matter, which is required by C. elegans to help take up the nutrients in the liquid medium (Flavel et al. Citation2018; Zečić, Dhondt, and Braeckman Citation2019). The growth-promoting effect of added yeast extract, e.g. in AXM medium (), as well as some pure precipitated proteins may also arise at least partially from their capacity to form particulates (Zečić, Dhondt, and Braeckman Citation2019). As a matter of fact, food uptake in the nematodes is indeed mediated by the pharynx which filters food particulates from liquid prior to pumping to the intestinal system (Zečić, Dhondt, and Braeckman Citation2019), and thus stimulation of the pharynx by the particles is likely involved in promoting nutrient uptake in C. elegans.
Development of axenic NemaFood microgranules for C. elegans
As discussed above, the use of defined liquid medium is satisfactorily effective in avoiding potential metabolic interference by bacteria in food and nutrition studies, but the added nutrients are absorbed not only through the pharynx, i.e. as food “eaten,” but also through the epidermis, which in a strict sense is not food. Even in the case of pharynx-mediated feeding, lack of particles in the chemically defined liquid medium may also have negative effects on food uptake and consequently physiological status. On the other hand, nanoparticles and nanoemulsions have been successfully used as delivery vehicles to bring drugs and nutrients into the digestive system of C. elegans (Colmenares et al. Citation2016; Lucio et al. Citation2017, Citation2018; Shen et al. Citation2019). Therefore, we have microgranulated the axenic liquid medium into nano- and micron-scale particles for use as nematode food (“NemaFood”) (Huang, Xiao, et al. Citation2022). The fabrication of NemaFood submicrogranules is performed by using edible polymers, e.g. formulation of the axenic liquid medium AXM () with quaternized chitosan and alginate using the method originally intended for oral delivery of proteins in other systems (Li et al. Citation2007).
The NemaFood is used to feed C. elegans on NGM agar plate in the same fashion as the OP50 bacterial food. As shown in , the semi-defined AXM-based NemaFood appears sufficient to support the growth of C. elegans. For instance, the body shape and behavior of NemaFood-fed animals are virtually comparable with the OP50-fed counterparts on NGM solid plates, significantly superior to those grown in S. medium with NA22 bacteria or in liquid AXM. These results demonstrate, as a proof of concept, the feasibility of nano/microparticulate NemaFood, either it be prepared from chemically defined medium, semi-defined nutrients, or killed bacteria. In particular, it is promising to standardize C. elegans base diet using microparticulated holidic food comprising of defined macro- and micronutrients, which mimics not only the nutrient composition but also morphology of bacterial food and thus provides a better platform for nutritional and pharmacological research in C. elegans.
Figure 4. Axenic culture of Caenorhabditis elegans using liquid medium and microgranulated NemaFood. Representative images are shown for wild-type C. elegans grown in axenic liquid AXM medium (A) and by feeding AXM–chitosan–alginate microparticles (“NemaFood”) on solid NGM plates (B); for comparison, the nematodes grown in liquid S medium with NA22 bacteria as food (C) and by feeding OP50 bacteria on solid NGM plates (D) are also shown. Scale bar, 100 μm. The AXM liquid medium is prepared as described previously (; Lenaerts et al. Citation2008). The AXM-based NemaFood microgranules are prepared by microgranulating the AXM liquid medium into nano- and micron-scale particles with edible polymers, as exemplified here by quaternized chitosan and sodium alginate using previously described method for oral delivery of nutrients (Li et al. Citation2007).
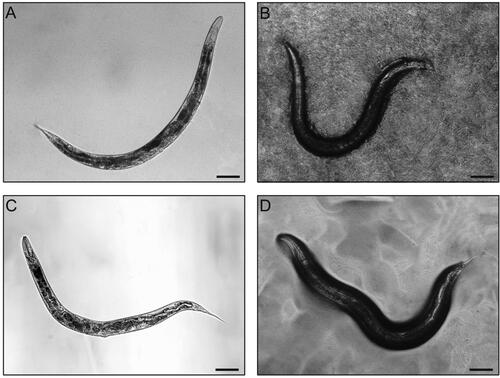
Conclusions
Caenorhabditis elegans has been used as a model animal for half a century in life sciences, and emerging evidence has begun to suggest its transformative impact on nutrition biology. The present article attempts to assemble the current knowledge on C. elegans as a nutrition model and draw a clearer picture of the tradeoffs between its strengths and limitations. Although the experimentally tractable nematode is small in size, it does have various tissues and organs, including nervous, muscle, intestinal and reproductive systems, and its key nutritional pathways and disease genes are highly conserved with humans. For example, C. elegans is known to have well conserved food-intake regulation mechanisms and displays food perception and preference. Alongside conventional culture on solid plates seeded with bacteria, C. elegans is increasingly grown in liquid culture with bacterial food at different scales and throughputs, from microliters in 384-well plates to decaliters in culture tanks. In addition, C. elegans can also grow axenically in chemically defined liquid medium, avoiding potential metabolic interference by bacteria. However, axenically grown and indeed even bacteria-fed C. elegans in liquid culture shows somewhat suboptimal, albeit healthy, growth as compared to bacteria-fed counterparts on solid plates. To eliminate experimental variability, therefore, dietary standardization and feeding mode in C. elegans culture are of great importance. In a proof-of-concept attempt, axenic liquid medium is microgranulated into microparticulate nematode food (“NemaFood”) and successfully applied to C. elegans by feeding on solid plates, essentially comparable with bacterial food. In conclusion, as an affordable in vivo model, C. elegans can be conveniently used in almost every aspect of food and nutrition research at molecular, cellular and organismal levels. It is our view that one of the major challenges to unleash the groundbreaking potential of C. elegans as a nutrition model is standardization of its base diet, which is overdue as compared to other model animals such as fruit fly, zebrafish and mice.
Disclosure statement
The authors declare no conflict of interest.
Additional information
Funding
References
- Adenle, A. A., B. Johnsen, and N. J. Szewczyk. 2009. Review of the results from the International C. elegans first experiment (ICE-FIRST). Advances in Space Research: The Official Journal of the Committee on Space Research (COSPAR) 44 (2):210–6. doi: 10.1016/j.asr.2009.04.008.
- Ai, L., F. Yang, J. Song, Y. Chen, L. Xiao, Q. Wang, L. Wang, H. Li, T. Lei, and Z. Huang. 2018. Inhibition of Abeta proteotoxicity by paeoniflorin in Caenorhabditis elegans through regulation of oxidative and heat shock stress responses. Rejuvenation Research 21 (4):304–12. doi: 10.1089/rej.2017.1966.
- Alcántar-Fernández, J., R. E. Navarro, A. M. Salazar-Martínez, M. E. Pérez-Andrade, and J. Miranda-Ríos. 2018. Caenorhabditis elegans respond to high-glucose diets through a network of stress-responsive transcription factors. PLoS One 13 (7):e0199888. doi: 10.1371/journal.pone.0199888.
- Alcántar-Fernández, J., A. González-Maciel, R. Reynoso-Robles, M. E. Pérez Andrade, A. J. Hernández-Vázquez, A. Velázquez-Arellano, and J. Miranda-Ríos. 2019. High-glucose diets induce mitochondrial dysfunction in Caenorhabditis elegans. PLoS One 14 (12):e0226652. doi: 10.1371/journal.pone.0226652.
- Andrusiak, M. G, and Y. Jin. 2016. Context specificity of stress-activated mitogen-activated protein (MAP) kinase signaling: the story as told by Caenorhabditis elegans. The Journal of Biological Chemistry 291 (15):7796–804. doi: 10.1074/jbc.R115.711101.
- Avery, L, and B. B. Shtonda. 2003. Food transport in the C. elegans pharynx. The Journal of Experimental Biology 206 (Pt 14):2441–57. doi: 10.1242/jeb.00433.
- Avery, L, and Y.-J. You. 2012. C. elegans feeding. WormBook 1–23. doi: 10.1895/wormbook.1.150.1.
- Bai, J., J. Li, R. Pan, Y. Zhu, X. Xiao, Y. Li, and C. Li. 2021. Polysaccharides from Volvariella volvacea inhibit fat accumulation in C. elegans dependent on the aak-2/nhr-49-mediated pathway. Journal of Food Biochemistry 45 (11):e13912. doi: 10.1111/jfbc.13912.
- Bansal, A., L. J. Zhu, K. Yenand, and H. A. Tissenbaum. 2015. Uncoupling lifespan and healthspan in Caenorhabditis elegans longevity mutants. Proceedings of the National Academy of Sciences of the United States of America 112 (3):E277–E286. doi: 10.1073/pnas.1412192112.
- Bargmann, C. I., E. Hartwieg, and H. R. Horvitz. 1993. Odorant-selective genes and neurons mediate olfaction in C. elegans. Cell 74 (3):515–27. doi: 10.1016/0092-8674(93)80053-H.
- Barrès, R, and J. R. Zierath. 2016. The role of diet and exercise in the transgenerational epigenetic landscape of T2DM. Nature Reviews. Endocrinology 12 (8):441–51. doi: 10.1038/nrendo.2016.87.
- Baugh, L. R, and P. J. Hu. 2020. Starvation responses throughout the Caenorhabditis elegans life cycle. Genetics 216 (4):837–78. doi: 10.1534/genetics.120.303565.
- Berg, M., B. Stenuit, J. Ho, A. Wang, C. Parke, M. Knight, L. Alvarez-Cohen, and M. Shapira. 2016. Assembly of the Caenorhabditis elegans gut microbiota from diverse soil microbial environments. The ISME Journal 10 (8):1998–2009. doi: 10.1038/ismej.2015.253.
- Bian, J., H. Zhang, S. Meng, and Y. Liu. 2018. Chemotaxis of Caenorhabditis elegans toward volatile organic compounds from Stropharia rugosoannulata induced by amino acids. Journal of Nematology 50 (1):3–8. doi: 10.21307/jofnem-2018-003.
- Bishop, N. A, and L. Guarente. 2007. Genetic links between diet and lifespan: shared mechanisms from yeast to humans. Nature Reviews. Genetics 8 (11):835–44. doi: 10.1038/nrg2188.
- Bito, T., T. Misaki, Y. Yabuta, T. Ishikawa, T. Kawano, and F. Watanabe. 2017. Vitamin B12 deficiency results in severe oxidative stress, leading to memory retention impairment in Caenorhabditis elegans. Redox Biology 11:21–9. doi: 10.1016/j.redox.2016.10.013.
- Blackwell, T. K., A. K. Sewell, Z. Wu, and M. Han. 2019. TOR signaling in Caenorhabditis elegans development, metabolism, and aging. Genetics 213 (2):329–60. doi: 10.1534/genetics.119.302504.
- Brenner, S. 1974. The genetics of Caenorhabditis elegans. Genetics 77 (1):71–94. doi: 10.1093/genetics/77.1.71.
- Brooks, K. K., B. Liang, and J. L. Watts. 2009. The influence of bacterial diet on fat storage in C. elegans. PLoS One 4 (10):e7545. doi: 10.1371/journal.pone.0007545.
- Buecher, E. J., Jr, E. Hansen, and E. A. Yarwood. 1966. Ficoll activation of a protein essential for maturation of the free-living nematode Caenorhabditis briggsae. Proceedings of the Society for Experimental Biology and Medicine 121 (2):390–3. doi: 10.3181/00379727-121-30786.
- C. elegans Sequencing Consortium. 1998. Genome sequence of the nematode C. elegans: a platform for investigating biology. Science 282 (5396):2012–8. doi: 10.1126/science.282.5396.2012.
- Cabreiro, F., C. Au, K.-Y. Leung, N. Vergara-Irigaray, H. M. Cochemé, T. Noori, D. Weinkove, E. Schuster, N. D. E. Greene, and D. Gems. 2013. Metformin retards aging in C. elegans by altering microbial folate and methionine metabolism. Cell 153 (1):228–39. doi: 10.1016/j.cell.2013.02.035.
- Cabreiro, F, and D. Gems. 2013. Worms need microbes too: microbiota, health and aging in Caenorhabditis elegans. EMBO Molecular Medicine 5 (9):1300–10. doi: 10.1002/emmm.201100972.
- Calvo, D. R., P. Martorell, S. Genovés, and L. Gosálbez. 2016. Development of novel functional ingredients: need for testing systems and solutions with Caenorhabditis elegans. Trends in Food Science & Technology 54:197–203. doi: 10.1016/j.tifs.2016.05.006.
- Castelein, N., D. Hoogewijs, A. De Vreese, B. P. Braeckman, and J. R. Vanfleteren. 2008. Dietary restriction by growth in axenic medium induces discrete changes in the transcriptional output of genes involved in energy metabolism in Caenorhabditis elegans. Biotechnology Journal 3 (6):803–12. doi: 10.1002/biot.200800003.
- Çelen, İ., J. H. Doh, and C. R. Sabanayagam. 2018. Effects of liquid cultivation on gene expression and phenotype of C. elegans. BMC Genomics 19 (1):562. doi: 10.1186/s12864-018-4948-7.
- Chantranupong, L., R. L. Wolfson, and D. M. Sabatini. 2015. Nutrient-sensing mechanisms across evolution. Cell 161 (1):67–83. doi: 10.1016/j.cell.2015.02.041.
- Chaubey, M. G., S. N. Patel, R. P. Rastogi, D. Madamwar, and N. K. Singh. 2020. Cyanobacterial pigment protein allophycocyanin exhibits longevity and reduces Aβ-mediated paralysis in C. elegans: complicity of FOXO and NRF2 ortholog DAF-16 and SKN-1. 3 Biotech 10 (8):332. doi: 10.1007/s13205-020-02314-1.
- Chen, H., S. Wang, A. Zhou, J. Miao, J. Liu, and S. Benjakul. 2020. A novel antioxidant peptide purified from defatted round scad (Decapterus maruadsi) protein hydrolysate extends lifespan in Caenorhabditis elegans. Journal of Functional Foods 68:103907. doi: 10.1016/j.jff.2020.103907.
- Clemens, R. A., J. M. Jones, M. Kern, S.-Y. Lee, E. J. Mayhew, J. L. Slavin, and S. Zivanovic. 2016. Functionality of sugars in foods and health. Comprehensive Reviews in Food Science and Food Safety 15 (3):433–70. doi: 10.1111/1541-4337.12194.
- Colmenares, D., Q. Sun, P. Shen, Y. Yue, D. J. McClements, and Y. Park. 2016. Delivery of dietary triglycerides to Caenorhabditis elegans using lipid nanoparticles: nanoemulsion-based delivery systems. Food Chemistry 202:451–7. doi: 10.1016/j.foodchem.2016.02.022.
- Coolon, J. D., K. L. Jones, T. C. Todd, B. C. Carr, and M. A. Herman. 2009. Caenorhabditis elegans genomic response to soil bacteria predicts environment-specific genetic effects on life history traits. PLoS Genetics 5 (6):e1000503. doi: 10.1371/journal.pgen.1000503.
- Cypser, J. R., D. Kitzenberg, and S.-K. Park. 2013. Dietary restriction in C. elegans: recent advances. Experimental Gerontology 48 (10):1014–7. doi: 10.1016/j.exger.2013.02.018.
- Darling, N. J, and S. J. Cook. 2014. The role of MAPK signalling pathways in the response to endoplasmic reticulum stress. Biochimica et Biophysica Acta 1843 (10):2150–63. doi: 10.1016/j.bbamcr.2014.01.009.
- Day, E. A., R. J. Ford, and G. R. Steinberg. 2017. AMPK as a therapeutic target for treating metabolic diseases. Trends in Endocrinology and Metabolism: TEM 28 (8):545–60. doi: 10.1016/j.tem.2017.05.004.
- Deline, M., J. Keller, M. Rothe, W.-H. Schunck, R. Menzel, and J. L. Watts. 2015. Epoxides derived from dietary dihomo-gamma-linolenic acid induce germ cell death in C. elegans. Scientific Reports 5:15417. doi: 10.1038/srep15417.
- Depuydt, G., F. Xie, V. A. Petyuk, A. Smolders, H. M. Brewer, D. G. Camp, IIR. D. Smith, and B. P. Braeckman. 2014. LC-MS proteomics analysis of the insulin/IGF-1-deficient Caenorhabditis elegans daf-2(e1370) mutant reveals extensive restructuring of intermediary metabolism. Journal of Proteome Research 13 (4):1938–56. doi: 10.1021/pr401081b.
- Dirksen, P., S. A. Marsh, I. Braker, N. Heitland, S. Wagner, R. Nakad, S. Mader, C. Petersen, V. Kowallik, P. Rosenstiel, et al. 2016. The native microbiome of the nematode Caenorhabditis elegans: gateway to a new host-microbiome model. BMC Biology 14:38. doi: 10.1186/s12915-016-0258-1.
- Dirksen, P., A. Assié, J. Zimmermann, F. Zhang, A.-M. Tietje, S. A. Marsh, M. A. Félix, M. Shapira, C. Kaleta, H. Schulenburg, et al. 2020. CeMbio - the Caenorhabditis elegans microbiome resource. G3 (Bethesda, Md.) 10 (9):3025–39. doi: 10.1534/g3.120.401309.
- Doh, J. H., A. B. Moore, İ. Çelen, M. T. Moore, and C. R. Sabanayagam. 2016. ChIP and Chips: introducing the WormPharm for correlative studies employing pharmacology and genome-wide analyses in C. elegans. Journal of Biological Methods 3 (2):e44. doi: 10.14440/jbm.2016.109.
- Edwards, C., J. Canfield, N. Copes, A. Brito, M. Rehan, D. Lipps, J. Brunquell, S. D. Westerheide, and P. C. Bradshaw. 2015. Mechanisms of amino acid-mediated lifespan extension in Caenorhabditis elegans. BMC Genetics 16 (1):8. doi: 10.1186/s12863-015-0167-2.
- Ewald, C. Y., J. I. Castillo-Quan, and T. K. Blackwell. 2018. Untangling longevity, dauer, and healthspan in Caenorhabditis elegans insulin/IGF-1-signalling. Gerontology 64 (1):96–104. doi: 10.1159/000480504.
- Efeyan, A., W. C. Comb, and D. M. Sabatini. 2015. Nutrient-sensing mechanisms and pathways. Nature 517 (7534):302–10. doi: 10.1038/nature14190.
- Estevez, A. O., K. L. Morgan, N. J. Szewczyk, D. Gems, and M. Estevez. 2014. The neurodegenerative effects of selenium are inhibited by FOXO and PINK1/PTEN regulation of insulin/insulin-like growth factor signaling in Caenorhabditis elegans. Neurotoxicology 41 (100):28–43. doi: 10.1016/j.neuro.2013.12.012.
- Fang, Z., Y. Chen, G. Wang, T. Feng, M. Shen, B. Xiao, J. Gu, W. Wang, J. Li, and Y. Zhang. 2019. Evaluation of the antioxidant effects of acid hydrolysates from Auricularia auricular polysaccharides using a Caenorhabditis elegans model. Food & Function 10 (9):5531–43. doi: 10.1039/C8FO02589D.
- Félix, M.-A, and F. Duveau. 2012. Population dynamics and habitat sharing of natural populations of Caenorhabditis elegans and C. briggsae. BMC Biology 10:59. doi: 10.1186/1741-7007-10-59.
- Flavel, M. R., A. Mechler, M. Shahmiri, E. R. Mathews, A. E. Franks, W. Chen, D. Zanker, B. Xian, S. Gao, J. Luo, et al. 2018. Growth of Caenorhabditis elegans in defined media is dependent on presence of particulate matter. G3 (Bethesda, Md.) 8 (2):567–75. doi: 10.1534/g3.117.300325.
- Fontana, L, and L. Partridge. 2015. Promoting health and longevity through diet: from model organisms to humans. Cell 161 (1):106–18. doi: 10.1016/j.cell.2015.02.020.
- Forsythe, M. E., D. C. Love, B. D. Lazarus, E. J. Kim, W. A. Prinz, G. Ashwell, M. W. Krause, and J. A. Hanover. 2006. Caenorhabditis elegans ortholog of a diabetes susceptibility locus: Oga-1 (O-GlcNAcase) knockout impacts O-GlcNAc cycling, metabolism, and dauer. Proceedings of the National Academy of Sciences of the United States of America 103 (32):11952–7. doi: 10.1073/pnas.0601931103.
- Franco-Juárez, B., F. Mejía-Martínez, E. Moreno-Arriola, A. Hernández-Vázquez, S. Gómez-Manzo, J. Marcial-Quino, R. Arreguín-Espinosa, A. Velázquez-Arellano, and D. Ortega-Cuellar. 2018. A high glucose diet induces autophagy in a HLH-30/TFEB-dependent manner and impairs the normal lifespan of C. elegans. Aging 10 (10):2657–67. doi: 10.18632/aging.101577.
- Franks, C. J., L. Holden-Dye, K. Bull, S. Luedtke, and R. J. Walker. 2006. Anatomy, physiology and pharmacology of Caenorhabditis elegans pharynx: a model to define gene function in a simple neural system. Invertebrate Neuroscience: IN 6 (3):105–22. doi: 10.1007/s10158-006-0023-1.
- Frézal, L, and M.-A. Félix. 2015. The natural history of model organisms: C. elegans outside the Petri dish. eLife 4:e05849. doi: 10.7554/eLife.05849.
- Garcia, A. M., M. L. Ladage, D. R. Dumesnil, K. Zaman, V. Shulaev, R. K. Azad, and P. A. Padilla. 2015. Glucose induces sensitivity to oxygen deprivation and modulates insulin/IGF-1 signaling and lipid biosynthesis in Caenorhabditis elegans. Genetics 200 (1):167–84. doi: 10.1534/genetics.115.174631.
- Garsin, D. A., J. M. Villanueva, J. Begun, D. H. Kim, C. D. Sifri, S. B. Calderwood, G. Ruvkun, and F. M. Ausubel. 2003. Long-lived C. elegans daf-2 mutants are resistant to bacterial pathogens. Science 300 (5627):1921. doi: 10.1126/science.1080147.
- GBD 2017 Diet Collaborators. 2019. Health effects of dietary risks in 195 countries, 1990-2017: a systematic analysis for the Global Burden of Disease Study 2017. Lancet 393 (10184):1958–72. doi: 10.1016/S0140-6736(19)30041-8.
- Gentile, C. L, and T. L. Weir. 2018. The gut microbiota at the intersection of diet and human health. Science 362 (6416):776–80. doi: 10.1126/science.aau5812.
- Gerbaba, T. K., L. Green-Harrison, and A. G. Buret. 2017. Modeling host-microbiome interactions in Caenorhabditis elegans. Journal of Nematology 49 (4):348–56. doi: 10.21307/jofnem-2017-082.
- Ghosh, D. D., D. Lee, X. Jin, H. R. Horvitz, and M. N. Nitabach. 2021. C. elegans discriminates colors to guide foraging. Science 371 (6533):1059–63. doi: 10.1126/science.abd3010.
- Giese, G. E., M. D. Walker, O. Ponomarova, H. Zhang, X. Li, G. Minevich, and A. J. Walhout. 2020. Caenorhabditis elegans methionine/S-adenosylmethionine cycle activity is sensed and adjusted by a nuclear hormone receptor. eLife 9:e60259. doi: 10.7554/eLife.60259.
- Gómez-Orte, E., E. Cornes, A. Zheleva, B. Sáenz-Narciso, M. de Toro, M. Iñiguez, R. López, J.-F. San-Juan, B. Ezcurra, B. Sacristán, et al. 2018. Effect of the diet type and temperature on the C. elegans transcriptome. Oncotarget 9 (11):9556–71. doi: 10.18632/oncotarget.23563.
- Gomez-Amaro, R. L., E. R. Valentine, M. Carretero, S. E. LeBoeuf, S. Rangaraju, C. D. Broaddus, G. M. Solis, J. R. Williamson, and M. Petrascheck. 2015. Measuring food intake and nutrient absorption in Caenorhabditis elegans. Genetics 200 (2):443–54. doi: 10.1534/genetics.115.175851.
- Gottschling, D.-C, and F. Döring. 2019. Is C. elegans a suitable model for nutritional science? Genes & Nutrition 14:1. doi: 10.1186/s12263-018-0625-3.
- Gourgou, E, and N. Chronis. 2016. Chemically induced oxidative stress affects ASH neuronal function and behavior in C. elegans. Scientific Reports 6:38147. doi: 10.1038/srep38147.
- Greene, J. S., M. Brown, M. Dobosiewicz, I. G. Ishida, E. Z. Macosko, X. Zhang, R. A. Butcher, D. J. Cline, P. T. McGrath, and C. I. Bargmann. 2016. Balancing selection shapes density-dependent foraging behaviour. Nature 539 (7628):254–8. doi: 10.1038/nature19848.
- Guo, K., L. Su, Y. Wang, H. Liu, J. Lin, P. Cheng, X. Yin, M. Liang, Q. Wang, and Z. Huang. 2020. Antioxidant and anti-aging effects of a sea cucumber protein hydrolyzate and bioinformatic characterization of its composing peptides. Food & Function 11 (6):5004–16. doi: 10.1039/d0fo00560f.
- Gusarov, I., L. Gautier, O. Smolentseva, I. Shamovsky, S. Eremina, A. Mironov, and E. Nudler. 2013. Bacterial nitric oxide extends the lifespan of C. elegans. Cell 152 (4):818–30. doi: 10.1016/j.cell.2012.12.043.
- Gusarov, I., B. Pani, L. Gautier, O. Smolentseva, S. Eremina, I. Shamovsky, O. Katkova-Zhukotskaya, A. Mironov, and E. Nudler. 2017. Glycogen controls Caenorhabditis elegans lifespan and resistance to oxidative stress. Nature Communications 8:15868. doi: 10.1038/ncomms15868.
- Hahm, J.-H., S. Kim, R. DiLoreto, C. Shi, S.-J V. Lee, C. T. Murphy, and H. G. Nam. 2015. C. elegans maximum velocity correlates with healthspan and is maintained in worms with an insulin receptor mutation. Nature Communications 6 (1):8919. doi: 10.1038/ncomms9919.
- Hahn, O., L. F. Drews, A. Nguyen, T. Tatsuta, L. Gkioni, O. Hendrich, Q. Zhang, T. Langer, S. Pletcher, M. J. O. Wakelam, et al. 2019. A nutritional memory effect counteracts benefits of dietary restriction in old mice. Nature Metabolism 1 (11):1059–73. doi: 10.1038/s42255-019-0121-0.
- Han, S., E. A. Schroeder, C. G. Silva-García, K. Hebestreit, W. B. Mair, and A. Brunet. 2017. Mono-unsaturated fatty acids link H3K4me3 modifiers to C. elegans lifespan. Nature 544 (7649):185–90. doi: 10.1038/nature21686.
- Hanover, J. A., M. E. Forsythe, P. T. Hennessey, T. M. Brodigan, D. C. Love, G. Ashwell, and M. Krause. 2005. A Caenorhabditis elegans model of insulin resistance: altered macronutrient storage and dauer formation in an OGT-1 knockout. Proceedings of the National Academy of Sciences of the United States of America 102 (32):11266–71. doi: 10.1073/pnas.0408771102.
- Heger, P., M. Kroiher, N. Ndifon, and E. Schierenberg. 2010. Conservation of MAP kinase activity and MSP genes in parthenogenetic nematodes. BMC Developmental Biology 10:51. doi: 10.1186/1471-213X-10-51.
- Hernández-Saavedra, D., L. Moody, G. B. Xu, H. Chen, and Y.-X. Pan. 2019. Epigenetic regulation of metabolism and inflammation by calorie restriction. Advances in Nutrition (Bethesda, Md.) 10 (3):520–36. doi: 10.1093/advances/nmy129.
- Herzig, S, and R. J. Shaw. 2018. AMPK: guardian of metabolism and mitochondrial homeostasis. Nature Reviews. Molecular Cell Biology 19 (2):121–35. doi: 10.1038/nrm.2017.95.
- Hibshman, J. D., A. E. Doan, B. T. Moore, R. E. Kaplan, A. Hung, A. K. Webster, D. P. Bhatt, R. Chitrakar, M. D. Hirschey, and L. R. Baugh. 2017. daf-16/FoxO promotes gluconeogenesis and trehalose synthesis during starvation to support survival. eLife 6:e30057. doi: 10.7554/eLife.30057.
- Hibshman, J. D., A. K. Webster, and L. R. Baugh. 2021. Liquid-culture protocols for synchronous starvation, growth, dauer formation, and dietary restriction of Caenorhabditis elegans. STAR Protocols 2 (1):100276. doi: 10.1016/j.xpro.2020.100276.
- Hirotsu, T, and Y. Iino. 2005. Neural circuit-dependent odor adaptation in C. elegans is regulated by the Ras-MAPK pathway. Genes to Cells: Devoted to Molecular & Cellular Mechanisms 10 (6):517–30. doi: 10.1111/j.1365-2443.2005.00856.x.
- Honda, Y., M. Tanaka, and S. Honda. 2010. Trehalose extends longevity in the nematode Caenorhabditis elegans. Aging Cell 9 (4):558–69. doi: 10.1111/j.1474-9726.2010.00582.x.
- Houthoofd, K., B. P. Braeckman, I. Lenaerts, K. Brys, A. De Vreese, S. Van Eygen, and J. R. Vanfleteren. 2002. Axenic growth up-regulates mass-specific metabolic rate, stress resistance, and extends life span in Caenorhabditis elegans. Experimental Gerontology 37 (12):1371–8. doi: 10.1016/S0531-5565(02)00173-0.
- Huang, Z, and A. Tunnacliffe. 2004. Response of human cells to desiccation: comparison with hyperosmotic stress response. The Journal of Physiology 558 (Pt 1):181–91. doi: 10.1113/jphysiol.2004.065540.
- Huang, Z., M. C. Banton, and A. Tunnacliffe. 2010. Modeling anhydrobiosis: activation of the mitogen-activated protein kinase ERK by dehydration in both human cells and nematodes. Journal of Experimental Zoology. Part A, Ecological Genetics and Physiology 313 (10):660–70. doi: 10.1002/jez.637.
- Huang, Z., L. Ma, A. Mishra, J. E. Turnbull, and H. Tu. 2022. C. elegans as an emerging model of pharmacological innovation. Frontiers in Pharmacology 13:1029752. doi: 10.3389/fphar.2022.1029752.
- Huang, Z., Y. Xiao, and Y. Wang. 2022. A C. elegans microparticle food and its preparation method. Chinese Patent Application No. 202210385449.9, filed April 13, 2022.
- Hunt, P. R., N. Olejnik, K. D. Bailey, C. A. Vaught, and R. L. Sprando. 2018. C. elegans development and activity test detects mammalian developmental neurotoxins. Food and Chemical Toxicology: An International Journal Published for the British Industrial Biological Research Association 121:583–92. doi: 10.1016/j.fct.2018.09.061.
- Ishita, Y., T. Chihara, and M. Okumura. 2020. Serotonergic modulation of feeding behavior in Caenorhabditis elegans and other related nematodes. Neuroscience Research 154:9–19. doi: 10.1016/j.neures.2019.04.006.
- Jenkins, N. L., S. A. James, A. Salim, F. Sumardy, T. P. Speed, M. Conrad, D. R. Richardson, A. I. Bush, and G. McColl. 2020. Changes in ferrous iron and glutathione promote ferroptosis and frailty in aging Caenorhabditis elegans. eLife 9:e56580. doi: 10.7554/eLife.56580.
- Jia, W., Q. Peng, L. Su, X. Yu, C. W. Ma, M. Liang, X. Yin, Y. Zou, and Z. Huang. 2018. Novel bioactive peptides from Meretrix meretrix protect Caenorhabditis elegans against free radical-induced oxidative stress through the stress response factor DAF-16/FOXO. Marine Drugs 16 (11):444. doi: 10.3390/md16110444.
- Jiménez-Chillarón, J. C., R. Díaz, D. Martínez, T. Pentinat, M. Ramón-Krauel, S. Ribó, and T. Plösch. 2012. The role of nutrition on epigenetic modifications and their implications on health. Biochimie 94 (11):2242–63. doi: 10.1016/j.biochi.2012.06.012.
- Jobson, M. A., J. M. Jordan, M. A. Sandrof, J. D. Hibshman, A. L. Lennox, and L. R. Baugh. 2015. Transgenerational effects of early life starvation on growth, reproduction, and stress resistance in Caenorhabditis elegans. Genetics 201 (1):201–12. doi: 10.1534/genetics.115.178699.
- Jordan, J. M., J. D. Hibshman, A. K. Webster, R. E. W. Kaplan, A. Leinroth, R. Guzman, C. S. Maxwell, R. Chitrakar, E. A. Bowman, A. L. Fry, et al. 2019. Insulin/IGF signaling and vitellogenin provisioning mediate intergenerational adaptation to nutrient stress. Current Biology: CB 29 (14):2380–8.e5. doi: 10.1016/j.cub.2019.05.062.
- Jung, Y., S. Kwon, S. Ham, D. Lee, H.-E H. Park, Y. Yamaoka, D.-E. Jeong, M. Artan, O. Altintas, S. Park, et al. 2020. Caenorhabditis elegans Lipin 1 moderates the lifespan-shortening effects of dietary glucose by maintaining ω-6 polyunsaturated fatty acids. Aging Cell 19 (6):e13150. doi: 10.1111/acel.13150.
- Kahn, B. B., T. Alquier, D. Carling, and D. G. Hardie. 2005. AMP-activated protein kinase: ancient energy gauge provides clues to modern understanding of metabolism. Cell Metabolism 1 (1):15–25. doi: 10.1016/j.cmet.2004.12.003.
- Kaletta, T, and M. O. Hengartner. 2006. Finding function in novel targets: C. elegans as a model organism. Nature Reviews. Drug Discovery 5 (5):387–98. doi: 10.1038/nrd2031.
- Kapahi, P., M. Kaeberlein, and M. Hansen. 2017. Dietary restriction and lifespan: lessons from invertebrate models. Ageing Research Reviews 39:3–14. doi: 10.1016/j.arr.2016.12.005.
- Ke, W., J. N. Reed, C. Yang, N. Higgason, L. Rayyan, C. Wählby, A. E. Carpenter, M. Civelek, and E. J. O’Rourke. 2021. Genes in human obesity loci are causal obesity genes in C. elegans. PLoS Genetics 17 (9):e1009736. doi: 10.1371/journal.pgen.1009736.
- Keith, S. A., F. R. G. Amrit, R. Ratnappanand, and A. Ghazi. 2014. The C. elegans healthspan and stress-resistance assay toolkit. Methods (San Diego, Calif.) 68 (3):476–86. doi: 10.1016/j.ymeth.2014.04.003.
- Kim, J, and K.-L. Guan. 2019. mTOR as a central hub of nutrient signalling and cell growth. Nature Cell Biology 21 (1):63–71. doi: 10.1038/s41556-018-0205-1.
- Köhnlein, K., N. Urban, D. Guerrero-Gómez, H. Steinbrenner, P. Urbánek, J. Priebs, P. Koch, C. Kaether, A. Miranda-Vizuete, and L. O. Klotz. 2020. A Caenorhabditis elegans ortholog of human selenium-binding protein 1 is a pro-aging factor protecting against selenite toxicity. Redox Biology 28:101323. doi: 10.1016/j.redox.2019.101323.
- Koopman, M., Q. Peter, R. I. Seinstra, M. Perni, M. Vendruscolo, C. M. Dobson, T. P. J. Knowles, and E. A. A. Nollen. 2020. Assessing motor-related phenotypes of Caenorhabditis elegans with the wide field-of-view nematode tracking platform. Nature Protocols 15 (6):2071–106. doi: 10.1038/s41596-020-0321-9.
- Korta, J., D. A. Clark, C. V. Gabel, L. Mahadevan, and A. D. T. Samuel. 2007. Mechanosensation and mechanical load modulate the locomotory gait of swimming C. elegans. The Journal of Experimental Biology 210 (Pt 13):2383–9. doi: 10.1242/jeb.004572.
- Koseki, K., Y. Maekawa, T. Bito, Y. Yabuta, and F. Watanabe. 2020. High-dose folic acid supplementation results in significant accumulation of unmetabolized homocysteine, leading to severe oxidative stress in Caenorhabditis elegans. Redox Biology 37:101724. doi: 10.1016/j.redox.2020.101724.
- Kumar, J., T. Barhydt, A. Awasthi, G. J. Lithgow, D. W. Killilea, and P. Kapahi. 2016. Zinc levels modulate lifespan through multiple longevity pathways in Caenorhabditis elegans. PLoS One 11 (4):e0153513. doi: 10.1371/journal.pone.0153513.
- Lackner, M. R., K. Kornfeld, L. M. Miller, H. R. Horvitz, and S. K. Kim. 1994. A MAP kinase homolog, mpk-1, is involved in ras-mediated induction of vulval cell fates in Caenorhabditis elegans. Genes & Development 8 (2):160–73. doi: 10.1101/gad.8.2.160.
- Lakowski, B, and S. Hekimi. 1998. The genetics of caloric restriction in Caenorhabditis elegans. Proceedings of the National Academy of Sciences of the United States of America 95 (22):13091–6. doi: 10.1073/pnas.95.22.13091.
- Lee, S.-J., C. T. Murphy, and C. Kenyon. 2009. Glucose shortens the life span of C. elegans by downregulating DAF-16/FOXO activity and aquaporin gene expression. Cell Metabolism 10 (5):379–91. doi: 10.1016/j.cmet.2009.10.003.
- Lee, K. S., S. Iwanir, R. B. Kopito, M. Scholz, J. A. Calarco, D. Biron, and E. Levine. 2017. Serotonin-dependent kinetics of feeding bursts underlie a graded response to food availability in C. elegans. Nature Communications 8:14221. doi: 10.1038/ncomms14221.
- Lee, M. B., C. M. Hill, A. Bitto, and M. Kaeberlein. 2021. Antiaging diets: separating fact from fiction. Science 374 (6570):593 (eabe7365). doi: 10.1126/science.abe7365.
- Leiteritz, A., T. Schmiedl, S. Baumanns, and U. Wenzel. 2021. Amyloid-beta induced paralysis is reduced by cholecalciferol through inhibition of the steroid-signaling pathway in an Alzheimer model of Caenorhabditis elegans. Nutritional Neuroscience 24 (2):82–9. doi: 10.1080/1028415X.2019.1596371.
- Lemieux, G. A., K. A. Cunningham, L. Lin, F. Mayer, Z. Werb, and K. Ashrafi. 2015. Kynurenic acid is a nutritional cue that enables behavioral plasticity. Cell 160 (1–2):119–31. doi: 10.1016/j.cell.2014.12.028.
- Lenaerts, I., G. A. Walker, L. Van Hoorebeke, D. Gems, and J. R. Vanfleteren. 2008. Dietary restriction of Caenorhabditis elegans by axenic culture reflects nutritional requirement for constituents provided by metabolically active microbes. The Journals of Gerontology. Series A, Biological Sciences and Medical Sciences 63 (3):242–52. doi: 10.1093/gerona/63.3.242.
- Lepre, B., K. J. Mansfield, S. Ray, and E. J. Beck. 2021. Nutrition competencies for medicine: an integrative review and critical synthesis. BMJ Open 11 (3):e043066. doi: 10.1136/bmjopen-2020-043066.
- Leulier, F., L. T. MacNeil, W.-J. Lee, J. F. Rawls, P. D. Cani, M. Schwarzer, L. Zhao, and S. J. Simpson. 2017. Integrative physiology: at the crossroads of nutrition, microbiota, animal physiology, and human health. Cell Metabolism 25 (3):522–34. doi: 10.1016/j.cmet.2017.02.001.
- Lev, I., R. Bril, Y. Liu, L. I. Ceré, and O. Rechavi. 2019. Inter-generational consequences for growing Caenorhabditis elegans in liquid. Philosophical Transactions of the Royal Society of London. Series B, Biological Sciences 374 (1770):20180125. doi: 10.1098/rstb.2018.0125.
- Li, H., J. Xu, Y. Liu, S. Ai, F. Qin, Z. Li, H. Zhang, and Z. Huang. 2011. Antioxidant and moisture-retention activities of the polysaccharide from Nostoc commune. Carbohydrate Polymers 83 (4):1821–7. doi: 10.1016/j.carbpol.2010.10.046.
- Li, H., R. Shi, F. Ding, H. Wang, W. Han, F. Ma, M. Hu, C. W. Ma, and Z. Huang. 2016. Astragalus polysaccharide suppresses 6-hydroxydopamine-induced neurotoxicity in Caenorhabditis elegans. Oxidative Medicine and Cellular Longevity 2016:4856761. doi: 10.1155/2016/4856761.
- Li, H., F. Ding, L. Xiao, R. Shi, H. Wang, W. Han, and Z. Huang. 2017. Food-derived antioxidant polysaccharides and their pharmacological potential in neurodegenerative diseases. Nutrients 9 (7):778. doi: 10.3390/nu9070778.
- Li, L., Y. Chen, C. Chenzhao, S. Fu, Q. Xu, and J. Zhao. 2018. Glucose negatively affects Nrf2/SKN-1-mediated innate immunity in C. elegans. Aging 10 (11):3089–103. doi: 10.18632/aging.101610.
- Li, T., X.-W. Shi, Y.-M. Du, and Y.-F. Tang. 2007. Quaternized chitosan/alginate nanoparticles for protein delivery. Journal of Biomedical Materials Research. Part A 83 (2):383–90. doi: 10.1002/jbm.a.31322.
- Li, W.-H., C.-H. Chang, C.-W. Huang, C.-C. Wei, and V. H.-C. Liao. 2014. Selenite enhances immune response against Pseudomonas aeruginosa PA14 via SKN-1 in Caenorhabditis elegans. PLoS One 9 (8):e105810. doi: 10.1371/journal.pone.0105810.
- Liggett, M. R., M. J. Hoy, M. Mastroianni, and M. A. Mondoux. 2015. High-glucose diets have sex-specific effects on aging in C. elegans: toxic to hermaphrodites but beneficial to males. Aging 7 (6):383–8. doi: 10.18632/aging.100759.
- Lin, C., Y. Lin, T. Meng, J. Lian, Y. Liang, Y. Kuang, Y. Cao, and Y. Chen. 2020. Anti-fat effect and mechanism of polysaccharide-enriched extract from Cyclocarya paliurus (Batal.) Iljinskaja in Caenorhabditis elegans. Food & Function 11 (6):5320–32. doi: 10.1039/c9fo03058a.
- Liu, Q., P. B. Kidd, M. Dobosiewicz, and C. I. Bargmann. 2018. C. elegans AWA olfactory neurons fire calcium-mediated all-or-none action potentials. Cell 175 (1):57–70.e17. doi: 10.1016/j.cell.2018.08.018.
- Liu, Y. J., G. E. Janssens, R. L. McIntyre, M. Molenaars, R. Kamble, A. W. Gao, A. Jongejan, M. v Weeghel, A. W. MacInnes, and R. H. Houtkooper. 2019. Glycine promotes longevity in Caenorhabditis elegans in a methionine cycle-dependent fashion. PLoS Genetics 15 (3):e1007633. doi: 10.1371/journal.pgen.1007633.
- Lodha, D., S. Rajasekaran, T. Jayavelu, and J. R. Subramaniam. 2022. Detrimental effects of fructose on mitochondria in mouse motor neurons and on C. elegans healthspan. Nutritional Neuroscience 25 (6):1277–86. doi: 10.1080/1028415X.2020.1853413.
- López, M., R. Nogueiras, M. Tena-Sempere, and C. Diéguez. 2016. Hypothalamic AMPK: a canonical regulator of whole-body energy balance. Nature Reviews. Endocrinology 12 (7):421–32. doi: 10.1038/nrendo.2016.67.
- López-Otín, C., M. A. Blasco, L. Partridge, M. Serrano, and G. Kroemer. 2013. The hallmarks of aging. Cell 153 (6):1194–217. doi: 10.1016/j.cell.2013.05.039.
- Lu, N. C, and K. M. Goetsch. 1993. Carbohydrate requirement of Caenorhabditis elegans and the final development of a chemically-defined medium. Nematologica 39 (1-4):303–11. doi: 10.1163/187529293X00259.
- Lu, M., A. Mishra, C. Boschetti, J. Lin, Y. Liu, H. Huang, C. F. Kaminski, Z. Huang, A. Tunnacliffe, and G. S. Kaminski Schierle. 2021. Sea cucumber-derived peptides alleviate oxidative stress in neuroblastoma cells and improve survival in C. elegans exposed to neurotoxic paraquat. Oxidative Medicine and Cellular Longevity 2021:8842926. doi: 10.1155/2021/8842926.
- Lublin, A. L, and C. D. Link. 2013. Alzheimer’s disease drug discovery: in vivo screening using Caenorhabditis elegans as a model for β-amyloid peptide-induced toxicity. Drug Discovery Today. Technologies 10 (1):e115–e119. doi: 10.1016/j.ddtec.2012.02.002.
- Lucio, D., M. C. Martínez-Ohárriz, G. Jaras, P. Aranaz, C. J. González-Navarro, A. Radulescu, and J. M. Irache. 2017. Optimization and evaluation of zein nanoparticles to improve the oral delivery of glibenclamide. In vivo study using C. elegans. European Journal of Pharmaceutics and Biopharmaceutics: Official Journal of Arbeitsgemeinschaft Fur Pharmazeutische Verfahrenstechnik e.V 121:104–12. doi: 10.1016/j.ejpb.2017.09.018.
- Lucio, D., M. C. Martínez-Ohárriz, C. J. González-Navarro, D. Navarro-Herrera, G. González-Gaitano, A. Radulescu, and J. M. Irache. 2018. Coencapsulation of cyclodextrins into poly(anhydride) nanoparticles to improve the oral administration of glibenclamide. A screening on C. elegans. Colloids and Surfaces. B, Biointerfaces 163:64–72. doi: 10.1016/j.colsurfb.2017.12.038.
- Lynn, D. A., H. M. Dalton, J. N. Sowa, M. C. Wang, A. A. Soukas, and S. P. Curran. 2015. Omega-3 and -6 fatty acids allocate somatic and germline lipids to ensure fitness during nutrient and oxidative stress in Caenorhabditis elegans. Proceedings of the National Academy of Sciences of the United States of America 112 (50):15378–83. doi: 10.1073/pnas.1514012112.
- Ma, H., K. A. Lenz, X. Gao, S. Li, and L. K. Wallis. 2019. Comparative toxicity of a food additive TiO2, a bulk TiO2, and a nano-sized P25 to a model organism the nematode C. elegans. Environmental Science and Pollution Research International 26 (4):3556–68. doi: 10.1007/s11356-018-3810-4.
- MacNeil, L. T., E. Watson, H. E. Arda, L. J. Zhu, and A. J. M. Walhout. 2013. Diet-induced developmental acceleration independent of TOR and insulin in C. elegans. Cell 153 (1):240–52. doi: 10.1016/j.cell.2013.02.049.
- Mark, K. A., K. J. Dumas, D. Bhaumik, B. Schilling, S. Davis, T. R. Oron, D. J. Sorensen, M. Lucanic, R. B. Brem, S. Melov, et al. 2016. Vitamin D promotes protein homeostasis and longevity via the stress response pathway genes SKN-1, IRE-1, and XBP-1. Cell Reports 17 (5):1227–37. doi: 10.1016/j.celrep.2016.09.086.
- Martin, F.-P J., B. Spanier, S. Collino, I. Montoliu, C. Kolmeder, P. Giesbertz, M. Affolter, M. Kussmann, H. Daniel, S. Kochhar, et al. 2011. Metabotyping of Caenorhabditis elegans and their culture media revealed unique metabolic phenotypes associated to amino acid deficiency and insulin-like signaling. Journal of Proteome Research 10 (3):990–1003. doi: 10.1021/pr100703a.
- Mautz, B. S., M. I. Lind, and A. A. Maklakov. 2020. Dietary restriction improves fitness of aging parents but reduces fitness of their offspring in nematodes. The Journals of Gerontology. Series A, Biological Sciences and Medical Sciences 75 (5):843–8. doi: 10.1093/gerona/glz276.
- Mendoza, A. D., T. K. Woodruff, S. M. Wignall, and T. V. O’Halloran. 2017. Zinc availability during germline development impacts embryo viability in Caenorhabditis elegans. Comparative Biochemistry and Physiology. Toxicology & Pharmacology: CBP 191:194–202. doi: 10.1016/j.cbpc.2016.09.007.
- Metzler, R., E. A. Meleshkevitch, J. Fox, H. Kim, and D. Y. Boudko. 2013. An SLC6 transporter of the novel B0,- system aids in absorption and detection of nutrient amino acids in Caenorhabditis elegans. The Journal of Experimental Biology 216 (Pt 15):2843–57. doi: 10.1242/jeb.081497.
- Miedel, M. T., X. Zeng, N. A. Yates, G. A. Silverman, and C. J. Luke. 2014. Isolation of serpin-interacting proteins in C. elegans using protein affinity purification. Methods (San Diego, Calif.) 68 (3):536–41. doi: 10.1016/j.ymeth.2014.04.019.
- Möller, S., N. Saul, A. A. Cohen, R. Köhling, S. Sender, H. M. Escobar, C. Junghanss, F. Cirulli, A. Berry, P. Antal, et al. 2020. Healthspan pathway maps in C. elegans and humans highlight transcription, proliferation/biosynthesis and lipids. Aging 12 (13):12534–81. doi: 10.18632/aging.103514.
- Momma, K., T. Homma, R. Isaka, S. Sudevan, and A. Higashitani. 2017. Heat-induced calcium leakage causes mitochondrial damage in Caenorhabditis elegans body-wall muscles. Genetics 206 (4):1985–94. doi: 10.1534/genetics.117.202747.
- Mukhopadhyay, S., Y. Lu, S. Shaham, and P. Sengupta. 2008. Sensory signaling-dependent remodeling of olfactory cilia architecture in C. elegans. Developmental Cell 14 (5):762–74. doi: 10.1016/j.devcel.2008.03.002.
- Na, H., O. Ponomarova, G. E. Giese, and A. J. M. Walhout. 2018. C. elegans MRP-5 exports Vitamin B12 from mother to offspring to support embryonic development. Cell Reports 22 (12):3126–33. doi: 10.1016/j.celrep.2018.02.100.
- Nikolic, I., M. Leiva, and G. Sabio. 2020. The role of stress kinases in metabolic disease. Nature Reviews. Endocrinology 16 (12):697–716. doi: 10.1038/s41574-020-00418-5.
- O’Donnell, M. P., B. W. Fox, P.-H. Chao, F. C. Schroeder, and P. Sengupta. 2020. A neurotransmitter produced by gut bacteria modulates host sensory behaviour. Nature 583 (7816):415–20. doi: 10.1038/s41586-020-2395-5.
- Offenburger, S.-L., E. Jongsma, and A. Gartner. 2018. Mutations in Caenorhabditis elegans neuroligin-like glit-1, the apoptosis pathway and the calcium chaperone crt-1 increase dopaminergic neurodegeneration after 6-OHDA treatment. PLoS Genetics 14 (1):e1007106. doi: 10.1371/journal.pgen.1007106.
- Olshansky, S. J. 2018. From lifespan to healthspan. Journal of the American Medical Association 320 (13):E1–E2. doi: 10.1001/jama.2018.12621.
- O’Rourke, E. J., P. Kuballa, R. Xavier, and G. Ruvkun. 2013. ω-6 polyunsaturated fatty acids extend life span through the activation of autophagy. Genes & Development 27 (4):429–40. doi: 10.1101/gad.205294.112.
- Ortiz de Ora, L, and E. N. Bess. 2021. Emergence of Caenorhabditis elegans as a model organism for dissecting the gut–brain axis. mSystems 6 (4):e00755–21. doi: 10.1128/mSystems.00755-21.
- Pallauf, K., J. K. Bendall, C. Scheiermann, K. Watschinger, J. Hoffmann, T. Roeder, and G. Rimbach. 2013. Vitamin C and lifespan in model organisms. Food and Chemical Toxicology: An International Journal Published for the British Industrial Biological Research Association 58:255–63. doi: 10.1016/j.fct.2013.04.046.
- Pang, S, and S. P. Curran. 2014. Adaptive capacity to bacterial diet modulates aging in C. elegans. Cell Metabolism 19 (2):221–31. doi: 10.1016/j.cmet.2013.12.005.
- Penkov, S., B. K. Raghuraman, C. Erkut, J. Oertel, R. Galli, E. J. M. Ackerman, D. Vorkel, J.-M. Verbavatz, E. Koch, K. Fahmy, et al. 2020. A metabolic switch regulates the transition between growth and diapause in C. elegans. BMC Biology 18 (1):31. doi: 10.1186/s12915-020-0760-3.
- Perez, M. F, and B. Lehner. 2019. Intergenerational and transgenerational epigenetic inheritance in animals. Nature Cell Biology 21 (2):143–51. doi: 10.1038/s41556-018-0242-9.
- Pho, K. B, and L. T. MacNeil. 2019. Biology is the root of variability: cautionary tales in Caenorhabditis elegans biology. Biochemical Society Transactions 47 (3):887–96. doi: 10.1042/BST20190001.
- Pierce-Shimomura, J. T., B. L. Chen, J. J. Mun, R. Ho, R. Sarkis, and S. L. McIntire. 2008. Genetic analysis of crawling and swimming locomotory patterns in C. elegans. Proceedings of the National Academy of Sciences of the United States of America 105 (52):20982–7. doi: 10.1073/pnas.0810359105.
- Polyak, E., J. Ostrovsky, M. Peng, S. D. Dingley, M. Tsukikawa, Y. J. Kwon, S. E. McCormack, M. Bennett, R. Xiao, C. Seiler, et al. 2018. N-acetylcysteine and vitamin E rescue animal longevity and cellular oxidative stress in pre-clinical models of mitochondrial complex I disease. Molecular Genetics and Metabolism 123 (4):449–62. doi: 10.1016/j.ymgme.2018.02.013.
- Qiao, L., S. Luo, Y. Liu, X. Li, G. Wang, and Z. Huang. 2013. Reproductive and locomotory capacities of Caenorhabditis elegans were not affected by simulated variable gravities and spaceflight during the Shenzhou-8 mission. Astrobiology 13 (7):617–25. doi: 10.1089/ast.2012.0962.
- Raabe, R. C., L. D. Mathies, A. G. Davies, and J. C. Bettinger. 2014. The omega-3 fatty acid eicosapentaenoic acid is required for normal alcohol response behaviors in C. elegans. PLoS One 9 (8):e105999. doi: 10.1371/journal.pone.0105999.
- Rajan, M., C. P. Anderson, P. M. Rindler, S. J. Romney, M. C. Ferreira Dos Santos, J. Gertz, and E. A. Leibold. 2019. NHR-14 loss of function couples intestinal iron uptake with innate immunity in C. elegans through PQM-1 signaling. eLife 8:e44674. doi: 10.7554/eLife.44674.
- Rangaraju, S., D. F. Levey, K. Nho, N. Jain, K. D. Andrews, H. Le-Niculescu, D. R. Salomon, A. J. Saykin, M. Petrascheck, and A. B. Niculescu. 2016. Mood, stress and longevity: convergence on ANK3. Molecular Psychiatry 21 (8):1037–49. doi: 10.1038/mp.2016.65.
- Rao, A. U., L. K. Carta, E. Lesuisse, and I. Hamza. 2005. Lack of heme synthesis in a free-living eukaryote. Proceedings of the National Academy of Sciences of the United States of America 102 (12):4270–5. doi: 10.1073/pnas.0500877102.
- Rashid, S., K. B. Pho, H. Mesbahi, and L. T. MacNeil. 2020. Nutrient sensing and response drive developmental progression in Caenorhabditis elegans. BioEssays: News and Reviews in Molecular, Cellular and Developmental Biology 42 (3):e1900194. doi: 10.1002/bies.201900194.
- Rathor, L., B. A. Akhoon, S. Pandey, S. Srivastava, and R. Pandey. 2015. Folic acid supplementation at lower doses increases oxidative stress resistance and longevity in Caenorhabditis elegans. Age (Dordrecht, Netherlands) 37 (6):113. doi: 10.1007/s11357-015-9850-5.
- Rechavi, O., L. Houri-Ze’evi, S. Anava, W. S. S. Goh, S. Y. Kerk, G. J. Hannon, and O. Hobert. 2014. Starvation-induced transgenerational inheritance of small RNAs in C. elegans. Cell 158 (2):277–87. doi: 10.1016/j.cell.2014.06.020.
- Revtovich, A. V., R. Lee, and N. V. Kirienko. 2019. Interplay between mitochondria and diet mediates pathogen and stress resistance in Caenorhabditis elegans. PLoS Genetics 15 (3):e1008011. doi: 10.1371/journal.pgen.1008011.
- Riedinger, C., M. Mendler, A. Schlotterer, T. Fleming, J. Okun, H.-P. Hammes, S. Herzig, and P. P. Nawroth. 2018. High-glucose toxicity is mediated by AICAR-transformylase/IMP cyclohydrolase and mitigated by AMP-activated protein kinase in Caenorhabditis elegans. The Journal of Biological Chemistry 293 (13):4845–59. doi: 10.1074/jbc.M117.805879.
- Robinson, S. M. 2018. Improving nutrition to support healthy ageing: what are the opportunities for intervention? The Proceedings of the Nutrition Society 77 (3):257–64. doi: 10.1017/S0029665117004037.
- Rodríguez-Palero, M. J., A. López-Díaz, R. Marsac, J.-E. Gomes, M. Olmedo, and M. Artal-Sanz. 2018. An automated method for the analysis of food intake behaviour in Caenorhabditis elegans. Scientific Reports 8 (1):3633. doi: 10.1038/s41598-018-21964-z.
- Rohn, I., S. Raschke, M. Aschner, S. Tuck, D. Kuehnelt, A. Kipp, T. Schwerdtle, and J. Bornhorst. 2019. Treatment of Caenorhabditis elegans with small selenium species enhances antioxidant defense systems. Molecular Nutrition & Food Research 63 (9):e1801304. doi: 10.1002/mnfr.201801304.
- Romney, S. J., B. S. Newman, C. Thacker, and E. A. Leibold. 2011. HIF-1 regulates iron homeostasis in Caenorhabditis elegans by activation and inhibition of genes involved in iron uptake and storage. PLoS Genetics 7 (12):e1002394. doi: 10.1371/journal.pgen.1002394.
- Rubio-Aliaga, I. 2012. Model organisms in molecular nutrition research. Molecular Nutrition & Food Research 56 (6):844–53. doi: 10.1002/mnfr.201100784.
- Sakamoto, T., K. Maebayashi, Y. Tsunoda, and H. Imai. 2020. Inhibition of lipid peroxidation during the reproductive period extends the lifespan of Caenorhabditis elegans. Journal of Clinical Biochemistry and Nutrition 66 (2):116–23. doi: 10.3164/jcbn.19-51.
- Sakoguchi, H., A. Yoshihara, K. Izumori, and M. Sato. 2016a. Screening of biologically active monosaccharides: growth inhibitory effects of D-allose, D-talose, and L-idose against the nematode Caenorhabditis elegans. Bioscience, Biotechnology, and Biochemistry 80 (6):1058–61. doi: 10.1080/09168451.2016.1146069.
- Sakoguchi, H., A. Yoshihara, T. Shintani, K. Okuma, K. Izumori, and M. Sato. 2016b. Growth inhibitory effect of D-arabinose against the nematode Caenorhabditis elegans: discovery of a novel bioactive monosaccharide. Bioorganic & Medicinal Chemistry Letters 26 (3):726–9. doi: 10.1016/j.bmcl.2016.01.007.
- Salim, C, and P. S. Rajini. 2017. Glucose-rich diet aggravates monocrotophos-induced dopaminergic neuronal dysfunction in Caenorhabditis elegans. Journal of Applied Toxicology: JAT 37 (6):772–80. doi: 10.1002/jat.3426.
- Samuel, B. S., H. Rowedder, C. Braendle, M.-A. Félix, and G. Ruvkun. 2016. Caenorhabditis elegans responses to bacteria from its natural habitats. Proceedings of the National Academy of Sciences of the United States of America 113 (27):E3941–9. doi: 10.1073/pnas.1607183113.
- Sánchez-Blanco, A., A. Rodríguez-Matellán, A. González-Paramás, S. González-Manzano, S. K. Kim, and F. Mollinedo. 2016. Dietary and microbiome factors determine longevity in Caenorhabditis elegans. Aging 8 (7):1513–39. doi: 10.18632/aging.101008.
- Sarasija, S, and K. R. Norman. 2015. A γ-secretase independent role for presenilin in calcium homeostasis impacts mitochondrial function and morphology in Caenorhabditis elegans. Genetics 201 (4):1453–66. doi: 10.1534/genetics.115.182808.
- Saxton, R. A, and D. M. Sabatini. 2017. mTOR signaling in growth, metabolism, and disease. Cell 168 (6):960–76. doi: 10.1016/j.cell.2017.02.004.
- Sayre, F. W., E. L. Hansen, and E. A. Yarwood. 1963. Biochemical aspects of the nutrition of Caenorhabditis briggsae. Experimental Parasitology 13:98–107. doi: 10.1016/0014-4894(63)90058-4.
- Schiavi, A., S. Maglioni, K. Palikaras, A. Shaik, F. Strappazzon, V. Brinkmann, A. Torgovnick, N. Castelein, S. De Henau, B. P. Braeckman, et al. 2015. Iron-starvation-induced mitophagy mediates lifespan extension upon mitochondrial stress in C. elegans. Current Biology: CB 25 (14):1810–22. doi: 10.1016/j.cub.2015.05.059.
- Schlotterer, A., B. Masri, M. Humpert, B. K. Krämer, H.-P. Hammes, and M. Morcos. 2021. Sulforaphane and vitamin E protect from glucotoxic neurodegeneration and lifespan reduction in C. elegans. Experimental and Clinical Endocrinology & Diabetes 129 (12):887–94. doi: 10.1055/a-1158-9248.
- Schlotterer, A., G. Kukudov, F. Bozorgmehr, H. Hutter, X. Du, D. Oikonomou, Y. Ibrahim, F. Pfisterer, N. Rabbani, P. Thornalley, et al. 2009. C. elegans as model for the study of high glucose-mediated life span reduction. Diabetes 58 (11):2450–6. doi: 10.2337/db09-0567.
- Schulenburg, H, and M.-A. Félix. 2017. The natural biotic environment of Caenorhabditis elegans. Genetics 206 (1):55–86. doi: 10.1534/genetics.116.195511.
- Schulz, T. J., K. Zarse, A. Voigt, N. Urban, M. Birringer, and M. Ristow. 2007. Glucose restriction extends Caenorhabditis elegans life span by inducing mitochondrial respiration and increasing oxidative stress. Cell Metabolism 6 (4):280–93. doi: 10.1016/j.cmet.2007.08.011.
- Scott, A. J., M. Ellisonand, and D. A. Sinclair. 2021. The economic value of targeting aging. Nature Aging 1 (7):616–23. doi: 10.1038/s43587-021-00080-0.
- Seals, D. R., J. N. Justice, and T. J. LaRocca. 2016. Physiological geroscience: targeting function to increase healthspan and achieve optimal longevity. The Journal of Physiology 594 (8):2001–24. doi: 10.1113/jphysiol.2014.282665.
- Seo, Y., S. Kingsley, G. Walker, M. A. Mondoux, and H. A. Tissenbaum. 2018. Metabolic shift from glycogen to trehalose promotes lifespan and healthspan in Caenorhabditis elegans. Proceedings of the National Academy of Sciences of the United States of America 115 (12):E2791–E2800. doi: 10.1073/pnas.1714178115.
- Shapira, M. 2017. Host-microbiota interactions in Caenorhabditis elegans and their significance. Current Opinion in Microbiology 38:142–7. doi: 10.1016/j.mib.2017.05.012.
- Shashikumar, S., H. Pradeep, S. Chinnu, P. S. Rajini, and G. K. Rajanikant. 2015. Alpha-linolenic acid suppresses dopaminergic neurodegeneration induced by 6-OHDA in C. elegans. Physiology & Behavior 151:563–9. doi: 10.1016/j.physbeh.2015.08.025.
- Shemesh, N., L. Meshnik, N. Shpigel, and A. Ben-Zvi. 2017. Dietary-induced signals that activate the gonadal longevity pathway during development regulate a proteostasis switch in Caenorhabditis elegans adulthood. Frontiers in Molecular Neuroscience 10:254. doi: 10.3389/fnmol.2017.00254.
- Shen, P., R. Zhang, D. J. McClements, and Y. Park. 2019. Nanoemulsion-based delivery systems for testing nutraceutical efficacy using Caenorhabditis elegans: demonstration of curcumin bioaccumulation and body-fat reduction. Food Research International (Ottawa, Ont.) 120:157–66. doi: 10.1016/j.foodres.2019.02.036.
- Shen, P., Y. Yue, J. Zheng, and Y. Park. 2018. Caenorhabditis elegans: a convenient in vivo model for assessing the impact of food bioactive compounds on obesity, aging, and Alzheimer’s disease. Annual Review of Food Science and Technology 9:1–22. doi: 10.1146/annurev-food-030117-012709.
- Shtonda, B. B, and L. Avery. 2006. Dietary choice behavior in Caenorhabditis elegans. The Journal of Experimental Biology 209 (Pt 1):89–102. doi: 10.1242/jeb.01955.
- Simonetta, S. H, and D. A. Golombek. 2007. An automated tracking system for Caenorhabditis elegans locomotor behavior and circadian studies application. Journal of Neuroscience Methods 161 (2):273–80. doi: 10.1016/j.jneumeth.2006.11.015.
- So, S., K. Miyahara, and Y. Ohshima. 2011. Control of body size in C. elegans dependent on food and insulin/IGF-1 signal. Genes to Cells: Devoted to Molecular & Cellular Mechanisms 16 (6):639–51. doi: 10.1111/j.1365-2443.2011.01514.x.
- Soukas, A. A., E. A. Kane, C. E. Carr, J. A. Melo, and G. Ruvkun. 2009. Rictor/TORC2 regulates fat metabolism, feeding, growth, and life span in Caenorhabditis elegans. Genes & Development 23 (4):496–511. doi: 10.1101/gad.1775409.
- Sowa, J. N., A. S. Mutlu, F. Xia, and M. C. Wang. 2015. Olfaction modulates reproductive plasticity through neuroendocrine signaling in Caenorhabditis elegans. Current Biology: CB 25 (17):2284–9. doi: 10.1016/j.cub.2015.07.023.
- Strange, K., M. Christensen, and R. Morrison. 2007. Primary culture of Caenorhabditis elegans developing embryo cells for electrophysiological, cell biological and molecular studies. Nature Protocols 2 (4):1003–12. doi: 10.1038/nprot.2007.143.
- Surafel, M. T., J. Markandeya, R. F. Matthew, L. C. Damien, and B. Devin. 2018. Rapid induction of vitamin B12 deficiency in Caenorhabditis elegans cultured in axenic medium. Journal of Nutrition & Intermediary Metabolism 13:20–5. doi: 10.1016/j.jnim.2018.08.001.
- Suzuki, T. A, and R. E. Ley. 2020. The role of the microbiota in human genetic adaptation. Science 370 (6521):eaaz6827. doi: 10.1126/science.aaz6827.
- Szewczyk, N. J., E. Kozak, and C. A. Conley. 2003. Chemically defined medium and Caenorhabditis elegans. BMC Biotechnology 3:19. doi: 10.1186/1472-6750-3-19.
- Szewczyk, N. J., I. A. Udranszky, E. Kozak, J. Sunga, S. K. Kim, L. A. Jacobson, and C. A. Conley. 2006. Delayed development and lifespan extension as features of metabolic lifestyle alteration in C. elegans under dietary restriction. The Journal of Experimental Biology 209 (Pt 20):4129–39. doi: 10.1242/jeb.02492.
- Tauffenberger, A, and J. A. Parker. 2014. Heritable transmission of stress resistance by high dietary glucose in Caenorhabditis elegans. PLoS Genetics 10 (5):e1004346. doi: 10.1371/journal.pgen.1004346.
- Tee, L. F., H.-M. Neoh, S. M. Then, N. A. Murad, M. F. Asillam, M. H. Hashim, S. Nathan, and R. Jamal. 2017. Effects of simulated microgravity on gene expression and biological phenotypes of a single generation Caenorhabditis elegans cultured on 2 different media. Life Sciences in Space Research 15:11–7. doi: 10.1016/j.lssr.2017.06.002.
- Templeman, N. M, and C. T. Murphy. 2018. Regulation of reproduction and longevity by nutrient-sensing pathways. The Journal of Cell Biology 217 (1):93–106. doi: 10.1083/jcb.201707168.
- Teshiba, E., K. Miyahara, and H. Takeya. 2016. Glucose-induced abnormal egg-laying rate in Caenorhabditis elegans. Bioscience, Biotechnology, and Biochemistry 80 (7):1436–9. doi: 10.1080/09168451.2016.1158634.
- The Integrative HMP (iHMP) Research Network Consortium. 2019. The integrative human microbiome project. Nature 569 (7758):641–8. doi: 10.1038/s41586-019-1238-8.
- Valentini, S., F. Cabreiro, D. Ackerman, M. M. Alam, M. B. A. Kunze, C. W. M. Kay, and D. Gems. 2012. Manipulation of in vivo iron levels can alter resistance to oxidative stress without affecting ageing in the nematode C. elegans. Mechanisms of Ageing and Development 133 (5):282–90. doi: 10.1016/j.mad.2012.03.003.
- Vanfleteren, J. R. 1976. Large scale cultivation of a free-living nematode (Caenorhabditis elegans). Experientia 32 (8):1087–8. doi: 10.1007/BF01933985.
- Virk, B., J. Jia, C. A. Maynard, A. Raimundo, J. Lefebvre, S. A. Richards, N. Chetina, Y. Liang, N. Helliwell, M. Cipinska, et al. 2016. Folate acts in E. coli to accelerate C. elegans aging independently of bacterial biosynthesis. Cell Reports 14 (7):1611–20. doi: 10.1016/j.celrep.2016.01.051.
- Voisine, C., H. Varma, N. Walker, E. A. Bates, B. R. Stockwell, and A. C. Hart. 2007. Identification of potential therapeutic drugs for Huntington’s disease using Caenorhabditis elegans. PLoS One 2 (6):e504. doi: 10.1371/journal.pone.0000504.
- Wang, N., J. Liu, F. Xie, X. Gao, J.-H. Ye, L.-Y. Sun, R. Wei, and J. Ai. 2015. miR-124/ATF-6, a novel lifespan extension pathway of Astragalus polysaccharide in Caenorhabditis elegans. Journal of Cellular Biochemistry 116 (2):242–51. doi: 10.1002/jcb.24961.
- Wang, Q., Y. Huang, C. Qin, M. Liang, X. Mao, S. Li, Y. Zou, W. Jia, H. Li, C. W. Ma, et al. 2016. Bioactive peptides from Angelica sinensis protein hydrolyzate delay senescence in Caenorhabditis elegans through antioxidant activities. Oxidative Medicine and Cellular Longevity 2016:1–10. doi: 10.1155/2016/8956981.
- Wang, Q., J. Zhang, Y. Jiang, Y. Xiao, X. Li, X. Mao, and Z. Huang. 2021. Caenorhabditis elegans as a model system for discovering bioactive compounds against polyglutamine-mediated neurotoxicity. Journal of Visualized Experiments 175 (175):e63081. doi: 10.3791/63081.
- Wang, S., J. Xue, S. Zhang, S. Zheng, Y. Xue, D. Xu, and X. Zhang. 2020. Composition of peony petal fatty acids and flavonoids and their effect on Caenorhabditis elegans lifespan. Plant Physiology and Biochemistry: PPB 155:1–12. doi: 10.1016/j.plaphy.2020.06.029.
- Wang, X., K. Yi, and Y. Zhao. 2018. Fucoidan inhibits amyloid-β-induced toxicity in transgenic Caenorhabditis elegans by reducing the accumulation of amyloid-β and decreasing the production of reactive oxygen species. Food & Function 9 (1):552–60. doi: 10.1039/c7fo00662d.
- Wang, X., L. Zhang, L. Zhang, W. Wang, S. Wei, J. Wang, H. Che, and Y. Zhang. 2020. Effects of excess sugars and lipids on the growth and development of Caenorhabditis elegans. Genes & Nutrition 15:1. doi: 10.1186/s12263-020-0659-1.
- Watson, E., L. T. MacNeil, A. D. Ritter, L. S. Yilmaz, A. P. Rosebrock, A. A. Caudy, and A. J. M. Walhout. 2014. Interspecies systems biology uncovers metabolites affecting C. elegans gene expression and life history traits. Cell 156 (4):759–70. doi: 10.1016/j.cell.2014.01.047.
- Watson, E., L. T. MacNeil, H. E. Arda, L. J. Zhu, and A. J. M. Walhout. 2013. Integration of metabolic and gene regulatory networks modulates the C. elegans dietary response. Cell 153 (1):253–66. doi: 10.1016/j.cell.2013.02.050.
- Watson, E., V. Olin-Sandoval, M. J. Hoy, C.-H. Li, T. Louisse, V. Yao, A. Mori, A. D. Holdorf, O. G. Troyanskaya, M. Ralser, et al. 2016. Metabolic network rewiring of propionate flux compensates vitamin B12 deficiency in C. elegans. eLife 5:e17670. doi: 10.7554/eLife.17670.
- Webster, A. K., J. M. Jordan, J. D. Hibshman, R. Chitrakar, and L. R. Baugh. 2018. Transgenerational effects of extended dauer diapause on starvation survival and gene expression plasticity in Caenorhabditis elegans. Genetics 210 (1):263–74. doi: 10.1534/genetics.118.301250.
- Wei, W, and G. Ruvkun. 2020. Lysosomal activity regulates Caenorhabditis elegans mitochondrial dynamics through vitamin B12 metabolism. Proceedings of the National Academy of Sciences of the United States of America 117 (33):19970–81. doi: 10.1073/pnas.2008021117.
- Wellen, K. E., G. Hatzivassiliou, U. M. Sachdeva, T. V. Bui, J. R. Cross, and C. B. Thompson. 2009. ATP-citrate lyase links cellular metabolism to histone acetylation. Science 324 (5930):1076–80. doi: 10.1126/science.1164097.
- Wickramasinghe, K., J. C. Mathers, S. Wopereis, D. S. Marsman, and J. C. Griffiths. 2020. From lifespan to healthspan: the role of nutrition in healthy ageing. Journal of Nutritional Science 9:e33. doi: 10.1017/jns.2020.26.
- Williams, A. B., F. Heider, J. E. Messling, M. Rieckher, W. Bloch, and B. Schumacher. 2019. Restoration of proteostasis in the endoplasmic reticulum reverses an inflammation-like response to cytoplasmic DNA in Caenorhabditis elegans. Genetics 212 (4):1259–78. doi: 10.1534/genetics.119.302422.
- Wood, J. G., B. Rogina, S. Lavu, K. Howitz, S. L. Helfand, M. Tatar, and D. Sinclair. 2004. Sirtuin activators mimic caloric restriction and delay ageing in metazoans. Nature 430 (7000):686–9. doi: 10.1038/nature02789.
- Wu, D., Y. Chen, X. Wan, D. Liu, Y. Wen, X. Chen, and C. Zhao. 2020. Structural characterization and hypoglycemic effect of green alga Ulva lactuca oligosaccharide by regulating microRNAs in Caenorhabditis elegans. Algal Research 51:102083. doi: 10.1016/j.algal.2020.102083.
- Xiang, Y., J. Zhang, H. Li, Q. Wang, L. Xiao, H. Weng, X. Zhou, C. W. Ma, F. Ma, M. Hu, et al. 2017. Epimedium polysaccharide alleviates polyglutamine-induced neurotoxicity in Caenorhabditis elegans by reducing oxidative stress. Rejuvenation Research 20 (1):32–41. doi: 10.1089/rej.2016.1830.
- Xiao, Y., Q. Wang, X. Mao, X. Li, and Z. Huang. 2022. Modulation of redox and aging related signaling pathways and biomarkers by naturally derived peptides. In Redox signaling and biomarkers in aging, ed. U. Çakatay, 229–54. Cham: Springer Nature. doi: 10.1007/978-3-030-84965-8_11.
- Yoon, D. S., D. S. Cha, Y. Choi, J. W. Lee, and M.-H. Lee. 2019. MPK-1/ERK is required for the full activity of resveratrol in extended lifespan and reproduction. Aging Cell 18 (1):e12867. doi: 10.1111/acel.12867.
- Young, L. E. A., C. Shoben, K. Ricci, and D. C. Williams. 2019. Genetic analysis of KillerRed in C. elegans identifies a shared role of calcium genes in ROS-mediated neurodegeneration. Journal of Neurogenetics 33 (1):1–9. doi: 10.1080/01677063.2018.1531857.
- Zanni, E., C. Laudenzi, E. Schifano, C. Palleschi, G. Perozzi, D. Uccelletti, and C. Devirgiliis. 2015. Impact of a complex food microbiota on energy metabolism in the model organism Caenorhabditis elegans. BioMed Research International 2015:621709. doi: 10.1155/2015/621709.
- Zečić, A., I. Dhondt, and B. P. Braeckman. 2019. The nutritional requirements of Caenorhabditis elegans. Genes & Nutrition 14:15. doi: 10.1186/s12263-019-0637-7.
- Zhang, Y., H. Lu, and C. I. Bargmann. 2005. Pathogenic bacteria induce aversive olfactory learning in Caenorhabditis elegans. Nature 438 (7065):179–84. doi: 10.1038/nature04216.
- Zhang, H., N. Pan, S. Xiong, S. Zou, H. Li, L. Xiao, Z. Cao, A. Tunnacliffe, and Z. Huang. 2012. Inhibition of polyglutamine-mediated proteotoxicity by Astragalus membranaceus polysaccharide through the DAF-16/FOXO transcription factor in Caenorhabditis elegans. The Biochemical Journal 441 (1):417–24. doi: 10.1042/BJ20110621.
- Zhang, L., D. G. Gualberto, X. Guo, P. Correa, C. Jee, and L. R. Garcia. 2015. TMC-1 attenuates C. elegans development and sexual behaviour in a chemically defined food environment. Nature Communications 6:6345. doi: 10.1038/ncomms7345.
- Zhang, J., R. Shi, H. Li, Y. Xiang, L. Xiao, M. Hu, F. Ma, C. W. Ma, and Z. Huang. 2016. Antioxidant and neuroprotective effects of Dictyophora indusiata polysaccharide in Caenorhabditis elegans. Journal of Ethnopharmacology 192:413–22. doi: 10.1016/j.jep.2016.09.031.
- Zhang, J., A. D. Holdorf, and A. J. Walhout. 2017. C. elegans and its bacterial diet as a model for systems-level understanding of host-microbiota interactions. Current Opinion in Biotechnology 46:74–80. doi: 10.1016/j.copbio.2017.01.008.
- Zhang, J.-J., J.-J. Hao, Y.-R. Zhang, Y.-L. Wang, M.-Y. Li, H.-L. Miao, X.-J. Zou, and B. Liang. 2017. Zinc mediates the SREBP-SCD axis to regulate lipid metabolism in Caenorhabditis elegans. Journal of Lipid Research 58 (9):1845–54. doi: 10.1194/jlr.M077198.
- Zhang, J., X. Li, M. Olmedo, A. D. Holdorf, Y. Shang, M. Artal-Sanz, L. S. Yilmaz, and A. J. M. Walhout. 2019. A delicate balance between bacterial iron and reactive oxygen species supports optimal C. elegans development. Cell Host & Microbe 26 (3):400–11.e3. doi: 10.1016/j.chom.2019.07.010.
- Zhang, M., X. Yang, W. Xu, X. Cai, M. Wang, Y. Xu, P. Yu, J. Zhang, Y. Zheng, J. Chen, et al. 2019. Evaluation of the effects of three sulfa sweeteners on the lifespan and intestinal fat deposition in C. elegans. Food Research International (Ottawa, Ont.) 122:66–76. doi: 10.1016/j.foodres.2019.03.028.
- Zhang, M., S. Chen, Y. Dai, T. Duan, Y. Xu, X. Li, J. Yang, and X. Zhu. 2021. Aspartame and sucralose extend the lifespan and improve the health status of C. elegans. Food & Function 12 (20):9912–21. doi: 10.1039/d1fo01579f.
- Zheng, S.-Q., A.-J. Ding, G.-P. Li, G.-S. Wu, and H.-R. Luo. 2013. Drug absorption efficiency in Caenorhbditis elegans delivered by different methods. PLoS One 8 (2):e56877. doi: 10.1371/journal.pone.0056877.
- Zheng, J., F. L. Greenway, S. B. Heymsfield, W. D. Johnson, J. F. King, M. J. King, C. Gao, Y.-F. Chu, and J. W. Finley. 2014. Effects of three intense sweeteners on fat storage in the C. elegans model. Chemico-Biological Interactions 215:1–6. doi: 10.1016/j.cbi.2014.02.016.
- Zheng, J., C. Gao, M. Wang, P. Tran, N. Mai, J. W. Finley, S. B. Heymsfield, F. L. Greenway, Z. Li, D. Heber, et al. 2017. Lower doses of fructose extend lifespan in Caenorhabditis elegans. Journal of Dietary Supplements 14 (3):264–77. doi: 10.1080/19390211.2016.1212959.
- Zhong, G., W. Pan, Z. Huang, K. Guo, J. Hu, P. Liu, S. Chen, Y. Wang, L. Ai, and Z. Huang. 2021. Physicochemical and geroprotective comparison of Nostoc sphaeroides polysaccharides across colony growth stages and with derived oligosaccharides. Journal of Applied Phycology 33 (2):939–52. doi: 10.1007/s10811-021-02383-6.