Abstract
There is a growing interest in using green technologies in the food industry. As a green processing technique, ultrasound has a great potential to be applied in many food applications. In this review, the basic mechanism of ultrasound processing technology has been discussed. Then, ultrasound technology was reviewed from the application of assisted food processing methods, such as assisted gelation, assisted freezing and thawing, assisted crystallization, and other assisted applications. Moreover, ultrasound was reviewed from the aspect of structure and property modification technology, such as modification of polysaccharides and fats. Furthermore, ultrasound was reviewed to facilitate beneficial food reactions, such as glycosylation, enzymatic cross-linking, protein hydrolyzation, fermentation, and marination. After that, ultrasound applications in the food safety sector were reviewed from the aspect of the inactivation of microbes, degradation of pesticides, and toxins, as well inactivation of some enzymes. Finally, the applications of ultrasound technology in food waste disposal and environmental protection were reviewed. Thus, some sonoprocessing technologies can be recommended for the use in the food industry on a large scale. However, there is still a need for funding research and development projects to develop more efficient ultrasound devices.
Introduction
Innovative thermal and non-thermal technologies have displayed immense potential owing to their wide-scale application in food processing. Among these technologies, ultrasound is widely used for various purposes in food science, including dispersion, catalysis, extraction, nanoparticle synthesis, and graphene generation (Muthoosamy and Manickam Citation2017). Ultrasound is gaining popularity due to its capacity to preserve natural freshness, taste, and nutritional elements in food while consuming less energy (Wang et al. Citation2019). Sonoprocessing utilizes ultrasound for transmitting energy to produce chemical and/or physical effects in both laboratories and in an industrial medium stream. Ultrasound is divided into two types based on the frequency range: low-intensity ultrasound (0–1 W. cm2, >100 kHz) and high-intensity ultrasound (>1 W cm2, 20–100 kHz) (Nowacka and Wedzik Citation2016). Both types of ultrasound treatments have practical applications in various disciplines, most notably in food processing and food safety-related sectors. As a nondestructive method, low-intensity ultrasound is utilized to monitor the changes in physicochemical properties during food preparation. In comparison, high-intensity ultrasound is used for many applications, including emulsification, and inactivation of enzymes and bacteria that leads to the extension of the shelf life of food products (Aadil et al. Citation2015; Wang et al. Citation2019).
The main ultrasound effects resulted from acoustic cavitation, including the formation and collapse of air bubbles in the treated system (Coussios et al. Citation2007; Yi et al. Citation2018; Gevari et al. Citation2020; ). This mechanical action of mixing and disruption promotes mass transfer between the sample and solvent. The cavitation impact of ultrasound during the sonication process mainly depends on product attributes, ultrasonic nature, and ambient circumstances (Boateng and Nasiru Citation2019). In this process, the ultrasound-generated bubbles grow over multiple oscillations and either remain stable (known as stable cavitation) or collapse violently after some oscillations (labelled as, transient cavitation). In the sonication treatment process, two types of bubbles are produced on a structural basis: (a) non-linear; generating large globule clouds with a balance scale during pressure cycles known as steady cavitation bubbles, and (b) non-constant; rapidly falling out and breaking down into smaller transient cavitation bubbles (Majid, Nayik, and Nanda Citation2015). The acoustic cavitation induces sonomechanical and sonochemical effects which are commonly used in sonoprocessing (Chia et al. Citation2019). Sonomechanical effects, including shock waves, high pressure, high-speed liquid jet, and turbulence, are caused by sonic energy in the medium. Whereas sonochemical effects, in general, make use of cavitation effects to accelerate or catalyze a certain chemical process (Rubio, Blandford, and Bond Citation2016).
Figure 1. Acoustic cavitation generated by ultrasound devices, adapted from Taha et al. (Citation2020) with permission.
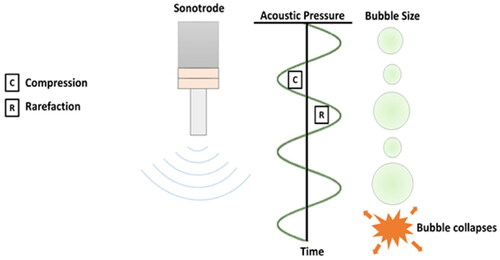
Ultrasound is used in food freezing, thawing frozen foods, drying, sterilizing, emulsifying, and extracting (Awad et al. Citation2012). Recently, ultrasound has been used in meat processing, quality control, crystallization, and performance modification (Ashokkumar Citation2015). Furthermore, to ascertain carcass distinguishing quality in meat processing, quality standards such as tenderness, protein extraction, gelatin, meat products restructuring, restructured products salt reduction, and maximizing cooking yield currently use ultrasound technology as a quick, comparatively inexpensive, simple, and tried and true alternative processing means (Boateng and Nasiru Citation2019). Ultrasound in food processing provides several benefits, such as more efficient mixing, faster energy transfer, easier cleaning and operating process, small equipment size, selective extraction, and faster response to process extraction control (Boateng and Nasiru Citation2019).
The aim of this review is to discuss the recent advances in sonoprocessing. The main applications, process mechanisms, insights into optimizing treatment parameters and challenges will be reviewed. In this review, we focused on the recent applications of ultrasound which are not fully addressed in the recently published review articles. This review could help researchers to develop novel ultrasonic-based green processing technologies for various industrial applications.
The application of power ultrasound in food science
Provide innovative or assist food processing methods
Ultrasound is widely applied in food systems to alter biochemical and physical characteristics (Ali et al. Citation2021). Moreover, ultrasound is also applied as a nondestructive analysis in-process quality control in the food industry, medical industry, and cosmetics industry (Astráin-Redín et al. Citation2020). Ultrasound is well known as a promising, innovative, safe, and effective eco-friendly technology that can be utilized to enhance the efficacy of the numerous food technologies, processes, and the quality of end products (Astráin-Redín et al. Citation2020; Zhang et al., Citation2019). Ultrasound-assisted food processing classically involves less time, water, and energy in manufacturing, processing, extraction, and heat treatments. In the food industry, manufacturing efficacy can be improved by using novel substitutes to conventional procedures and processing techniques (Singla and Sit Citation2021). Recently, many studies investigated the applications of power ultrasound in food processing, including extraction, emulsification, ultrasound-assisted gelation, freezing and thawing, drying, ultrasound-assisted crystallization, tenderization, and other applications such as cooking, separation and filtration, cutting, degassing, foaming, and defoaming (Xiong et al. Citation2018, Taha et al. Citation2020, Bhargava et al. Citation2021, Nowacka, Dadan, and Tylewicz Citation2021, Singla and Sit Citation2021). This section will review the recent and innovative studies regarding food processing approaches through power ultrasound.
Ultrasound-assisted gelation
Ultrasound treatment can influence the gelling properties of food proteins. Our study found that ultrasound can enhance the water-holding capacity (WHC) and gel strength of soy protein isolate (SPI) gels induced by calcium sulfate (Hu, Li-Chan, et al. Citation2013) and glucono-δ-lactone (Hu, Fan, et al. Citation2013). Recently, we studied the effects of ultrasound and different gelation mechanisms (salt-induced, acidic, and enzymatic gelation) on the performance of ultrasound-induced SPI gels as a carrier for β-carotene. It was concluded that ultrasound-treated transglutaminase-induced emulsion gels had higher WHC, β-carotene bio-accessibility, and gel strength than the emulsion gels prepared using mechanical homogenization and other materials gelation mechanisms (Geng et al. Citation2022). The possible mechanisms of the ultrasound effects on the gelling properties of proteins are discussed in the following context. First, ultrasound can reduce the particle size of protein molecules and improve the solubility of proteins (Hu, Fan, et al. Citation2013; Hu, Wu, et al. Citation2013). These changes can improve the dispersibility of proteins, forming a denser gel network. Moreover, ultrasound can unfold the protein structure and expose the hydrophobic groups to the surface of protein molecules. This could improve the gelling properties of proteins as the hydrogen bridges, and hydrophobic interactions could promote the gel network formation (Zheng et al. Citation2019). Furthermore, ultrasound could also expose the sulfhydryl groups to the surface of protein molecules, facilitating the formation of S–S bonds. S–S bonds contribute significantly to the gel network and gel strength (Hu, Fan, et al. Citation2013; Hu, Wu, et al. Citation2013; Hu, Li-Chan, et al. Citation2013). Ultrasound was found to soften the gel produced from milk (10% protein) and thus improve high-protein yogurt quality. This could be due to the effects of cavitation and shear forces induced by sound waves (Körzendörfer et al. Citation2019).
A recent study by Zou et al. (Citation2021) reported the impact of ultrasound-assisted konjac glucomannan (KGG) treatment on the properties of chicken plasma protein (CPP) gelation through various treatments as follow: (1) Control or untreated plasma protein gelation, (2) Gelation by adding KGG, (3) Gelation by ultrasound treatment (UG), and (4) Gelation added KGG combined with ultrasound (KGUG). The study exhibited that the WHC and gelation strength of all treatments were significantly improved compared with control. The content of bound water was presented in group UG, and the average particle size of UG and KGUG decreased significantly after ultrasound application. Thus, the gelation properties of ultrasound-treated CPP combined with KGG had great gelling characteristics. Another study demonstrated that ultrasound-assisted gelation was a low-cost green approach to modify the molecular orientation and gelation properties attributed to the native functional properties and chemical composition of the plant proteins (Bangar et al. Citation2022). The improved gelation aspects thru ultrasound power could be a novel technology in the nearest future that increase the utility of proteins in food formulation and processing.
Freezing and thawing
Freezing is an effective preservation method that is applied to foodstuffs, and thawing is the reverse course of freezing. Though conventional freezing or thawing techniques have low efficiency, ultrasound is an effective method to enhance the freezing and thawing performance of foods. Appling ultrasound technology is effective in maintaining the microstructure, along with drip loss reduction, decrease in texture and color changes, and retention of natural nutrients of foods during freezing. Meanwhile, enhancing the quality is also visualized in thawed food commodities by ultrasound-assisted thawing approaches. Researchers have demonstrated that ultrasound is a better operative technique for accelerating ice nucleation and freezing, controlling the size of ice crystals in frozen foods (Kiani and Sun Citation2017). Ultrasound effectively increases the freezing speed of fresh foods and improves the quality of frozen foodstuffs.
Mechanism of ultrasound-assisted freezing
On applying ultrasound, rapid conventional cooling occurs and furnishes uniform seeding, which brings shorter dwell time. More seeds decrease the ultimate crystal size and give rise to slight damage to the cells (). Thus, by boosting heat transfer, accelerated cooling can be attained. Initially, the acoustic cavitation formulates a microstreaming, leading to a concentrated collision between micro-particles in the solution, thereby creating thinner solid-liquid interfaces (Xin, Zhang, and Adhikari Citation2014; Qiu et al. Citation2020). In the next step, the formation and collapse of cavitation bubbles in the transport media can induce ultra-high pressure of 100 MPa. The rapid nucleation is promoted by the high supercooling degree produced by the high pressure (Li et al. Citation2020). Lastly, the primary nucleation process is brought about by the cavitation bubbles of a certain size, and secondary nucleation is apprehended by the small pieces from large dendritic ice crystals (Dalvi-Isfahan et al. Citation2017).
Figure 2. Schematic diagram of cavitation bubbles breaking the already formed nuclei into smaller ones. Source: This figure was created with BioRender.com
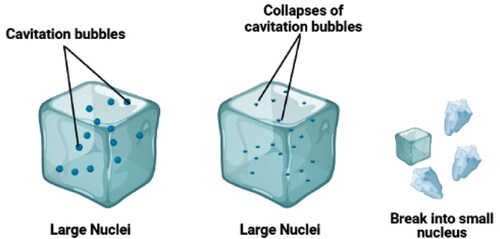
Ultrasound treatment can accelerate the process of heat transfer and thus produce smaller ice crystals, preserving the nutritional values of frozen food products (James, Purnell, and James Citation2015, Mahato, Zhu, and Sun Citation2019). Several studies have projected the impact of freezing aided by ultrasound on the quality of food products, such as microstructure, chemical, and physical changes. Results exhibited that in cut apples, ultrasound application at conditions of 40 kHz; 0.23 W/cm2 for 120 s reduced the freezing time to 8%, compared to immersion freezing without sonication, probably due to the faster formation of the nucleus in a crystal-free media (Delgado, Zheng, and Sun Citation2009). The freezing phase, ultrasound power, and duration are three factors influencing the mass and heat transfer rates during freezing (Taylor Citation2015). The increase in freezing rate is obtained due to strong ultrasound vibration produced by high-power ultrasound. The freezing rate could be enhanced by selecting an appropriate ultrasound duration without thermal effect for various foods, as mentioned in .
Table 1. Application of ultrasound-assisted freezing of various food matrices.
Research proved that in radish “ultrasound-assisted osmo-dehydro-freezing” pretreatment is also exhibited as a potential tool for better retention of its microstructure (Taylor et al. Citation2014). Ultrasonic irradiation with various frequencies could reduce the ice crystals size created within potatoes (Zhu, Zhang, and Sun Citation2019). The ultrasound application during immersion freezing vividly enhanced the freezing rate, and the creation of smaller ice crystals, consequently minimizing the drip loss of food materials after thawing (Shi et al. Citation2019; Zhang, Hui, et al. Citation2019). Results also showed that with the application of ultrasound combined with freezing and thawing resulted in increased porosity and decreased particle density in blueberries (Vaccinium corymbosum L.). Moreover, ultrasound-assisted freezing produced gummy, less chewy, and softer berries (Nowak, Zielinska, and Waszkielis Citation2019). The higher porosity of the fruits might attribute to the hot spots and higher temperatures formed because of the acoustic cavitation.
Parameters such as the ice bubble concentration in the solution, ultrasound duration and power, and supercooling degree are optimized for improving the ice nucleation during freezing, which should be specifically selected for each food product. For ice crystal formation, a higher gas bubble concentration is well-suited, while a higher supercooling degree is suitable for the initiation of ice nucleation. Also, a high-power ultrasound at an appropriate range is desirable for ice nucleation. In contrast, low-power ultrasound is not strong enough to initiate nucleation. The nucleation process is also affected by the thermal effect induced at high-power ultrasound levels. Acoustic cavitation caused by power ultrasound substantially influences nucleation. The gas bubbles development facilitates the crystallization of nuclei or influence the crystallization process by collapsing or inducing supersaturated degrees high or supercooling. When a successive oscillation is provided by power ultrasound, if the gas bubbles are steady, it results in the initiation of microstreaming, causing an improvement of the mass and heat transfer effectiveness. This technology is pertinent in crystallizing foodstuffs like triglyceride oils, milk fat, and ice cream. Also, it prevents the encrustation of crystals on cooling elements, ensuring appropriate heat transfer during the cooling process.
Application of ultrasonic irradiation in thawing
The thawing process can be augmented by the heat produced within the frozen food constituents, thus preventing food’s microbial and enzymatic spoilage. Also, a control can be driven by problems such as the dripping and surface heating and the extreme protein denaturation consequential of the conventional thawing approaches like microwave thawing or air thawing and hot water.
Also, ultrasound application in thawing is limited because of localized heating, meager penetration, and high-power necessity. Still, the acoustic action can be applied as an additional treatment to traditional thawing to hasten the thawing rate of frozen foods for the speedy phase change (). Liu et al. observed that in mango pulp, the thawing time shortened as the ultrasound intensity increased with maintained sensory and nutritional quality (Liu, Chen, et al. Citation2019). This could be attributed to the improvement in heat transfer induced by high-speed jets of ultrasound. It was also indicated that ultrasound treatment (25 kHz and 0.6 W/cm2) pointedly decreased the thawing time by 87% while retaining the technological and textural features of pork samples (Gambuteanu and Alexe Citation2015). Cheng et al. observed that in edamame, ultrasound-assisted thawing at a power level of 1.8 W/g demonstrated the greatest preservation of chlorophyll, ascorbic acid, and original hardness, along with reduction of the drip loss after thawing. Besides, ultrasound influenced the noticeable ascorbic acid reduction at a power level of 2.4 W/g (Cheng, Zhang, and Adhikari Citation2014).
Figure 3. Phase change region of conventional and ultrasound-assisted thawing. Source: This figure was created with BioRender.com
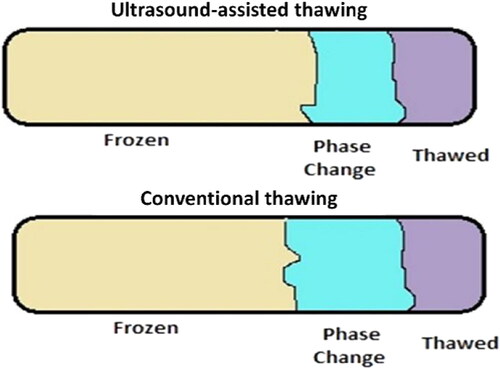
Some other technologies were combined with ultrasound thawing to overcome the adverse effects of using ultrasound treatment alone. Cai et al. found a reduction in water migration and protein denaturation by applying ultrasound in combination with infrared and ultrasound in combination with microwave (Cai et al. Citation2019). Numerous aspects influence the thawing progression, counting ultrasound parameters, sample orientation, and thawed product composition. Ultrasound frequency is an essential factor in boosting the thawing process. To prevent the meager penetration of ultrasound and attain a suitable surface temperature, the applied frequency must be in the low kilohertz range. In contrast, ultrasound generally works at a frequency more than 20 kHz. The ultrasound’s attenuation coefficients in case of parallel transmission were higher than that of perpendicular. The total thawing time is affected by the structure of thawed items that can be influenced by the transmission coefficient of ultrasonic. Thus, it can be inferred that ultrasound-assisted thawing is an innovative technique utilized for the thawing of frozen foods to accelerate the thawing rate and retain its initial quality attributes (). It has also been proven to be competent in preserving the quality of foods during the thawing process. With the efficient application of ultrasound, the thawing rate can be improved, avoid surface heating and extreme product dehydration, and better color retention and inhibition of lipid oxidation. The optimal ultrasound frequencies or intensities must be sensibly selected through pre-experiments to gain a fast-thawing rate and high-quality thawed foods after ultrasound-assisted thawing technologies for different foodstuffs to preserve a balance between thawing rate and food quality.
Table 2. Application of ultrasound in meat tenderization.
Ultrasound-assisted crystallization
Ultrasound can be applied to control crystallization in a process called sonocrystallization, as has been demonstrated for pharmaceutical applications (Ruecroft Citation2007; Belca et al. Citation2019). Moreover, ultrasound-assisted crystallization has been studied in food, especially oil or oil-wax gels. It can also be necessary to either dampen (e.g. honey) or promote (e.g. chocolate) crystallization in the food industry. Crystallization occurs when a solute reaches a concentration above its equilibrium in some area within a liquid phase and thus starts forming organized solid particles (Deora et al. Citation2013).
Through local changes in pressure and temperature, acoustic cavitation can induce nucleation at lower supersaturation levels than mechanical agitation-based processes (Luche Citation1998; Ruecroft Citation2007; Deora et al. Citation2013). On the other hand, the crystal growth rate and, thus, final size are reduced in sonicated systems (Li et al. Citation2021; Nalajala and Moholkar Citation2011). As an additional benefit, it presents a well-defined and thus controllable and reproducible initial point for crystallization and may eliminate the need for crystal seeding (Deora et al. Citation2013). As for applications, sonocrystallization has been shown to reduce oil migration in palm kernel fat (da Silva et al. Citation2020) and enhance the properties of some trans-free fats, such as elasticity, under the right conditions (Lee et al. Citation2018). Especially the latter case is relevant since a push for the replacement of partially hydrogenated oils, making it necessary for the food industry to pursue alternatives with the same techno-functional properties. Sonocrystallization can also increase the nucleation rate of lactose, enhancing lactose crystallization. This can greatly increase lactose recovery from whey while also modifying crystal shapes and sizes. Modifying the power and frequency of ultrasound treatment was also found to impact the recovery rate and purity (Koca, Urgu, and Saatli Citation2018). Moreover, our recent study concluded that ultrasound could enhance the crystal strength and physical properties of nut oil/candelilla wax oleogels as ultrasound could create more nucleation sites (Li et al. Citation2021). For these above narrations, ultrasound could also facilitate the interactions and arrangement of crystals, increasing the crystal intensity of oleogels.
Recently, the study of lysozyme crystallization in hydrogel media under ultrasound irradiation performed by Savchenko et al. (Citation2022) stated that sonocrystallization implies the application of ultrasound radiation to control the crystal growth and nucleation depending on several factors, that is, the intensity and actuation time. Its application allows to induce nucleation at lower supersaturations than required under standard conditions. Moreover, the study concluded that ultrasound as an externally generated low-energy Sonocrystallization-based irradiation of protein in gel media was significant and reproducible in crystal-size populations.
Moreover, based on the study of Sabnis, Singh, and Gogate (Citation2022), who investigated the improvement in azithromycin (ARZ) recrystallization using ultrasound for size reduction; and concluded that ultrasound-assisted antisolvent crystallization at low temperatures, mainly less than (10 °C) could enhance various characteristics, particularly the shape and mean size of the particles with improvements in the bioavailability of ARZ.
Tenderization
The quality of meat tenderness determined by its texture is considered one of the most appreciated traits of consumers. Currently, ultrasound is applied to enhance the quality, taste, and tenderness, which characterize the major factor of consumer satisfaction. Ultrasound applications have been used at 15–130 kHz, 1.89 to 64 W/cm2 for 33 s to 90 min to enhance the meat tenderness (Zhou, Xu, and Liu Citation2010; Stadnik and Dolatowski Citation2011; Xiong et al. Citation2012; Siró et al. Citation2009) and curtails the duration of aging without even influencing other quality attributes (Xue et al. Citation2018; Wang, Kang, et al. Citation2017; Wang, Ma, et al. Citation2017).
Mechanism of tenderization
The tenderness is determined based on the size of the longitudinally organized fiber bundles in muscles, delimited by the connective tissue septa forming the perimysium. This effect was mostly ascribed to the mechanical rupture of myofibrillar protein structures (Jayasooriya et al. Citation2007), collagen macromolecule fragmentation, migration of proteins, and other constituents. Thus, when meat is either sonicated in baths/by probes hastens the proteolysis/protein denaturation process with a rise of desmin and troponin-T degradation and myofiber fracture along Z-lines and I-bands (Barekat and Soltanizadeh Citation2017a). These effects have also been associated with proteolysis activation by releasing intracellular calcium ions and/or lysosome cathepsins that activate calpains, and reduce aging (Amiri and Shari Citation2018). The characteristics of collagen, especially its thermal properties, are significantly affected by ultrasound without affecting the insoluble collagen composition (Contreras, Benedito, and Bon Citation2017). Similarly, ultrasound effects on the solubility of collagen throughout cooking have been studied (Chang et al. Citation2015) when meat was treated in ultrasonic baths (40 kHz and 1500 W). Hence, it has been proved that ultrasound technology can result in better physical traits of meat foods, like water-binding capacity and cohesiveness tenderness. Both physical and chemical changes occur in the sonicated meat surface, bringing alterations in tenderness and other physical characteristics ().
Figure 4. Process of acoustic cavitation. Ultrasonic irradiation > radical formation > shock waves > sonoluminescence > fluid jet > bubble jet > surface erosion > bubble nucleation > cracks. Source: This figure was created with BioRender.com
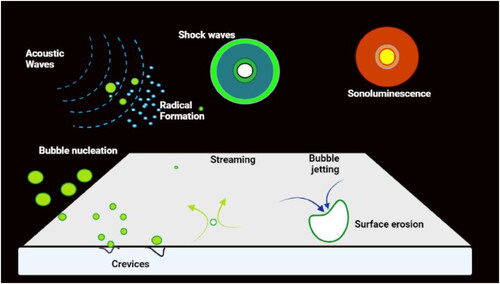
Microstructural tissue changes resulting from high-intensity ultrasound (HIU) confirmed an improved meat texture, either when HIU has been applied alone or when HIU is combined with other techniques like marination or extrinsic enzyme addition such as papain. Microstructural changes comprise the separation and breakage of myofibrils through z- and m-lines, desmin and troponin-N degradation, changes in secondary structures of myofibrillar proteins, and denaturation of myofibrillar proteins myosin (Alves et al. Citation2018; Yeung and Huang Citation2017; Fan et al. Citation2016; Gómez-Salazar et al. Citation2018).
To intensify the curing process of the dry ham and thereby improve the texture, the application of HIU as an emerging technology could be utilized. Several investigations have been directed to develop approaches to improve the tenderness of the meat. The application of ultrasound systems at various sonication intensities, frequencies, and times was studied (). Most studies agreed that ultrasound can enhance meat tenderness and cuts the aging period without changing other quality parameters. The fracture degree of muscle fiber is determined by Warner-Bratzler shear force, reflecting the muscle tenderness. HIU enhanced meat tenderization as several studies reported a significant reduction of shear force after sonication. Recently, HIU (at 500 W for 30 min) decreased the muscle fiber diameter and shear forces of chicken gizzard by 26.2% and 27.1%, respectively. It was concluded that sonication could enhance the tenderness of chicken gizzards (Du et al. Citation2021). The strong acoustic cavitation could decrease the meat’s hardness by softening muscle tissue and promoting muscle fiber fracture. The available information specifies that ultrasound has a crucial effect on meat tenderization, while the applied parameters need to be well-known while designing large-scale sonicators.
Cooking
The principle of ultrasound cooking is uniform heat transfer (Bhargava et al. Citation2021). Chemat, Khan, and Zill-e-Huma (Citation2011) reviewed the benefits of ultrasound cooking compared to conventional techniques. When foods are exposed to high temperatures, the external layer of food may be overheated with the internal inadequate cooking, which is a key to the quality drop. Instead, ultrasound can offer improved cook transfer properties, which is crucial to avoid food quality decline. It was revealed that ultrasound cooking of meat systems resulted in more moisture retention, an increase in cooking speed, and proved to have high energy efficacy, suggesting that it could provide a rapid and innovative strategy to improve the textural and sensory qualities and increase the water-binding properties of cooked meat products (Su et al. Citation2018; Gonzalez-Gonzalez et al. Citation2020). A study recommended that ultrasound cooking provides humid pre-cooked or cooked meat and reduces the loss of essential nutrients during the cooking process (Bhargava et al. Citation2021). As with other ultrasound applications in foodstuffs, it was stated that ultrasound-cooking in fruits and vegetables could enhance the retention of nutrients, improve heat transfer rate, and enhance sensory attributes (Singla and Sit Citation2021). The acoustic cavitation, high pressure, and shear jets of ultrasound could soften the tissues of fruits and vegetables, facilitating the release of moisture and thus enhancing the heat transfer rate and maintaining the quality of cooked products.
Separation and filtration
Power ultrasound filtration has been applied in the food industry to produce solid isolates from a liquid or a solid-free liquid (Chemat, Khan, and Zill-e-Huma Citation2011). Contamination and residues accumulation are the main issues in membrane filtration and cause a decrease in filtration efficiency (Kyllönen, Pirkonen, and Nyström Citation2005). However, ultrasound could overcome these issues by increasing the flux by breaking the concentration polarization and cake layer at the membrane surface without affecting the intrinsic permeability of the membrane (Camara, Doan, and Lohi Citation2020; Singla and Sit Citation2021). It was found that fouling visualization with scanning electron microscopy (SEM) showed that ultrasound successfully removed membrane fouling (Al-Juboori et al. Citation2021). Therefore, ultrasound filtration could reduce safety concerns and increase the efficacy of processed items (Bhargava et al. Citation2021). Chemat, Khan, and Zill-e-Huma (Citation2011) stated the applications and benefits of ultrasonic filtration in the food industry, for example, the separation of fruit beverages and its utility for enhancing the industrial wastewater filtration. Moreover, optimized ultrasound intensity is essential for preventing the filters’ damage. Furthermore, Singla and Sit (Citation2021) stated another recent application of ultrasound-filtration as a novel method in dairy emulsions separation and wastewater filtration. In this review, it has been claimed that splitting an emulsion into its water and oil components required applying high-intensity ultrasonic waves with a low frequency (about 30 kHz). However, this idea needs to be further studied because high-intensity ultrasonic waves can readily induce the opposite effect by forming a finer emulsion or dispersion. Acoustic filtration is effectively used to enhance wastewater in businesses where the procedure is typically considered unpleasant. However, adjusting velocity in ultrasound is crucial to prevent damage that forms inside filters. When paired with filters, ultrasound prolongs the life of the filter by preventing membrane caking and clogging, allowing the cavity around the filter surface to remain open. Ultrasound combined with adsorption and membrane filtration was used to remove emerging contaminants from wastewater (Naddeo et al. Citation2020). In this process, amoxicillin, carbamazepine, and diclofenac were removed by around 99%. The acoustic and mechanical forces of ultrasound constantly clean the membrane and thus facilitate the adsorption. Another reason for the effects of ultrasound on filtration efficiency could be that ultrasound irradiation could facilitate the contaminants degradation via sonolytic action and enhance intra-particle diffusion and liquid mass transfer in sonication-adsorption filtration (Naddeo et al. Citation2020).
Cutting
Various foods are prepared in considerable amounts in the market; therefore, they must be reduced for consumption and marketing purposes. Cutting-based power ultrasound via ultrasonic probes or acoustic equipment as a knife-edge is used, which vibrates at a specific ultrasonic frequency longwise (Astráin-Redín et al. Citation2020). The main mechanism of sonication cutting is the cavitation phenomenon and/or high-frequency vibrations of the cutting blades (Chavan et al. Citation2022). This novel technology could enhance food processing performance, improve overall quality, reduce product waste, and lessen food costs, enhancing the cutting effectiveness process (Schneider, Zahn, and Rohm Citation2008, Bhargava et al. Citation2021). Ultrasound-cutting characteristics depend on the food category and its state, like thawing or freezing. Ultrasonic vibrations provide auto-cleaning of the blade and improve the hygiene status. This vibration avoids food adherence on the edge and decreases the microorganism’s growth and development on the surface (Chemat, Khan, and Zill-e-Huma Citation2011). Cutting has been applied to several food products such as heterogeneous, fragile, sticky, or cheese or bakery products like cakes, bread, pastries, and biscuits. The increased cutting accuracy can decrease losses caused by crumbs or cracks, offering a good standardization of weight and portion sizes (Liu, Wang, and Zhang Citation2020). Therefore, the cutting-based ultrasound approach offers several advantages, including improving cutting quality, decreasing food waste, and reducing product deformation. Thus, ultrasound-based cutting can be considered an effective method in novel food processing.
Degassing
The boiling and reducing pressure are the two communal procedures used for degassing the food liquid that contains gases such as carbon dioxide, dissolved oxygen, and nitrogen. In contrast, degassing-assisted ultrasonic technology is highly advantageous and visible when ultrasound-degassing is used with regular tap water inside. Degassing occurs in the liquid when the acoustic waves via rapid vibration of gas bubbles promote the growth of air bubbles to a large size, allowing them to rise in the top of the liquid against gravity (Tervo, Mettin, and Lauterborn Citation2006). Ultrasound can be utilized to degas carbonated drinks like beer before bottling; this process is called demobbing. In processing carbonated beverages, the aim is to remove the air from the liquid surface to avoid sensorial damage to the food product by oxygen and microorganisms. Ultrasonic-degassing is mainly rapid in aqueous systems; nevertheless, removing gas in viscous liquids, for example, melted chocolate, is challenging (Chemat, Khan, and Zill-e-Huma Citation2011, Bhargava et al. Citation2021). Degassing carbonated beverages by ultrasound has various advantages compared with mechanical agitation, such as the overflow of beverages, decreased broken bottles, and requiring less time (Singla and Sit Citation2021). The rapid freezing technique for porous foods using tissue pre-degassing treatment followed by ultrasound assisted freezing (UF) was developed by Tian et al. (Citation2020). The findings showed that degassing followed by ultrasound assisted freezing (DUF) can improve quality attributes, and freezing rate of frozen radishes with more integrated microstructure. Sample groups treated by pre-degassing for (5 min) at −0.09 MPa, then followed by UF presented the phase transition rate and freezing rate increased by 29.8% and 28.8%, respectively. Thus, DUF have great potentials in rapid freezing of porous foodstuffs.
Foaming and defoaming
The principle of ultrasound-induced foaming is the dispersion of gas bubbles. Ultrasound can improve the foaming properties due to decreasing the particle size, solubility, and increased stability of food proteins (Higuera-Barraza et al. Citation2016). The serum proteins are sonicated at 20 kHz, and it was found that improving the functional properties like the foaming solubility and capacity owing to exposure to increased temperatures (Bhargava et al. Citation2021). Xiong et al. (Citation2018) informed that the enhanced foam stability interaction of pea proteins after sonication promotes and forms a smooth film at the air-water interface. Also, foam capacity and stability improved after sonication was detected in soy protein at low-power probe sonication. However, ultrasonic bath treatment (500 kHz) did not significantly improve the foaming characteristics (Sheng et al. Citation2018). Ultrasound-treated soy protein dispersions at a high temperature (75–80 °C) enhanced the foaming properties, probably due to modifying the particle size of proteins. A synergetic effect of ultrasound and temperature was pragmatic on foam stability (Morales et al. Citation2015). The foaming capacity and stability of sonicated wheat protein gradually increased with the increase in ultrasound power (Bhargava et al. Citation2021). Some applications, that is, (cooking, cutting, foaming, separation, and degassing) of power ultrasound technology for innovative food processing are presented in (Bhargava et al. Citation2021; Chemat, Khan, and Zill-e-Huma Citation2011; Gonzalez-Gonzalez et al. Citation2020; Liu, Wang, and Zhang Citation2020; Naji et al. Citation2020; Singla and Sit Citation2021; Su et al. Citation2018).
Table 3. Several applications of ultrasound for innovative various food processing.
Modify the structural and functional properties of food
HIU-induced changes in proteins
Green processing technologies have been used to modify food ingredients to obtain different functionalities. This technology has recently generated interest in the food industry due to its promise to develop novel and targeted processes to enhance the safety and quality of processed foods. To achieve this objective, ultrasound technology has emerged as an effective technique. This process has been used for many years in the case of proteins to change their structure-functional properties. The acoustic cavitation can cause changes in the native structure of proteins which are desirable for protein functionality (Higuera-Barraza et al. Citation2016). Both bath and probe types of ultrasound devices can be used to treat protein isolates. Zhou et al. (Citation2016) used the probe type sonication at different power levels (200, 400, and 600 W for 15 and 30 min). They found that emulsifying stability index, emulsifying activity index, solubility and sulfhydryl groups increased after ultrasound treatment. Flores-Jiménez et al. (Citation2019) found that sonication (40 kHz for 15 min and 30 min) improved the functional properties of canola (Brassica napus) protein isolates. It was also found that ultrasound can improve the foaming properties of faba (Vicia faba L.) beans (Martínez-Velasco et al. Citation2018). Moreover, the particle sizes of pea and SPI s reduced after ultrasound treatment, mainly due to the shear forces generated from acoustic cavitations (O'Sullivan, Park, and Beevers Citation2016). Moreover, the emulsifying properties and solubility of poorly soluble soy and pea protein isolate were significantly enhanced after sonication. O'Sullivan, Park, and Beevers (Citation2016) investigated the effects of ultrasound (34 W cm−2 for 2 min) on the emulsifying and physicochemical properties of soy and wheat protein isolates. The study results indicated that ultrasound could improve the emulsifying properties and solubility of protein isolates. A probe-type sonicator was used to treat pea protrin isolate and the physicochemical and functional properties were studied (Xiong et al. Citation2018). The results showed that ultrasound reduced the particle sizes and increased the number of exposed hydrophobic groups. This indicates the unfolding of protein molecules, improving the functional properties, particularly foaming properties. In another study, sonication resulted in higher adsorption dynamics at the air-water interface, overrun, solubility, and improved foaming properties of faba bean protein isolates (Martínez-Velasco et al. Citation2018). The effects of ultrasound on the thermal properties and structural changes of whey protein concentrate (WPC) solutions were studied (Chandrapala et al. Citation2011). The denaturation enthalpy of WPC decreased when sonicated for up to 5 min. Longer sonication increased the denaturation enthalpy because of protein aggregation. Arzeni et al. (Citation2012) investigated the sonication effects on the emulsifying properties, gelation, foaming and thermal aggregation of egg white (EW) proteins. They concluded that no significant change in total sulfhydryl (SH) content, while an increased surface hydrophobicity was observed. The decrease in the apparent viscosity of sonicated protein samples resulted in improved foaming stability. Moreover, the stability (against creaming and flocculation) of emulsions stabilized by sonicated proteins was higher than those stabilized by native proteins. Ultrasound treatment did not significantly change the gelation temperature of EW. The rate of aggregate formation after heating was accelerated by sonication due to increased protein hydrophobicity. Our previous studies investigated the effects of ultrasound (20 kHz) at power ranging from 200 to 600 W for 15–30 min on the structural and technofunctional properties of SPI dispersions (Hu, Li-Chan, et al. Citation2013; Hu, Fan, et al. Citation2013; Hu, Wu, et al. Citation2013). It was reported that ultrasonic treatment formed more viscous dispersions (fluid character) and reduced both the storage modulus and loss modulus of SPI dispersions. Larger aggregates were observed in ultrasound-treated SPI compared to untreated SPI after observing the lyophilized samples via scanning electron microscopy. However, ultrasonic treatment increased the protein solubility, surface hydrophobicity, and free sulfhydryl content of SPI dispersions. Circular dichroism results showed that low-power treatment decreased the α-helix and random coil content in SPI, while lower β-sheet and higher α-helix were observed with high-power ultrasound (600 W).
Carbohydrates: ultrasound processing of starches
Currently, starch is increasingly finding tremendous applications in the food processing, cosmetic, and pharmaceutical industries because it is ubiquitous, inexpensive, biodegradable, biocompatible, nontoxic, and possesses other important physicochemical and functional properties (Chen et al. Citation2020). The polymeric form of starch contains straight-chain amylose (≈ 30%), connected by α-1,4-glycosidic bond, and branched-chain amylopectin (≈ 70%), which is connected by α-1,6-glycosidic bond (Zhong et al. Citation2020). Although starch possesses several properties, practical and industrial application demands starch with excellent physicochemical and techno-functional aspects. Native starch obtained from different plant sources is usually low in physico-functional properties and is also associated with problems like syneresis, retrogradation, and poor thermal and shear stability. In order to overcome these problems, different approaches, like chemical, enzymatic, genetic, and physical, are used to enhance the techno-functional characteristics of native starches. Still, on the other hand, chemical modification techniques conflict with the increasing consumer demand for clean labels and minimally processed foods. Enzymatic and genetic approaches demand sophisticated and pure enzymes for imparting desirable functional properties. The high cost of the enzymes coupled with sophisticated equipment further increases the overall process cost. Physical modification approaches like ultrasonication are gaining importance due to their accuracy, non-chemical, non-thermal, and eco-friendly nature. The basic principle of ultrasonication involves cavitation and shear forces, which is a series of dynamic processes occurring in the liquid when exposed to ultrasonic treatment. Due to this reason, it produces three kinds of mechanical forces, viz; ultra-high local pressure, high-speed jet and high-frequency vibration (Thanh Nguyen et al. Citation2017). It is proven that ultrasound is an effective modification approach, and the influence mechanism is expected to be explained using mechanochemical theory. This theory works on the principle of conversion of mechanical force into chemical energy. This phenomenon occurs in three stages: the stress stage, the aggregation stage, and the agglomeration stage. Ultrasound is an effective technique that could produce a significant mechanochemical effect on starch granules, which can impart desirable characteristics to the structure and functional properties of starch molecules. Controlled modification by ultrasound technology has successfully been employed to modify the functional, structural, morphological, and physicochemical properties of starches obtained from different plant sources. Sonoprocessing can change material properties, such as physical disruption and acceleration of chemical reactions and the formation of intense cavitation (McClements Citation1995). Previously reported studies have suggested that ultrasound is an effective tool to modify the starches obtained from different sources like rice, oat (Falsafi et al. Citation2019), cassava (Monroy, Rivero, and García Citation2018) and maize (Hu et al. Citation2015).
Recent studies reported that ultrasonication increases the water solubility, alter the pasting viscosity, gelatinization, retrogradation, and swelling parameters, clearly showing that the ultrasonication effect is dependent on numerous factors, that is, type and structure of the starch, temperature and time of ultrasonication process, settings of ultrasonication, intensity, frequency, water percentage in the starch, and composition of the gas in the atmosphere (Zhu Citation2015; Cui and Zhu Citation2021). This method improved the biological activities and physicochemical characteristics of starch for wide potential in food industries. Thus, the effectiveness of ultrasound technology mainly depends upon the process conditions and the type of material to be sonicated. enlists some typical examples of the application of ultrasound for the modification of different types of starches.
Table 4. The application of ultrasound for modification of different types of starches.
Lipids
Lipids play an essential role in the quality of food products as they impart mouthfeel, texture, and flavor. Lipids should possess optimum physical, chemical, and nutritional properties for their dedicated end-use. Among the physical properties, thermal properties are the most important ones associated with crystallization and melting. Lipids observe polymorphism which indicates that lipids will have a mixture of solids and liquids. But for best quality lipids, a single-phase lipid is most desirable. For example, salad oil should not contain components that will solidify during refrigerated conditions, and most cooking oils should be free from any solid components. Crystal size and state of lipids will influence their application in the food industry. Lipids with small crystals with an average value below 50 μm are favorable for spreads or margarine, while hard and brittle fats are used (Wagh, Birkin, and Martini Citation2016). To have a lipid with optimized functional properties, lipids can be modified using different techniques that will affect lipids’ crystallization behavior and, ultimately, their functional properties. Among the different techniques applied to modify lipids, ultrasound technology has proven to be an efficient one.
Different authors have used ultrasound techniques to modify the lipid for the specific target application in the food system. Suzuki et al. (Citation2010) reported that HIU treatment of anhydrous milk fat, palm kernel oil, and an all-purpose shortening enhanced their functional properties. It was observed that primary and secondary nucleation was induced in lipids after ultrasound treatment, generating smaller crystals, and resulting in harder material formation. It was also observed that hardness was more efficiently influenced by HIU when applied at higher crystallization temperatures (26 and 28 °C). Further, steeper and sharper melting profiles were observed in the network of anhydrous milk fat and all-purpose shortening after sonication. According to da Silva, Danthine, and Martini (Citation2021), secondary nucleation is induced by sonication either by making more nucleation or forming smaller nuclei, which results in a more uniform crystal network, more elastic harder texture, and is capable of entrapping more oil. In their study, palm-based fats were crystallized using ultrasound techniques. It was observed that physical properties like melting behavior, oil binding capacity, hardness, and viscoelastic properties were all improved. In the study of Abesinghe et al. (Citation2020) on buffalo milk fat globules, it was observed that sonication reduced the size of the globules of buffalo milk fats to sub-micron level with better stability and improved gel strength. They reported that the average volume-weighted mean diameter of milk fats globules was reduced by 93%, and surface area was increased by 8.5%. It was also observed that the gel strength of sonicated milk was increased by 98%, along with improved stability. Further, in addition to functional properties, sonication influenced the nutritional properties of milk fat globules by increasing the free saturated fatty acids.
Crystallization is an important factor governing the food structure, texture, and consumer appeal. Ultrasonication facilitates the crystallization process by modulating crystal size and morphology distribution, which results in the production of crystals with desired properties (Deora et al. Citation2013). Lipids in cocoa butter can crystalize in six polymorphic crystal forms (I–IV). Form V is considered the most stable among all these six forms and has a melting point of 34–35 °C (Beckett Citation2009). Well-tempered chocolates form V are most desirable because they impart resistance to fat bloom and good visual sensation (Beckett Citation2008). In the study of Higaki et al. (Citation2001), tripalmitoylglycerol and cocoa butter were ultrasonicated (20 kHz, 100–300 W for 3 s), and it was observed that the stable form V was directly crystallized out. However, in the normal tempering process of cocoa butter, unstable forms III and IV are crystallized in the first cooling process and later during the reheating process. These unstable forms are converted to the stable form V (Deora et al. Citation2013). This indicates that an ultrasonication technique can obtain a crystal structure, resulting in a desirable texture and mouthfeel of food products.
Facilitate beneficial food reactions
Glycosylation (Maillard reaction)
Several studies have found effective glycosylation processes enhanced by ultrasound using various carbohydrates and plant protein isolates. Examples include dextran and buckwheat protein (Xue et al. Citation2017), β-Glucan and naked oat protein (Lin et al. Citation2020), glucose and casein (Bi et al. Citation2017), glucose and black bean (Jin et al. Citation2019), gum acacia and whey protein (Chen et al. Citation2019), sweet potato protein hydrolysates (Habinshuti, Mu, and Zhang Citation2021) and glucose and ovotransferrin (Zhang et al. Citation2021). Most of these studies assessed the improvement of the Maillard reactions rate, structural changes, and emulsifying properties of the conjugates. Compared to classical heating, improved emulsifying properties were found for ultrasound-indued Maillard reactions (Xue et al. Citation2017; Chen et al. Citation2019), with a lower browning rate (Chen et al. Citation2019). Ultrasound pretreatment before heating Maillard reactions was also found to enhance the reaction rate and emulsifying properties of the conjugates (Bi et al. Citation2017; Jin et al. Citation2019). Moreover, the glycation of food proteins through ultrasound-assisted Maillard reaction (USMR) promotes their techno-functional properties. Also, several positive characteristics brought by USMR, that is, improved sensorial attributes like (desirable flavor, enhanced color, and decreased bitterness), enhanced antioxidant power and inhibited hazardous elements formed in the processed food, including acrylamide, and advanced glycation final-products (Yu et al. Citation2020).
After using ultrasound-assisted enzymatic treatments, Maillard reaction products of potato protein hydrolysates increased aroma and antioxidant contents (Habinshuti, Mu, and Zhang Citation2021). Furthermore, the antibacterial activity and browning rate of ovotransferrin improved by ultrasound-assisted glycosylation with glucose, reducing free amino groups and a more flexible protein tertiary structure (Zhang et al. Citation2021). After ultrasound treatment, the protein unfolding and the exposure of hydrophobic groups to the protein surface could improve the emulsifying properties of SPI-pectin conjugates. Moreover, reducing the particle sizes of proteins and the release of some free amino acids into the medium could facilitate the formation of glycosidic bonds between amino groups of amino acids and carboxyl groups of polysaccharides (Ma et al. Citation2020; Wang et al. Citation2021).
Enzymatic cross-linking and protein hydrolyzation
Ultrasound has also been tested in conjunction with enzymes to enhance enzymatic cross-linking. Approximately 20 kHz and 41–45 W cm−2 for different durations combined with heat pretreatment resulted in a larger molecular size, highest intrinsic fluorescence capacity and loss of free amino groups. HIU (∼69 W cm−2) pretreatment for transglutaminase-linking of whey protein was also found to significantly increase gel strength, viscosity, and WHC. This may be attributed to the increased cross-linking (Ahmadi, Razavi, and Varidi Citation2017).
Ultrasound pretreatment alone or combined with transglutaminase before hydrolysis can also produce yeast milk and whey gluten mixtures with a salty and umami taste. Here, the ultrasound helped to embed yeasts in the wheat gluten, increased hydrolysis degree owing to higher amino acid cleaving, producing molecules fractions with a molecular weight below 3 kDa. A 56% of the proteins had a molecular weight below one kDa (Su et al. Citation2021). However, no significant changes in the conformational structure of the lactalbumin-ferulic acid conjugates were observed (Yuan et al. Citation2018).
Jin et al. (Citation2015) studied the effects of ultrasound on the enzymolysis of corn gluten meal and the structures of zein and glutelin fractions. They found that ultrasound pretreatments enhanced the hydrolysis degree, unfolded glutelin and zein, increased β-sheet and decreased the α-helix of glutelin (Jin et al. Citation2015). Similarly, Dabbour et al. (Citation2019) investigated the effects of HIU on the conformational and functional properties of sunflower protein meal and its hydrolysates. The results showed that sonication pretreatment prior to hydrolysis enhanced the emulsifying, foaming properties as well as the solubility of proteins. Moreover, HIU improved the ABTS radical scavenging capacity and superoxide of proteins (Dabbour et al. Citation2019).
Xu et al. (Citation2020) studied the impact of multi-frequency power ultrasound (MF-PU) on the degree of hydrolysis and mechanism of casein during alcalase enzymolysis and reported that MF-PU in three frequency values (20/40/60 kHz) model dramatically enhanced the degree of hydrolysis of casein. Therefore, MF-PU could be utilized as an effective pretreatment approach to elevate the casein enzymolysis.
Fermentation
In fermentation, power ultrasound at frequencies of 20–50 kHz has been employed for different purposes, such as improving mass transfer and cell permeability or eliminating undesired microorganisms (Ojha et al. Citation2017; Akdeniz and Akalın Citation2019). For the latter use case, acoustic cavitation through power ultrasound is used to disrupt living cells in microbiology. It is also under investigation to disinfect water by the same principle, supplementing oxidation processes and working on its own at sufficient intensities (Gracin et al. Citation2015). Power ultrasound can also benefit microorganisms at lower intensities (meaning lower exposure durations, frequencies, acoustic power, or a combination of the three). Such process concepts are sometimes called “sonobioreactors” (Chisti Citation2003), where the fermentation tank is subjected to ultrasonic irradiation (Yang et al. Citation2020). The mechanism by which low-intensity ultrasound enhances fermentation is not entirely clear; however, it has been postulated that the collapse of microbubbles on the cell wall causes microjets which weaken the cell wall, increase its permeability, and activate calcium channels (Avhad and Rathod Citation2014; Pawar and Rathod Citation2020). The increased transfer of materials across the cell membrane, including substrates and gene material, is called sonoporation. Activation of enzymes is another observed effect (Ojha et al. Citation2017).
In the case of a study on apple cider fermentation using power ultrasound, the biomass growth rate was increased 10-fold, and the final concentration by 63% when treated in 0.5 s pulses every 6 seconds. On the other hand, ethanol productivity decreased. Only treating during the first 12 h of the growth phase was found to have the highest benefit (Al Daccache et al. Citation2020). Another study found inhibitory effects on glucose to ethanol fermentation at different direct (23 and 32 W L−1) and indirect (1.4 W L−1) ultrasound intensities. That study aimed at biofuel production. The results did not support the theory of increased mass transfer under ultrasonic treatment. The authors mentioned no mass transfer limitation on glucose, CO2, and ethanol and thus no beneficial effect of enhanced mass transfer as a reason for the ineffective ultrasound treatment in their study (Huezo, Shah, and M Citation2019).
The application of power ultrasound in wine fermentation has also been studied (Ojha et al. Citation2017). In recent studies, it has been shown to reduce contamination by Brettanomyces yeasts and lactic acid bacteria by 89.1–99.7% and 71.8–99.3%, respectively, when applying 24 kHz at 400 W in continuous flow treatment (0.25–1 L min−1). However, the ultrasonic treatment also impaired sensory properties (Gracin et al. Citation2015).
For yogurt fermentation using Lactobacillus paracasei, an increase in peptide content of 64.23% has been observed when applying 100 W L−1 of 28 kHz ultrasound in 10 s pulses every 100 s starting 9 h after the start of fermentation. This was attributed to the increased enzymatic activity of extracellular proteases (Huang et al. Citation2019). In combination with microwave treatment, ultrasonicated milk fermentation with Lactobacillus acidophilus exhibited higher antioxidant and anticancer activities (Gholamhosseinpour et al. Citation2020). Ultrasound has also been employed experimentally in soybean meal fermentation using Bacillus subtilis. That study found optimum conditions at 0.08 W mL−1 for 1 h treatment time and a temperature of 36.7 °C. In experiments, these conditions increased angiotensin-converting enzyme (ACE) inhibitory activity by 26.4%, peptides content by 36.2%, and biomass by 55.0%. The product of this process also decreased systolic blood pressure by 20.7 ± 1.8 mmHg in rats (Ruan et al. Citation2020).
Marination
In recent years especially for poultry meat, ultrasound-assisted marination has been researched, with tenderization as a common application (Zou et al. Citation2019, Shi et al. Citation2020). It has been stated that the combination of sodium bicarbonate (Zou et al. Citation2019) or potassium alginate (Shi et al. Citation2020) marination and ultrasound lead to conformation changes in actomyosin, creating larger interfibrillar spaces and thus increasing the myofibril fragmentation and reducing filtering residues, cooking loss and shear force (Zou et al. Citation2019, Shi et al. Citation2020). This might be an option for old chicken breast meat and reduce cooking time (Shi et al. Citation2020). Furthermore, ultrasound can increase the sodium uptake of meat such as chicken breast, reducing the amount of sodium that needs to be applied and the necessary marination time. Frequencies from 25 to 130 kHz and treatment times of 1–6 h were tested, but no significant correlation was found except for sodium uptake and treatment time at 130 kHz. At the same time, the treatment decreases lipid oxidation and does not significantly impact the quality of chicken meat (Inguglia et al. Citation2019).
Other types of meat and marination have also been tested with power ultrasound, including acid marination of rabbit meat (Gómez-Salazar et al. Citation2018), commercial marination brine on pork (Contreras-Lopez et al. Citation2020) or beef (González-González et al. Citation2017) with 5′-monophosphate marination also tested on beef (Zou et al. Citation2019). When ultrasound was applied, all types of meat exhibited more tenderness, whether it was assessed by myofibrillar fragmentation (Zou et al. Citation2019) or sensory panel (Contreras-Lopez et al. Citation2020). Mass transfer of, e.g. salt, was also generally higher with ultrasound (González-González et al. Citation2017; Gómez-Salazar et al. Citation2018; Contreras-Lopez et al. Citation2020). However, one study did not find the general acceptability to be increased (González-González et al. Citation2017). In terms of color ultrasound application may decrease the saturation (Contreras-Lopez et al. Citation2020), while another study found the color lighter and shifted toward red (Gómez-Salazar et al. Citation2018).
Food safety and food preservation
Inactivation of microbes (contain biofilms) and pasteurization
Conventional thermal sterilization and pasteurization methods are the most commonly used microbial inactivation technique in the food industry. However, these thermal treatments could significantly affect the nutritional and sensory qualities of food. Due to the increasing consumer demand for fresh and nutritious food, an alternative processing method with a reduced impact on food quality has become necessary. Ultrasound sonication alone or in combination with heat (thermosonication), pressure (manosonication), or heat and pressure (manothermosonication) are likely the most energy-efficient and environmentally friendly alternatives for conventional thermal treatments. High-power ultrasound at lower frequencies is capable of causing cavitation and has a wide spectrum of applications, such as the inactivation of microbes. Acoustic cavitation occurs in a fluid medium during the sonication process due to longitudinal waves with alternating compression and expansion zones. This will result in the liquids’ local pressure falling below the saturated vapor pressure. Once this negative pressure falls below the cavitation threshold (minimum negative pressure needed to form a cavity in liquid), it causes the formation of gas bubbles (Lauterborn and Mettin Citation2015). These gas bubbles in the rarefaction phase will expand from a small gas pocket and grow until it reaches the compression phase causing bubble oscillation (). Acoustic cavitation can be of two types: inertial and non-inertial. Non-inertial cavitation is where the bubbles repeatedly oscillate in a low-amplitude sound field. Whereas, in the case of inertial cavitation, the bubbles will rapidly expand until they cross the energy supplied by the ultrasound. At this point, the energy will not be sufficient to maintain the vapor pressure of the bubble, making it unstable and implode. This will cause repeated growth and collapse of the gas bubbles leading to the production of shock waves that produce regions of high temperature (5500 °C) and pressure (50,000 kPa) (Lauterborn and Mettin Citation2015). In addition, cavitation can also cause high-energy shear waves, causing turbulence. The high pressure, temperature, and turbulence can lead to the decomposition of water molecules, resulting in free radicals (H2O → H + OH*) (Riesz and Kondo Citation1992). These chemical (free radical generation) and physical (pressure and temperature) change created due to acoustic cavitation can act on the bacterial cell wall, disrupting the cell and leading to cell death.
Figure 5. Microbial inactivation of ultrasound. Source: This figure was created with BioRender.com
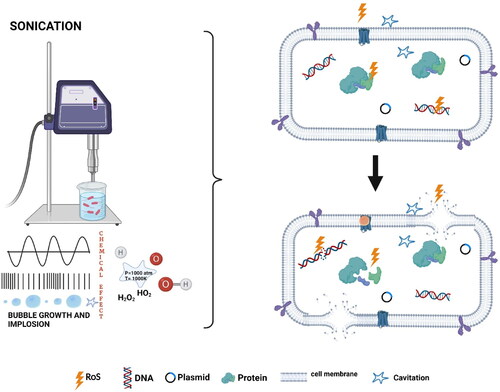
The primary site of action by the ultrasound for microbial inactivation is the bacterial cell wall. The bacterial inactivation involving the cell wall is generally attributed to pore formation or mechanical damage to the cell wall and cell membranes caused by the shock waves during cavitation. The mechanism of bacterial inactivation by ultrasound waves on Escherichia coli, a Gram-negative bacterium and Listeria monocytogenes, a Gram-positive bacterium, was investigated by Gera and Doores (Citation2011). The SEM analysis suggested extensive physical damage to the cell wall and cell membrane as the reason for cell death. Moreover, pore formation was also a reason for cell death, as the pores created on the cell wall are small enough to prevent the passage of small molecules through the cell membrane, thereby significantly affecting the osmotic balance of the cell. The ultrasound treatment was more effective on E. coli than on L. monocytogenes. This higher sensitivity of E. coli toward ultrasound waves was attributed to the difference in its cell wall structure. The gram-positive L. monocytogenes have a thicker peptidoglycan layer in their cell wall than gram-negative E. coli, making the former more resistant to the sonic waves. Similar results where the membrane integrity and metabolomics performance were compromised upon ultrasound treatment were also reported in E. coli and S. aureus (Li et al. Citation2016). The study used flow cytometric analysis with Propidium iodide (PI) and carboxyfluorescein diacetate (cFDA) staining along with transmission electron microscopy (TEM) imaging techniques to evaluate the ultrasound-induced damage to cell structures. The study reported a 98.14% and 91.68% inactivation for E. coli and S. aureus, respectively, after sonication for 20 min at 20 kHz frequency. Moreover, the primary target for the ultrasound on gram-negative bacteria was found to be its outer membrane and gram-positive bacteria to be its cytoplasmic membrane suggesting that the lethal effects of ultrasound to be species depended. He et al. (Citation2021) have reported extensive cell membrane damage, misshapen cells, and leakage of intercellular components of E. coli cells after sonication in a time- and intensity-dependent manner. The E. coli cells were exposed to ultrasound intensities of 64, 191, 372, and 573 W/cm2 for 9, 18, and 27 min and a microbial reduction of 0.76–3.52 log CFU/mL was obtained. Another possibility of microbial inactivation by ultrasound is the free radical mechanism. The cavitation results in the production of free radicals that can inactivate microbes. The primary reactive oxygen species produced by ultrasound is the hydroxyl free radical; the hydroxyl ions thus produced could later react with other cell components of the bacterial cells leading to the production of secondary free radicals resulting in potential damage to the bacteria. Studies using electron paramagnetic resonance (EPR) spectroscopy, a technique used to find free radicals with the help of unpaired electrons, have reported a considerable variation in the spectral peaks of E. coli cells before and after ultrasound treatment (Lin et al. Citation2019). The study identified no prominent peaks for E. coli, but for phosphate buffer saline (PBS) solution, four spectral peaks corresponding to hydroxyl radicals were obtained after sonication. Two additional peaks were reported for a solution containing PBS + E. coli suggesting the formation of secondary free radicals. The formation of these free radicals increases the ROS concentration in the bacterial cell, thereby increasing its intracellular oxidative stress. This can damage the cell membrane, protein and DNA of the cell. A microbial reduction of 98.1% and 81.3% for E. coli and S. aureus, respectively, during ultrasound treatment (20 kHz, 12 min) with damaged DNA and altered enzyme activity was reported by Liao et al. (Citation2018). Furthermore, a dramatic decrease in microbial inactivation efficacy of ultrasound (500 kHz) in the presence of t-butanol- a radical scavenging agent indicates OH free radicals as one of the main reasons for microbial inactivation at high-frequency sonication (Koda et al. Citation2009). A similar observation was reported by Gao et al. (Citation2014) when high-frequency ultrasound (850 kHz) was used to inactivate bacteria and yeast. The study reported 99% inactivation of bacterial cells and a prolonged inactivation even after the ceasing of ultrasound due to the formation of free radicals and H2O2.
Despite this versatility, most published data shows a relatively lower bactericidal activity for ultrasound. The limited antimicrobial effect of ultrasound points out the importance of hurdle technology. Applying ultrasound with other sterilization techniques has improved microbial safety without compromising the nutritional and sensory qualities (Pérez-Andrés et al. Citation2018). A substantial inactivation in Salmonella typhimurium from 0.48 log CFU/g to 2.21 log CFU/g and Campylobacter jejuni from 0.25 log CFU/g to 2.08 log CFU/g were reported when ultrasound treatment was used in combination with peroxyacetic acid (Joo et al. Citation2020). An increase in microbial load reduction from less than 1 log CFU to 6.35, 5.67 and 3.47 log reduction, respectively for S. typhimurium, E. coli and L. monocytogenes were reported in apple juice when sonicated at 40 kHz for 5 min was used in combination with 0.15% fumaric acid (Park and Ha Citation2019). A similar synergistic effect was observed for sodium hypochlorite with ultrasound treatment on inactivating E. coli and L. monocytogenes (Alenyorege et al. Citation2019; Guo, Sun, et al. Citation2020). The study reported a disruption of the cytoplasmic membrane, which accelerated the entry of NaOCl into cells causing changes in protein conformation and leading to cell death. Ultrasound also enhanced the inactivation ability of lysozyme enzyme on S. typhimurium in liquid whole egg (Bi et al. Citation2020). The inactivation efficiency of the ultrasound + lysozyme treatment increased concerning treatment time, temperature, and power. Essential oils such as thyme and oregano have also shown significant synergy with ultrasound in inactivating microbes (Millan-Sango, McElhatton, and Valdramidis Citation2015; Guo, Zhang, et al. Citation2020; He, Guo, et al. Citation2021; He, Liu, et al. Citation2021). As the bactericidal effect of ultrasound is due to cavitation, an increase in temperature can decrease the water vapor tension and thereby modify the cavitation intensity. The increased temperature can also affect the structural changes in the cell and lower its mechanical resistance.
Many recent studies have also explored the possibilities of using mild heat with ultrasound to inactivate microbes (Condón, Mañas, and Cebrián Citation2011; Fan et al. Citation2019; Li et al. Citation2017). The thermosonication has also proved efficient against Bacillus subtilis spores (Fan et al. Citation2019). The thermosonication gave 2.43 log reductions when sonicated at 20 kHz at 80 °C for 40 min. The spore coat, cortex and inner membrane were destroyed, which was not observed during heat treatment or sonication. The study suggested this phenomenon is linked to the formation of liquid jets. Liquid jets are formed as a result of cavitation near the solid-liquid interface. The heat treatment tends to increase the mechanical shear caused by the liquid jets. Additionally, it It also damages the coat proteins causing them to unfold during thermosonication, thereby weakening spore resistance. The synergistic effect of ultrasound is also reported with UV-C and supercritical carbon dioxide (Cappelletti, Ferrentino, and Spilimbergo Citation2014; Ferrentino and Spilimbergo Citation2015; Ortuño et al. Citation2013; Wang, Liu, et al. Citation2020). The application of ultrasound in inactivating microbes in some selected food is described in .
Table 5. Application of ultrasound technology in microbial inactivation of food products.
Microorganisms on wet surfaces can form adherent aggregates and grow into micro-colonies called biofilm. Biofilms can be single or multi-layered and comprise one or more microbial species enclosed in a self-produced polymeric matrix (Bjarnsholt et al. Citation2018). The biofilm development cycle consists of four stages (Tolker-Nielsen Citation2015): (i) initial attachment of planktonic cells to the surfaces, (ii) irreversible attachment and formation of micro-colonies, (iii) biofilm maturation and (iv) dispersal or return to planktonic phase. Biofilms are designed to survive harsh environmental conditions and are well protected against disinfectants, making them a source of recalcitrant contamination, causing food spoilage and public health concerns (Singh et al. Citation2017). Therefore, to develop a proper elimination strategy for biofilms, simultaneous degradation of the protective extracellular polymeric substances (EPS) along with the resident and dispersed cells is necessary (Koo et al. Citation2017). Ultrasound can simultaneously target the EPS and microorganisms, as the microjets and microstreaming could exert enough shear force to disrupt the EPS, and the cavitation and free radicals can target the bacterial population ().
Figure 6. Biofilm inactivation by ultrasound. Source: This figure was created with BioRender.com
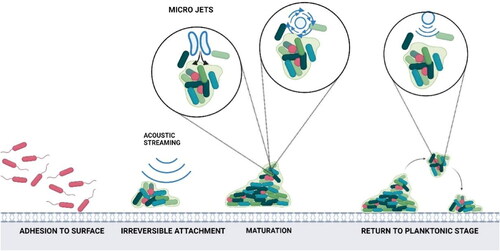
The cleaning efficacy of biofilm by ultrasound contributed to bubbles collapse and physical phenomena such as microjets, cavitation clouds, shock waves, acoustic streaming, micro streaming, and biofilm properties (Vyas et al. Citation2019). Dong et al. (Citation2017) used ultrasound (1 MHz, 5 min) in combination with microbubbles to inactivate Staphylococcus epidermidis biofilms and reported a significant reduction in the biofilm biomass after the combined ultrasound and microbubble treatment. A similar result was reported by Agarwal, Jern Ng, and Liu (Citation2014) on mixed-species biofilm. The 24 h old mixed-species biofilm were treated with ultrasound, microbubbles and a combined ultrasound (42 kHz) + microbubble treatment for 15 min and was analyzed for fixed biomass, extracellular protein and extracellular polysaccharides. The study found the combined treatment to remove 75% of the fixed biomass, while only 10% and 30% removal efficiency was observed for ultrasound and microbubble treatment alone. After the combined treatment, the study also reported a 79% and 72% reduction in the extracellular proteins and polysaccharides. Ultrasound was also used to remove Bacillus cereus biofilm on polyurethane conveyor belts (Fink et al. Citation2017). The cleaning efficacy was measured for ultrasound alone (37 kHz, 30 min) and the combined ultrasound + commercial alkaline sanitizer (10% alcohols, 2.5% benzakolium chloride, and 2.5% didecyldimethyl ammonium chloride). The ultrasound treatment alone had a 21.7% removal efficacy, whereas 60.9% efficacy was observed for the combination treatment. A similar synergistic effect with ultrasound and sodium hypochlorite was observed while removing L. monocytogenes biofilms from stainless steel surfaces (Lee, Kim, and Ha Citation2014). The study used ultrasound at 37 kHz and 200 ppm of sodium hypochlorite for 5 min to obtain a 66.2% reduction. A higher reduction of 85.3% was observed when the treatment time was increased to 100 min. A reduction in the total bacterial count of Cronobacter sakazakii biofilm was also reported by Bang et al. (Citation2017) when ultrasound was used in combination with peroxyacetic acid.
Recently, modeling of inactivation kinetics study was applied successfully to assess decontamination methods in foodstuffs through combining ultrasound with plasma functionalized buffer against E. coli and L. monocytogenes. Ultrasound and plasma functionalized buffers were more effective in reducing the bacterial count in fish samples when compared with individual applications (Esua et al. Citation2022).
Degradation of pesticides and toxins
In agricultural fields, pesticides are sprayed on the fields or directly at the crop to improve yield and quality. Pesticides can be categorized into organophosphorus, organo-chlorine, chlorophenols, carbamates, and pyrethroids based on their chemical structure. Pesticide residue in fresh fruits and vegetables negatively influences human health and natural resources such as land, water, and air (Jankowska, Łozowicka, and Kaczyński Citation2019). Consumers are already becoming concerned about the presence of pesticide residues in fresh produce and commodities. As a result, the food processing industry is looking for technologies that can remove such hazardous chemicals from food materials. Today, researchers are investigating the feasibility of using innovative processing technologies such as ultrasound to reduce the level of pesticides in food products.
Ultrasonic cleaning aims to shatter the adsorption between pesticides and food surfaces, allowing the pesticides to be removed. Moreover, ultrasound can also degrade the pesticides that have adhered to fruits and vegetables. Pesticides are degraded sonolytically through a hydroxylation and oxidation reaction with the H* and HO* free radicals generated by ultrasound. Thus, pyrolysis degrades dissolved volatile, organic, non-polar, or hydrophobic pesticides inside the cavitation cavity, whereas dissolved nonvolatile, polar, or hydrophilic pesticides are decomposed after implosion reaction with extremely reactive hydroxyl radicals. Hydrogen peroxide is produced in several reactions, which aids in the sonolytic destruction (Ashokkumar Citation2011). represents the applications of ultrasound in reducing pesticide residues on fruits and vegetables. Debabrata and Sivakumar recently investigated the mechanism of sonochemical degradation of dicofol, a widely used organochlorine pesticide (Debabrata and Sivakumar Citation2018). The study suggested that due to the semi-volatile and hydrophobic nature of dicofol, the possibility of pyrolysis inside the cavitation bubbles is less. As a result, the interfacial region appears to be the primary site for dicofol degradation. Dicofol degradation can be divided into three distinct steps. At first, dicofol gets adsorbed at the cavitating bubble-liquid interface. Second, the thermal decomposition of dicofol takes place at the interface along with a radical attack on degraded molecules. Finally, pyrolytic degradation of intermediates takes place inside the bubble core. Pesticide degradation can also be enhanced by lowering the pH. Increased degradation in the acidic range can be due to a higher rate of hydroxyl radical production (Patil, Patil, and Gogate Citation2014). Zhang, Xiao, et al. (Citation2010) and Zhang, Zhang, et al. (Citation2010) studied the inactivation mechanism of Chlorpyrifos and Malathion. The study reported that the pesticide inactivation is due to the oxidative desulfurization of Chlorpyrifos into chlorpyrifosoxon and Malathion to Malaoxon induced by the free radical attack on the P = S bonds. At the same time, a complex degradation pathway including hydrolysis of the ester moiety, oxidation, hydroxylation, dehydration, and decarboxylation was proposed for Diazinon (Zhang, Zhang, et al. Citation2010). Zhou et al. (Citation2019) studied the removal mechanism of five different pesticides: difenoconazole, azoxystrobin, thiamethoxam, abamectin, and tebuconazole from grapes and rape surfaces by ultrasound washing. The study concluded that the pesticide residue on the surface of fruits is an adsorption mechanism governed by characters of pesticide molecules and matrix surface. As lignin and cellulose are rich in hydroxyl groups, pesticide molecules have a high chance of forming hydrogen bonds with these hydroxyl groups. Therefore, pesticides have varying adsorption behavior with the fruit surfaces depending on their hydrophilic ability. The study reported a removal rate of 14.7–59.8% for rape and 72.1–100% for grapes, respectively.
Table 6. Application of ultrasound on reducing pesticide residues on fruits and vegetables.
Pesticides have a wide range of chemical properties, with some being fat-soluble and others being water-soluble; this must be considered when choosing a cleaning approach. The efficacy of pesticide residue removal from the surface of fruits and vegetables depends on the ultrasound power, frequency, time, type and concentration of the pesticides and reactor types (Azam et al. Citation2020). Sonochemical reactors of various designs have been utilized in the food industry for several applications. Probe systems (UP), ultrasound baths (UB), and flow systems are the most common ultrasonic reactors. By dosing cherry tomatoes with, thiamethoxam, captan, and metalaxyl, two types of ultrasonic treatments, including a UB at 40 kHz and a UP at 24 kHz, were used to test the effectiveness of ultrasound treatment. The UB treatment were more effective in reducing captan. In contrast, thiamethoxam, and metalaxyl were more effectively inactivated by UP treatment. The study also reported a synergistic effect when ultrasound was combined with low-intensity electrical current (EC). The results show that the most effective conditions were 1400 mA + 40 kHz, 800 MA + 24 kHz, and 1400 mA + 24 kHz, respectively, to reduce the amount of thiamethoxam, captan, and metalaxyl residues (Cengiz et al. Citation2018). The higher efficiency of UP in removing pesticide residue was suggested due to the lower frequency. Low-frequency ultrasound can cause physical effects that improve mass transport and cavitation bubble pulsation. Ultrasound removed 16 pesticide residues, including fungicides and insecticides used in strawberry cultivation. For 1, 2, and 5 minu, 200 g of the fruits were treated with ultrasonic cleaning at 40 kHz. The cavitation bubbles formed by ultrasonication resulted in more efficient elimination of pesticides (ten fungicides and six insecticides) in strawberries, with reduction rates ranging from 45.6% to 91.2% (Lozowicka et al. Citation2016). Ultrasonic washing also effectively reduced the fluopyram and tebuconazole levels in apples (Słowik-Borowiec and Szpyrka Citation2020). The reduction of fluopyram and tebuconazole on apples increased with treatment time. A maximum reduction of 84% and 79% were observed after 15 min, which was much higher than the reduction reported following washing with tap water (56.2% and 57%) and boiling water at 100 °C (72.1% and 78%). Zhang, Xiao, et al. (Citation2010) and Zhang, Zhang, et al. (Citation2010) studied the degradation of chlorpyrifos and malathion in apple juice. They reported that pesticide degradation by ultrasound significantly affected the output power. Maximum degradation of 82.0% and 41.7% were reported for chlorpyrifos and malathion after 120 min of ultrasound treatment at 500 W. The level of degradation is determined by the pesticide’s chemical structure. Chlorpyrifos, for example, is far more susceptible to ultrasonic treatment than Malathion. In addition, studies on the influence of ultrasound on the removal of phorate from apple juice revealed that the degradation followed first-order kinetics. The decomposition of phorate was confirmed by the degradation products phorate-oxon and phorate sulfoxide. Furthermore, sonication had no significant effect on pH, titratable acidity, total soluble solids, and sugar contents of apple juice (Zhang et al. Citation2012). The effects of sonication in reducing the concentration of 5 different organophosphorus pesticides (trichlorfon, chlorpyrifos dimethoate, fenitrothion, and dichlorvos) from cucumber surfaces were investigated by Liang et al. (Citation2012). Pesticide concentrations were reduced due to the cavitation caused by ultrasonic waves. The reduction percentages of 82.9%, 63.0%, 52.2%, 84.4%, and 49.8% were reported, respectively, for trichlorfon, chlorpyrifos dimethoate, fenitrothion, and dichlorvos after 20 min sonication.
The efficacy of ultrasound on pesticide degradation is also studied in aqueous solutions. A 98.96% and 60% degradation of chlorpyrifos and azinphos-methyl was reported by Agarwal et al. (Citation2016) after sonication at 130 kHz, 500 W for 20 min. Application of high-frequency ultrasound at 1.6 MHz for 90 min destroyed 90% of DDT in liquid solution (Thangavadivel et al. Citation2009). The efficiency of ultrasound in reducing organochlorine pesticides was studied by Kida, Ziembowicz, and Koszelnik (Citation2018). The study used a mixed solution containing about 21 organochlorine pesticides, and the mixture was subjected to ultrasound treatment at 20 kHz for 5 min. The pesticide destruction efficiency was 68%, 56.2%, and 49%, respectively, at pH 3, 7, and 11. Similar results were also obtained using sonication to decrease parathion concentration in aqueous solutions. The inactivation rate was governed by first-order kinetics and decreased with increasing pesticide content and decreasing sonication strength (Yao et al. Citation2010). Moreover, increased efficiency in inactivating methyl parathion was reported by Shriwas and Gogate (Citation2011) during the combination treatment of ultrasound with Fenton reagent. Ultrasound degradation of dichlorvos (Schramm and Hua Citation2001), carbofuran (Hua and Pfalzer-Thompson Citation2001), and diazinon (Zhang et al. Citation2011) in aqueous solution is also reported. A significant reduction in pesticide residue was also reported when ultrasound was combined with other technologies such as the Fenton process (Wang and Shih Citation2016), photo Fenton process (Zhang, Hui, et al. Citation2019), ozone (Fan et al. Citation2015), and hydrogen peroxide (Raut-Jadhav et al. Citation2016).
Agricultural commodities such as fruits, vegetables and cereals are an important part of human diet. However, due to poor cultivation and improper storage practices, these items may be tainted with health-threatening pesticides and mycotoxins. Mycotoxins are compounds of low molecular weight which are produced as secondary metabolites by certain fungal strains belonging to Aspergillus, Alternaria, Claviceps, Penicillium, Fusarium, and Stachybotrys. Even though more than 400 toxins have been found in food and feed products but those with substantial economic and health implications include aflatoxins, ochratoxins, patulin, fumonisins, trichothecenes, and zearalenone (Adebo et al. Citation2021). As these toxins are stable, conventional processing methods such as washing with different chemical agents, peeling, heating, etc., do not have a satisfactory effect on reducing these toxins. Moreover, extreme treatment conditions can impair the nutritional and sensory quality of food. Hence, ultrasound technology can be a potential alternative to these traditional processing techniques. A study conducted by Hernández-Falcón et al. (Citation2018) on milk samples showed that thermoultrasound (20 kHz, 15 min, 95% amplitude, 45 °C) could decrease the aflatoxin AFM1 concentration by 52.07% (9.41 ± 0.13 to 4.51 ± 0.17 pg) at the end of 14-day storage study. The degradation of AFM1 due to ultrasound was suggested due to the changes in the molecular structure, such as lactone ring hydrolysis, which lead to the production of nontoxic aflatoxin D1 or due to furan double ring breakage caused by cavitation. The potential of using ultrasound to inactivate mycotoxins such as aflatoxin B1 (AFB1), zearalenone, deoxynivalenol and ochratoxin A (OTA) with respect to ultrasound parameters such as power, duty cycle and time were investigated by Liu et al. (Citation2019). The results showed that increasing the ultrasonic power from 2.2 W/cm3 progressively to 11 W/cm3 for 40 min, has improved the mycotoxin degradation efficiency of ultrasound. In the case of deoxynivalenol and AFB1 the inactivation rate increased from 30.9% to 53.1% and 80.02 to 93.6%, respectively, after treatment. This was due to the increased number of cavitation bubbles and hydroxyl radicals formed because of high ultrasound power. The sonochemical degradation of mycotoxin was also influenced by the sonication time. Increasing the time of sonication from 10 min to 50 min increased the degradation efficacy of ultrasound from 54.7% to 81.2%, 66.4% to 94.2%, 19% to 43.2% and 54.4% to 76.4% for AFB1, zearalenone, deoxynivalenol and OTA, respectively. The degradation was also influenced by the duty cycle, where an increase in percent duty cycle up to 25% increased the pesticide degradation and then declined gradually with further increase. A similar reduction in aflatoxin was also reported by Mortazavia, Sania, and Mohsenib (Citation2015) where an ultrasound frequency of 20 kHz for 10 min was able to give a 41% total reduction of the aflatoxins mixture (AFB1, AFB2, AFG1, and AFG2). A decrease of nearly 85% in AFB1 concentration was reported after 80 min of sonication of an aqueous AFB1 sample with a 550-W power ultrasound at a frequency of 20 kHz (Liu & Bian, 2019). The study also analyzed the reaction by-products formed after ultrasound treatment of AFB1 using UHPLC Q-Orbitrap mass spectrometry and identified eight different molecules corresponding to the AFB1 degradation by-product. Based on this result, two distinct degradation pathways for AFB1 were proposed (). The major reactive oxygen species (ROSs) involved in AFB1 degradation are hydroxyl free radical, hydrogen atom and hydrogen peroxide, which are formed due to water sonolysis. In the first pathway, AFB1 is degraded to a 16-carbon compound with the molecular formula C16H13O7 by losing a methyl group from the CH3O in the benzene side chain. In the next step, the C16H13O7 can either lose methanol in the lactone ring, forming C15H11O5, or it can get hydrated at the 8th and 9th C = C giving rise to C16H13O7. The epoxidation of C = C in C15H11O5 later results in and C15H11O7. In the second pathway, the AFB1 directly undergoes epoxidation on the 8th and 9th C = C, resulting in C17H13O7 this can later undergo hydration at the lactone ring producing C17H15O8. The AFB1 can also produce C14H11O6, which is formed due to the breakage and oxidation of the furofuran ring.
Figure 7. Schematic diagram of aflatoxin inactivation by ultrasound (adapted from Liu et al. Citation2019). Source: This figure was created with BioRender.com
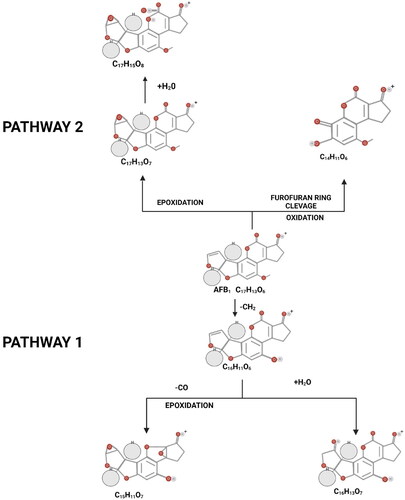
Enzyme inactivation
Enzymes are a specialized class of proteins that act as biological catalysts. The enzyme activity plays a significant role in determining the final quality of the produced food. The presence of endogenous enzymes in food samples can cause deterioration of their flavor, color, and nutrition values. Today, the food industry uses thermal and chemical treatment methods for enzyme inactivation. These traditional treatments can decrease the sensory and nutritional qualities of food, and chemical usage can negatively affect human health. As consumers demand more fresh-like or minimally processed foods, researchers and industries are trying to replace traditional processing methods with emerging non-thermal technologies such as ultrasound. Power ultrasound alone or in combination with other thermal and non-thermal techniques has proved to be efficient in inactivating most food-borne microorganisms (section 2.4.1). The inactivation of both spoilage microbes and enzymes is mainly due to cavitation’s physical and chemical effects. The cavitation contributes to the enzyme inactivation by three main mechanisms: (i) through the high micro-zone temperature and pressure created during cavitation, (ii) due to the free radical formation, and (iii) due to the mechanical and shear forces created via streaming, shock waves and micro-jets. These cavitation effects cause irreversible changes to the conformation of the tertiary protein structure.
The changes in tertiary protein structure will further modify the three-dimensional structure of the enzyme active sites affecting the enzyme–substrate (E–S) interaction (Islam, Zhang, and Adhikari Citation2014). The enzyme inactivation mechanism of ultrasound is shown in . The enzyme inactivation by ultrasound depends on the spatial conformation and amino acid composition of the targeted enzyme. The inhibitory action of ultrasound on trypsin inhibitors was reported due to the conformational changes caused by ultrasound (Huang, Kwok, and Liang Citation2008). The free radicals produced by ultrasound influenced the disulfide bond of Kunitz trypsin inhibitor (KTI), transforming it into a terminal thiol group. This leads to a change in enzyme conformation and inactivation. The peroxidase inactivation by manothermosonication (MTS) was suggested due to splitting prosthetic heme group from the enzyme (Lopez and Burgos Citation1995). Two different mechanisms were suggested as the reason for trypsin inactivation by ultrasound: alternations to molecular confirmations and damage to molecular structures (Tian et al. Citation2004). The alternations of molecular conformations were due to creating a large interfacial area between water and air during cavitation. This disturbed the hydrogen bonds and hydrophobic interactions, which altered the trypsin molecule’s structure. The alternation and damage of trypsin was due to the action of free radicals. When the free radicals react with trypsin, the charge distribution on the protein surface and damage the enzyme’s active sites. This was evident by the presence of fragments of trypsin molecules in the sonicated solution. Furthermore, the addition of sucrose, an additive that prevents the protein from unfolding, does not significantly affect the inactivation, suggesting that protein unfolding is not the major reason behind trypsin inactivation by ultrasound.
Figure 8. Ultrasound inactivation of enzymes via physical (a) and chemical (b) means. Source: This figure was created with BioRender.com
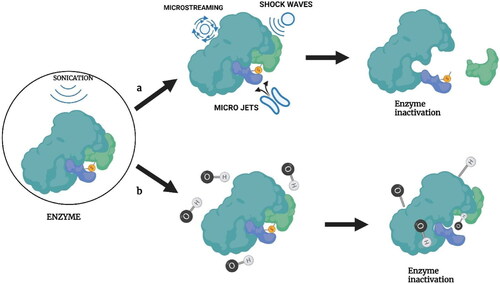
In contrast, the activities of some enzymes are found to increase under mild ultrasound treatment (Delgado-Povedano and Luque de Castro Citation2015). This is because the bulky enzyme aggregates get dispersed under mild ultrasound treatment and break down the large molecular structure, making them more accessible by the substrate. Since the inactivation of enzymes by ultrasound is specific to an individual enzyme, a detailed discussion on the action of ultrasound on food enzymes is necessary. The food enzymes are classified with respect to their relationship to the food quality in flavor-related enzymes, color-related enzymes, and texture-related enzymes. Their reaction to ultrasound treatment is discussed below.
Flavor related enzymes
The major flavor-related enzymes hydrolyze protein, fat, and some low molecular weight precursors such as organo-sulfur compounds (Christen and López-Munguía Citation1994). The important enzymes in this group are lipoxygenase (LOX), lipase, phospholipase, super oxidase dismutase (SOD) and so on. LOX is an oxidative enzyme that catalysis the oxidation of polyunsaturated fatty acids (PUFAs) into peroxide derivatives. These derivatives can later decompose into secondary by-products, giving many fruits and vegetables characteristic flavors. However, excessive oxidation and improper processing conditions can lead to the decomposition of hydroperoxides leading to the development of “beany” off-odors due to the release of volatile aldehydes and alcohols. The numbers of publications investigating the effect of ultrasound on inactivating LOX are limited. Lopez et al. studied the inactivation kinetics of LOX (using a single isozyme) by MTS (López et al. Citation1994). The study observed synergy between heat and ultrasound on inactivating LOX and reported the inactivation kinetics following a first-order reaction. The enzyme inactivation increased with an increase in amplitude (0–104 µm) and temperature (67–76 °C) and decreased with increasing LOX concentration and presence of sugars or polyols (Lopez and Burgos Citation1995). Inactivation of LOX enzyme in whole soy flour by ultrasound was pH-depended (Thakur and Nelson Citation1997). The pH of the media strongly influenced the inactivation of LOX by ultrasound, as the application of ultrasound (20 kHz) for 3 h at pH > 5.0 was found to have no significant effect on the enzyme activity. However, a decrease in enzyme activity by 70–85% under similar treatment conditions was observed when the pH was lowered to 5.0–4.0. This was suggested due to the formation of hydroperoxy radicals (HO2*). As the pKa value of HO2* is around 4.7, the concentration of these free radicals increases at lower pH, thereby favoring the production of H2O2. The hydrogen peroxide thus formed reacts with the LOX leading to enzyme inactivation. At lower pH, the enzyme inactivation was reported to depend on frequency. The LOX activity decreased with increasing frequency at a pH lower than 5.0.
Lipases are enzymes that hydrolyzes lipids into glycerol and free or non-esterified fatty acids. The by-product of lipolysis can degrade sensory qualities due to the production of rancid aroma (butyric acid production) and bitter/soapy taste (Santos et al. Citation2003). Lipase enzyme is widely studied in cereal and dairy products. The lipase enzyme can be either indigenous to milk or from the microbial origin in cow’s milk. The indigenous lipase in cow milk is mainly the lipoprotein lipase that gets activated during the disruption of the fat globules. The bacterial lipase is from psychotropic bacteria, many of which are heat stable and are a significant determinant of product shelf life (Deeth and Fitz-Gerald Citation2009). Vercet, Lopez, and Burgos (Citation1997) studied the effect of MTS on inactivating the heat-resistant lipase from Pseudomonas fluorescens. Ultrasound (20 kHz) was used in combination with high temperature (110–140 °C) and pressure (650 kPa) to inactivate the lipase enzyme. MTS was more effective than heat treatment in inactivating lipase enzymes.
The inactivation efficacy was reduced with increased temperature, but an increase in hydrostatic pressure overcame the loss in efficacy. MTS at 110 °C reduced the residual lipase activity by 0.5%, but in combination with pressure and temperature at 140 °C, the residual activity was reduced to 7%. The MTS inactivation of heat-resistant bacterial lipase is pH-independent (Vercet, Burgos, and Lopez-Buesa Citation2002). In the case of pressure, the lipase inactivation increased with the increase in pressure from 300 to 500 kPa, and no further inactivation was reported when pressure was increased to 600 kPa (Vercet, Burgos, and Lopez-Buesa Citation2002). The inactivation of two different lipase enzymes: phospholipase A2 and porcine pancreatic lipase (PPL), by using MTS was studied by Vercet et al. (Citation2001). The study found MTS efficiency depended strongly on the enzyme. The phospholipase enzyme was almost insensitive to the MTS treatment and required high temperatures for inactivation. This behavior was mainly due to the relatively compact structure and extremely high heat resistance of the enzyme. Phospholipase is a relatively small enzyme (13.927 kDa) stabilized by seven disulfide bonds, making them less vulnerable to shear forces. Moreover, phospholipase has two calcium-binding sites (Ca2+ is a cofactor for phospholipase), and calcium binding stabilizes the enzyme against thermal inactivation. In contrast, PPL was highly sensitive to heat and MTS because of its higher molecular size (43 kDa) and its extended shape. As the PPL contains two different domains with a length almost double its diameter, it experiences higher shear force than phospholipase.
Color related enzymes
The major pigments responsible for the perceived color in food products are carotenoids, chlorophylls, anthocyanins, betalains, and myoglobin. Degradation of any of these components in food can lead to a significant loss in color quality. Polyphenol oxidase (PPO) is one of the oxidoreductase enzymes responsible for the browning reaction in fruits and vegetables. The enzyme is also called as phenolase, catecholase and o-diphenol oxidase. PPO has a dual catalytic function and oxidizes monohydroxy phenols to o-dihydroxy phenols and subsequently dehydrogenates the o-dihydroxy phenols into corresponding o-quinones. The polymerization of quinones will then result in the formation of brown, black- or reddish- pigments causing enzymatic browning. Investigations of the inactivation mechanism of PPO by ultrasonic treatments (200 W for 5, 10, 15, 20, and 30 min) exhibited that ultrasound changes the conformation of the PPO enzyme leading to aggregation after sonication (Iqbal et al. Citation2020). The particle size distribution study of PPO showed a gradual increase in particle size diameter from 140–341 nm with the increase in treatment time, suggesting the disruption of inter and intramolecular linkages of the PPO enzyme. The huge shear force produced by the ultrasound causes fragmentation of the protein structure, leading to aggregation and subsequent reduction of PPO activity. Even though ultrasound did not affect the primary structure of the PPO enzyme, a significant loss in the α-helix followed by a reorganization of the secondary structure and a disruption of the tertiary structure were identified by circular dichroism and fluorescent spectroscopic methods, respectively. The reduction of enzyme activity was reported to increase with the increase in treatment time and intensity, with a maximum reduction of 65% reported after 20 min and 400 W treatment. A similar decrease in residual PPO activity in bayberry juice with respect to increasing temperature, intensity and treatment time was also reported (Cao et al. Citation2018). The PPO activity in bayberry juice was reduced by 53.23% and 12.84% after 10 min of ultrasound treatment at 90 W/cm2 and 181 W/cm2. The inactivation followed the first-order kinetics with a complete inactivation of PPO observed at 452 W/cm2.
Furthermore, cooling samples in an ice bath to separate the effect of temperature and cavitation has significantly decreased the inactivation efficiency by ultrasound. Cervantes-Elizarrarás et al. (Citation2017) optimized the treatment conditions for thermal ultrasound conditions of blackberry juice by using response surface methodology to maximize the enzyme and microbial inactivation without significantly affecting the physicochemical properties of the juice. The obtained response variable had a correlation coefficient (R2) of 0.91 with the mathematical model. The optimized conditions of 50 ± 1 °C at 17 ± 1 min showed higher enzyme inactivation and higher antioxidant capacity than conventional pasteurization treatment. Thermosonication was also found to be more effective than traditional pasteurization methods in inactivating PPO in soursop nectar. Thermosonication (24 KHz at 54 °C for 10 min) gave a 99% reduction on PPO activity whereas only 81% decrease was obtained by traditional pasteurization treatment (65 °C for 30 min). A significant decrease in enzyme activity by ultrasound was also reported in raspberry and blueberry puree (Medina-Meza, Boioli, and Barbosa-Cánovas Citation2016), mushroom (Cheng, Zhang, and Adhikari Citation2013), melon juice (Fonteles et al. Citation2012), and pineapple juice (Costa et al. Citation2013).
Peroxidase (POD) is an oxidoreductase enzyme that oxidizes the phenolic compounds in fruits and vegetables. It also reduces hydrogen and other hydro-peroxides in water. Depending upon the catalytic center, POD can be classified as heme and non-heme POD. The enzyme can exist in multiple isoforms, making them capable of acting on various substrates. POD is found in most fruits and vegetables and is associated with the development of brown pigments and off-odors. POD can remain active even at temperatures as low as −18 °C (Robinson Citation1991), therefore, it is necessary to blanch fruits and vegetables before refrigeration. Guo et al. (Citation2021) studied the potential mechanism behind ultrasound-assisted and thermosonication-assisted blanching of carrot peroxidase at varying frequencies. The ultrasound-assisted blanching (residual activity = 82.40%) showed a lesser inactivation effect on carrot POD as compared to traditional blanching (residual activity = 64.97%). Whereas thermosonication-assisted blanching (60 °C for 12 min at 40 kHz) significantly decreased the POD activity to 34.83%. The study also reported a synergistic effect when more than one frequency was coupled together. The synergy was due to the formation of new microbubble clusters under two different ultrasonic wavelengths. This can exert stronger mechanical and shear forces on the POD molecule leading to the disruption of Van der Waals and hydrogen bonds of the apo-enzyme. Ultrasound is also reported to affect the binding capacity of the enzyme by causing degradation to the hematin group/active site of POD.
Furthermore, the presence of ultrasound-induced free radicals (hydrogen, hydroxyl, and alkyl free radical) was confirmed by EPR spectroscopy and were reported to involve in POD inactivation. The effect of different ultrasound frequencies on inactivating commercial horseradish peroxidase was studied by Tsikrika et al. (Citation2018). The study reported an enhanced inactivation potential by ultrasound with increased power and sonication time for each frequency range. Even though ultrasound at low frequency and power combination were ineffective in inactivating POD, a complete inactivation after 60 min was achieved using 378 kHz and 583 kHz (at 48 W) and 114 kHz (49 W) frequency-power combinations. Kulmann de Medeiros et al. (Citation2021) investigated the effect of sonication parameters such as pulse regime, processing time and intensity on the inactivation of POD in sugar cane juice. They reported that the pulse regime had no significant effect on the POD inactivation kinetics. Therefore, considering the lower energy requirement, 20/10 s on/off pulse regime for 25 min and 75% power intensity was considered as the best condition for POD inactivation (77.3% reduction). Few authors have explored the effect of pulse regime on POD inactivation and reported ambiguous results. Kulmann de Medeiros et al. (Citation2021) reported an increase in POD inactivation in green beans using a pulse regime. The residual enzyme activity decreased with the increase of in duty cycle to 0.7 s. Whereas, reduced efficiency in enzyme inactivation by pulse mode as compared to continuous mode was reported by Illera et al. (Citation2018) during POD inactivation in apple juice. Increased efficiency of thermosonication over conventional heat treatment on POD inactivation has been reported by few authors. Saeeduddin et al. (Citation2015) compared the inactivation potential of POD in pear juice by conventional and thermosonication methods. Analyzing the two processes at similar temperatures (60 °C), thermosonication gave a significantly lower residual activity (4.3%) than conventional thermal treatment (66%). Complete inactivation of POD by thermosonication after 1 min versus 25% residual activity after conventional blanching was reported by Cruz, Vieira, and Silva (Citation2006) in watercress. Similarly, thermosonication at 90 °C for 1 min resulted in a residual activity of 4% compared to 17% for conventional blanching at 90 °C for 1 min in guava fruits (Ali et al. Citation2011).
Texture related enzymes
Pectic substances are complex polymers present in the plant cell wall and account for about 0.5–4% of the fresh weight. They are made up of a galacturonic acid backbone with α-1-4 linkages and consist of L-rhamnose, arabinose, galactose and xylose side chains. Pectinases are enzymes that hydrolyze pectin and are widely seen in bacteria, certain fungi and plants. The major pectin lytic enzymes are pectinesteases (PE), protopectinase, polygalacturonase (PG), pectin methylesterase(Arancon Citation2013) and polygalacturonaselyases (PGL)(Kashyap et al. Citation2001). The application of ultrasound has been reported to enhance the inactivation ratio of pectinmethylesterase (PME). PME is one of the major proteolytic enzymes in tomatoes. Thermosonication (20 kHz, 61 °C, 100 W) has increased the enzyme inactivation rate of tomato PME extract by 39-374-fold compared to thermal treatment (Raviyan, Zhang, and Feng Citation2005). However, in the case of tomato juice, only a 2.3- and 2.1-fold decrease in D-value as compared to thermal treatment was reported after thermosonication at 55 °C and 65 °C (Wu et al. Citation2008). Terefe et al. (Citation2009)studied the inactivation kinetics of PME in tomato juice by thermosonication. They reported an increase in the enzyme inactivation rate by six times compared to the thermal inactivation at 60 °C. This change in the inactivation potential of PME juice and extract was suggested due to the variation in viscosity of the treatment media; as tomato juice has a higher viscosity than buffer solution, the cavitation effect will be less pronounced in tomato juice than in buffer solution. Thermosonication was also reported to be a more efficient ultrasound treatment for inactivating PME. Thermosonication of pear juice at (65 °C, 10 min, 700 W) yielded a residual activity of 3% and was more effective than ultrasonication (25 °C) which had a residual activity of 93% (Saeeduddin et al. Citation2015). Similarly, a 7% and 97% residual PME activity was observed in apple juice after thermosonication (60 °C) and ultrasound treatment (20 °C), respectively (Abid et al. Citation2014). Thermosonication (60 °C) also reduced the PME activity in carrot juice to 6% compared to 100% residual enzyme activity after ultrasound treatment at 20 °C (Jabbar et al. Citation2015). Polygalacturonase (PG) is an enzyme that catalyzes the hydrolytic cleavage of α-1,4 glycosidic bonds between galacturonic acid present in the plant cell wall. The action of PG leads to a decrease in viscosity and phase separation in juices. Terefe et al. (Citation2009) investigated the effect of temperature and ultrasound on inactivating PG in tomato juice. Thermal treatment in the range of 50–75 °C was only able to partially inactivate the enzyme due to the presence of thermostable PG isoforms. Tomato PG has three isoforms: PG1, PG2A, and PG2B, of which PG2A and PG2B, are heat-liable, whereas PG1 is heat–stable. The application of ultrasound and heat treatment showed a synergistic effect in inactivating PG. Thermosonication (20 kHz, 75 µm) at 60 °C enhanced the PG enzyme inactivation by 4-fold compared to thermal inactivation alone. Thermal treatment at 70 °C did not alter the activity of PG in tomato juice, but MTS treatment (20 kHz, 200 kPa, 70 °C) decreased the PG activity by 62% (Vercet, Sánchez, et al. Citation2002).
Amylases are hydrolases enzymes present in plants, animals, and some microbes. They cleave O-glycosidic bonds of starch molecules and are classified into endo- and exo-amylases depending on whether the cleavage is random (endo-) or endwise (Alexopoulos et al. Citation2017). Endo-amylases cleave the 1,4- glycosidic bonds, whereas exo-amylase cleave the 1,4- and 1,6- glycosidic bonds in the starch molecule. Ultrasound can both activate and inactivate amylase enzyme depending on its nature (Oliveira et al. Citation2017). Oliveira et al. (Citation2017) used four different types of α-amylases, including one fungal α-amylase (from Aspergillus oryzae (AOA)) and three bacterial α-amylase (two isolated from Bacillus licheniformis: Liquozyme (LQA) and heat-stable (HAS) and one from Bacillus amyloliquefaciens (BAA)) to study the effect of ultrasound treatment. Ultrasound inactivated both BAA and AOA, but in contrast, activated HAS and LQA. The highest activation and inactivation of α-amylase ranged from +1657% for LQA to −44% for BBA. In the case of AOA, maximum inactivation of 22.52% was observed at ultrasound treatment of 40 kHz, at 60 °C and pH 5.5. Ultrasound treatment at 40 kHz, at 45 °C and pH 5.0 decreased the BAA activity by 43.98%. This decrease in enzyme activity was due to the activity of free radicals formed as a result of cavitation. A decrease in enzyme activity in the range of 8%-310% was reported in the case of HAS. This promotion of enzyme activity was suggested due to the formation of hot spots in the sonication media due to cavitation. As amylases are usually activated at temperatures above 80 °C, the formation of hot spots increases the local area temperature and thereby activates the enzyme. Gas content and probe face dimensions were also found to be major factors influencing the inactivation of α-amylase (Kadkhodaee and Povey Citation2008). Degassing of the medium before sonication has been shown to decrease the inactivation efficiency of ultrasound because the dissolved gas entrapped in the liquid medium act as a nucleus for cavitation during ultrasound treatment. Removing the gas from the medium will increase the cavitation threshold, affecting enzyme inactivation. Ultrasound also inactivates α-amylase enzyme in germinated barley at temperatures as low as 30 °C Yaldagard, Mortazavi, and Tabatabaie (Citation2007).
Contributions to environmental protection – food waste disposal
According to FAO data, approximately 33% of the total food produced annually is wasted (Sharma et al. Citation2020). Food waste (FW) is defined as raw or cooked, edible, or inedible parts; produced before, during or after processing, delivery, selling, and home preparation (Torres-León et al. Citation2021). Among FW, oil-bearing crops, plant materials, leaves, and by-products of vegetables and fruits (Gavahian et al. Citation2021; Razola-Díaz et al. Citation2021), or marine by-products are included that consist of non-consumed parts of seafood also (Ali et al. Citation2021). FW contains substantial amounts of bioactive substances that can be valorized and reduce environmental anxieties (Gavahian, Chu, and Mousavi Khaneghah Citation2019). Recovering these valued ingredients from FW by ultrasound attracts extensive attention and will open novel prospects for economic growth to produce a value-added product (Li et al.; Sharma et al. Citation2021). For the mentioned narration, FW is a serious economic and pollution concern that can negatively impact our bioreserves. Thus, we highlight the available studies regarding the impact of ultrasound on environmental protection thru various FW treatments. Moreover, converting FW to biobased materials that may tackle food insecurity offers a healthy diet and its potential capability in the pharmaceutical and food industries.
Recovery of bioactive components from food waste
FW includes but is not limited to vitamins, peptides, antioxidants, polyunsaturated fats, saccharides, pigments, proteins, biosurfactants, enzymes, bioplastics, and biofuels (Georganas et al. Citation2020; Sharma et al. Citation2021; Torres-León et al. Citation2021; Yukesh Kannah et al. Citation2020). Ultrasound is an environmentally friendly technique, an effective mixing, fast energy, reduced extraction temperature, fast response to process extraction control, improved production, and elimination of process steps (Ali et al. Citation2021, Gavahian et al. Citation2021). Ultrasound disturbs the cell walls of FW, enabling the release of bioactive substances. Extraction yield from FW greatly depends on ultrasound frequency (Kumar et al. Citation2017). Ultrasound extraction resulted in a high extraction yield of pectin from FW with a desirable esterification degree. Ultrasound is considered a more effective and simpler technique than the conventional techniques for pectin extraction due to its limits, for example, energy-intensive, low yield, and related to environmental concerns (Gavahian et al. Citation2021).
Ultrasound-recovering bioactive compounds from marine by-products, for example, polysaccharides (chitin), increased the yield and decreased the treatment time due to the cavitation effect (Kjartansson et al. Citation2006). These bioproducts are applied in various industries, including pharmaceutical, biomedical, food, agriculture, and cosmetics (Ali et al. Citation2021). Furthermore, ultrasound increased the carotenoid recovery by 43% from tomato waste (Yukesh Kannah et al. Citation2020); and applied to recover bioactive compounds from winter melon (Benincasa hispida) seeds (Bimakr et al. Citation2013). Water-soluble polysaccharides (β-Glucan) were also extracted from dried and milled by-products from Agaricus bisporus thru ultrasound (Aguiló-Aguayo et al. Citation2017). Some studies stated the possible extraction of phenolic compounds and anthocyanins from the grape peel (Ghafoor, Hui, and Choi Citation2011, Kumar et al. Citation2017). Also, the recovery of bioactive molecules from exhausted olive pomace to detect mannitol and maximize the antioxidant compounds, especially hydroxytyrosol, was studied (Gómez-Cruz et al. Citation2020). Nevertheless, the large-scale industrial application of ultrasound can be challenging owing to limited professional proficiency, expensive, and insufficient data on procedure upscaling (Ali et al. Citation2021, Gavahian et al. Citation2021).
Food industry wastewater disposal
Agrofood industrial wastewater is a major environmental concern due to its negative consequences such as natural water coloring, surface pollution, a serious risk to the aquatic lifecycle (Paraskeva and Diamadopoulos Citation2006), and negative effects on soil and groundwater (Barbera et al. Citation2013). Due to the undesirable effects of the food industry wastewater (FIWW) on environmental safety, ultrasound processing can be used for environmental maintenance by destructing chemical or biological contaminants (Mason and Pétrier Citation2004) or reducing the ratios of hazardous and toxic agents in their permissible limits or altering formulations (Kidak and Ince Citation2006). The ultrasound-assist wastewater degradation mainly depends on ultrasound emitters, the magnitude of the pressure, the frequency of vibrations, the physicochemical characteristics of the materials, the presence of solid particles, and the consumption of oxidizing substances (Sukhatskiy, Znak, and Zin Citation2020).
As an example of FIWW treatment thru ultrasound, olive mill industry wastewater (OMIWW) has been treated with a sonication process at 35 kHz. Ultrasound was shown to be an effective tool for removing several hazardous compounds from wastewater like chemical oxygen demand, total organic carbon, color, total phenol, total aromatic amines, and total fatty acids, providing a cost-effective alternative for destroying detoxifying the refractory compounds in OMIWW (Oztekin and Sponza Citation2021). Also, ultrasound pretreatment improved Fenton-based advanced oxidation as a sustainable treatment scheme to decrease toxicity, total phenol and organic matter from OMIWW (Ciggin et al. Citation2021). So, to reduce the cost of ultrasound wastewater treatment, it is recommended to combine with advanced oxidation process (Mahamuni and Adewuyi Citation2010) with photolysis or ozonation (Sukhatskiy, Znak, and Zin Citation2020).
Food waste fermentation
Ultrasound fermentation technology is becoming popular in the food industry (Zhang, Xiong, et al. Citation2019). Ultrasound-assisted FW fermentation could produce volatile fatty acids (VFAs) through a desirable effect of the sonoelectrochemical treatment on enzymatic hydrolysis and microbial activity (Taheri et al. Citation2021). Similarly, it was found that treated two kinds of FW that contain total solids of 40 and 100 g L−1, for 15 min at an ultrasonic power density of 480 W/L. Ultrasound-assisted FW fermentation dramatically boosted proteins, reducing sugars and chemical oxygen demand; however, it decreased the lipids ratio in FW supernatant. Also, this pretreatment increased the VFAs production significantly during the FW fermentation (Jiang et al. Citation2014). It was concluded that utilizing FW by the chain elongation process and ultrasound fermentation increased caproic and VFAs concentration (Ma et al. Citation2021). Moreover, a novel low-cost strategy and solution designed for cellulase production from Trichoderma reesei with ultrasound -fermentation using spent mushroom as FW substrate (He et al. Citation2021). Ultrasound treatment in the fermentation process could improve the cellulase activity by 24.5% after treatment for 40 min at 0.8 duty cycle after 4 days of fermentation. This work delivers a probable key for mushroom disposal used for cellulase manufacture without pretreatment (He et al. Citation2021).
Limitations and advantages of sonoprocessing for food treatment
summarizes the advantages and disadvantages of sonoprocessing for food treatment. In sonoprocessing, various issues must be resolved before commercial implementation, despite the remarkable academic results from different research groups. Any novel ultrasonic process in the food industry requires equipment producers to customize and build new designs. The design of novel sonication devices to meet the ongoing, large-scale applications is the first and most significant issue (Zhang, Zhu, and Sun Citation2018). Second, to ensure food safety after sonication, toxicological studies are essential since some contaminants could be converted into toxic degradation products (Yao et al. Citation2010). Third, ultrasound treatment may degrade phenolic compounds and vitamins, modify color, and decrease anthocyanin content. Fourthly, some applications have issues with liquids contacting ultrasonic probes. Using a flow-through and non-contact ultrasonic reactors can reduce residence times and help to overcome this issue (Ashokkumar Citation2016). Finally, the effects of ultrasound in food applications are greatly influenced by the treatment conditions such as output power, frequency, treatment time, pH, and temperature (Yuan et al. Citation2021). Thus, finding the appropriate treatment conditions for each application is strongly recommended.
Table 7. Advantages and disadvantages of sonoprocessing for food treatment.
The main advantages of ultrasound treatments are summarized in this paragraph. It was concluded that ultrasound has higher energy efficiency and ultrasonic devices are easy to clean and operate (Taha et al. Citation2020). Sonication has the potential to produce both the physical and chemical effects needed for some processes, such as the polymerization of emulsions. Products produced without external chemical reagents would be purer; thus, ultrasound treatment is considered as a green technology (Chemat, Khan, and Zill-e-Huma Citation2011). The ability to initiate reactions without external reagents is a further advantage. External reagents are unnecessary as collapsing air bubbles produce radicals that could perform redox reactions (Ashokkumar Citation2011). Moreover, the ability of ultrasound to induce mass transfer at both the microscopic and macroscopic scales in chemical reactions is one of its main advantages. Finally, the mechanical effects produced by ultrasound, such as microstreaming, shock waves, and turbulence, increase mass transfer within the medium and may have a positive impact on chemical reactions and other processes even in the absence of the chemical effects of ultrasound (i.e. radical production) (Ashokkumar Citation2016).
Conclusion and recommendations
As an eco-friendly technology, ultrasound has been utilized in many food applications. With the increased interest in using green and energy-efficient techniques, ultrasound devices will be used extensively in the food industry. Many studies investigated the effects and applications of ultrasound in many food and environmental applications. The results showed that ultrasound could be applied in several food applications such as extraction, emulsification, gelation, tenderization, microbial and enzyme inactivation, cutting, tenderization, modification of structural and functional properties of biological macromolecules, freezing, cooking, foaming, food waste valorization and some other applications. The ultrasound approach improved the physicochemical and techno-functional properties of the targeted foodstuffs, increased the shelf life during storage without dramatic effects on the food quality attributes, and other outstanding advantages stated in the current review. Less energy and solvent consumption, easy operation, and less/zero-emission during ultrasound processing are the main advantages of ultrasound application. Recommendations of this study can be summarized in the following points:
The safety of ultrasound devices needs to be fully investigated as metal contamination could occur from the contact between the medium and ultrasound probes.
There is a need to fund research and development projects to develop more effective ultrasound devices in the food industry on a large scale.
Ultrasound probes are easily damaged and need to be changed almost every year; therefore, developing high-quality probes with an extended working period is required.
Disclosure statement
No potential conflict of interest was reported by the authors.
Correction Statement
This article has been corrected with minor changes. These changes do not impact the academic content of the article.
Additional information
Funding
References
- Aadil, R. M., X. A. Zeng, M. S. Wang, Z. W. Liu, Z. Han, Z. H. Zhang, J. Hong, and S. Jabbar. 2015. A potential of ultrasound on minerals, micro-organisms, phenolic compounds and colouring pigments of grapefruit juice. International Journal of Food Science & Technology 50 (5):1144–50. doi: 10.1111/ijfs.12767.
- Abesinghe, A M. N. L., J. K. Vidanarachchi, N. Islam, S. Prakash, K. F. S. T. Silva, B. Bhandari, and M. A. Karim. 2020. Effects of ultrasonication on the physicochemical properties of milk fat globules of Bubalus bubalis (water buffalo) under processing conditions: A comparison with shear-homogenization. Innovative Food Science & Emerging Technologies 59:102237. doi: 10.1016/j.ifset.2019.102237.
- Abid, M., S. Jabbar, B. Hu, M. M. Hashim, T. Wu, S. Lei, M. A. Khan, and X. Zeng. 2014. Thermosonication as a potential quality enhancement technique of apple juice. Ultrasonics Sonochemistry 21 (3):984–90. doi: 10.1016/j.ultsonch.2013.12.003.
- Adebo, O. A., T. Molelekoa, R. Makhuvele, J. A. Adebiyi, A. B. Oyedeji, S. Gbashi, M. A. Adefisoye, O. M. Ogundele, and P. B. Njobeh. 2021. A review on novel non-thermal food processing techniques for mycotoxin reduction. International Journal of Food Science & Technology 56 (1):13–27. doi: 10.1111/ijfs.14734.
- Agarwal, A., W. Jern Ng, and Y. Liu. 2014. Removal of biofilms by intermittent low-intensity ultrasonication triggered bursting of microbubbles. Biofouling 30 (3):359–65. doi: 10.1080/08927014.2013.876624.
- Agarwal, S., I. Tyagi, V. K. Gupta, M. H. Dehghani, A. Bagheri, K. Yetilmezsoy, A. Amrane, B. Heibati, and S. Rodriguez-Couto. 2016. Degradation of azinphos-methyl and chlorpyrifos from aqueous solutions by ultrasound treatment. Journal of Molecular Liquids 221:1237–42. doi: 10.1016/j.molliq.2016.04.076.
- Aguiló-Aguayo, I., J. Walton, I. Viñas, and B. K. Tiwari. 2017. Ultrasound assisted extraction of polysaccharides from mushroom by-products. LWT 77:92–9. doi: 10.1016/j.lwt.2016.11.043.
- Ahmadi, Z., S. M. A. Razavi, and M. Varidi. 2017. Sequential ultrasound and transglutaminase treatments improve functional, rheological, and textural properties of whey protein concentrate. Innovative Food Science & Emerging Technologies 43:207–15. doi: 10.1016/j.ifset.2017.08.013.
- Akdeniz, V., and A. S. Akalın. 2019. New approach for yoghurt and ice cream production: High-intensity ultrasound. Trends in Food Science & Technology 86:392–8. doi: 10.1016/j.tifs.2019.02.046.
- Alenyorege, E. A., H. Ma, I. Ayim, J. H. Aheto, C. Hong, and C. Zhou. 2019. Reduction of Listeria innocua in fresh-cut Chinese cabbage by a combined washing treatment of sweeping frequency ultrasound and sodium hypochlorite. LWT 101:410–8. doi: 10.1016/j.lwt.2018.11.048.
- Alexopoulos, A., S. Plessas, Y. Kourkoutas, C. Stefanis, S. Vavias, C. Voidarou, I. Mantzourani, and E. Bezirtzoglou. 2017. Experimental effect of ozone upon the microbial flora of commercially produced dairy fermented products. International Journal of Food Microbiology 246:5–11. doi: 10.1016/j.ijfoodmicro.2017.01.018.
- Ali, A., S. Wei, Z. Liu, X. Fan, Q. Sun, Q. Xia, S. Liu, J. Hao, and C. Deng. 2021. Non-thermal processing technologies for the recovery of bioactive compounds from marine by-products. LWT 147:111549. doi: 10.1016/j.lwt.2021.111549.
- Ali, G., A. R. Russly, B. Jamilah, O. Azizah, and B. Mandana. 2011. Effect of heat and thermosonication on kinetics of peroxidase inactivation and vitamin C degradation in seedless guava (Psidium guajava L.). International Food Research Journal 18:1289–1294.
- Al Daccache, M., M. Koubaa, D. Salameh, R. G. Maroun, N. Louka, and E. Vorobiev. 2020. Ultrasound-assisted fermentation for cider production from Lebanese apples. Ultrasonics Sonochemistry 63:104952. doi: 10.1016/J.ULTSONCH.2019.104952.
- Al-Juboori, R. A., O. Naji, L. Bowtell, A. Alpatova, S. Soukane, and N. Ghaffour. 2021. Power effect of ultrasonically vibrated spacers in air gap membrane distillation: Theoretical and experimental investigations. Separation and Purification Technology 262:118319. doi: 10.1016/j.seppur.2021.118319.
- Al-Taher, F., Y. Chen, P. Wylie, and J. Cappozzo. 2013. Reduction of pesticide residues in tomatoes and other produce. Journal of Food Protection 76 (3):510–5. doi: 10.4315/0362-028X.JFP-12-240.
- Alves, L., M. Stefanello da Silva, D. R. Martins Flores, D. Rodrigues Athayde, A. Roggia Ruviaro, D. da Silva Brum, V. S. Fagundes Batista, R. de Oliveira Mello, C. Ragagnin de Menezes, P. C. Bastianello Campagnol, et al. 2018. Effect of ultrasound on the physicochemical and microbiological characteristics of Italian salami. Food Research International 106:363–73. doi: 10.1016/j.foodres.2017.12.074.
- Alzate, P., L. Gerschenson, and S. Flores. 2020. Ultrasound application for production of nano-structured particles from esterified starches to retain potassium sorbate. Carbohydrate Polymers 247:116759. doi: 10.1016/J.CARBPOL.2020.116759.
- Amiri, A., P. Sharifian, and N. Soltanizadeh. 2018. Application of ultrasound treatment for improving the physicochemical, functional and rheological properties of myo fi brillar proteins. International Journal of Biological Macromolecules 111:139–47. doi: 10.1016/j.ijbiomac.2017.12.167.
- Amiri, A., P. Sharifian, and N. Soltanizadeh. 2018. Application of ultrasound treatment for improving the physicochemical, functional and rheological properties of myofibrillar proteins. International Journal of Biological Macromolecules 111:139–47. doi: 10.1016/j.ijbiomac.2017.12.167.
- Anwar, M., G. Babu, and A. E. D. Bekhit. 2021. Utilization of ultrasound and pulse electric field for the extraction of water-soluble non-starch polysaccharide from taro (Colocasia esculenta) peel. Innovative Food Science & Emerging Technologies 70:102691. doi: 10.1016/j.ifset.2021.102691.
- Arzeni, C., K. Martínez, P. Zema, A. Arias, O. E. Pérez, and A. M. R. Pilosof. 2012. Comparative study of high intensity ultrasound effects on food proteins functionality. Journal of Food Engineering 108 (3):463–72. doi: 10.1016/j.jfoodeng.2011.08.018.
- Ashokkumar, M. 2011. The characterization of acoustic cavitation bubbles – An overview. Ultrasonics Sonochemistry 18 (4):864–72. doi: 10.1016/j.ultsonch.2010.11.016.
- Ashokkumar, M. 2015. Applications of ultrasound in food and bioprocessing. Ultrasonics Sonochemistry 25:17–23. doi: 10.1016/j.ultsonch.2014.08.012.
- Ashokkumar, M. 2016. Advantages, disadvantages and challenges of ultrasonic technology. In Ultrasonic synthesis of functional materials, 41–2. Springer, Cham. doi: 10.1007/978-3-319-28974-8_3.
- Astráin-Redín, L., S. Ciudad-Hidalgo, J. Raso, S. Condón, G. Cebrián, and I. Álvarez. 2020. Application of high-power ultrasound in the food industry. In Sonochemical reactions, ed. S. Karakuş, 103–26. London, UK: IntechOpen. doi: 10.5772/intechopen.90444.
- Avhad, D. N., and V. K. Rathod. 2014. Ultrasound stimulated production of a fibrinolytic enzyme. Ultrasonics Sonochemistry 21 (1):182–8. doi: 10.1016/J.ULTSONCH.2013.05.013.
- Awad, T. S., H. A. Moharram, O. E. Shaltout, D. Asker, and M. M. Youssef. 2012. Applications of ultrasound in analysis, processing and quality control of food: A review. Food Research International 48 (2):410–27. doi: 10.1016/j.foodres.2012.05.004.
- Azam, S. M. R., H. Ma, B. Xu, S. Devi, M. A. B. Siddique, S. L. Stanley, B. Bhandari, and J. Zhu. 2020. Efficacy of ultrasound treatment in the and removal of pesticide residues from fresh vegetables: A review. Trends in Food Science & Technology 97:417–32. doi: 10.1016/j.tifs.2020.01.028.
- Bang, H. J., S. Y. Park, S. E. Kim, M. Md Furkanur Rahaman, and S. D. Ha. 2017. Synergistic effects of combined ultrasound and peroxyacetic acid treatments against Cronobacter sakazakii biofilms on fresh cucumber. LWT 84:91–8. doi: 10.1016/j.lwt.2017.05.037.
- Bangar, S. P., O. J. Esua, N. Sharma, and R. Thirumdas. 2022. Ultrasound-assisted modification of gelation properties of proteins: A review. Journal of Texture Studies 53 (6):763–74. doi: 10.1111/jtxs.12674.
- Barbera, A. C., C. Maucieri, V. Cavallaro, A. Ioppolo, and G. Spagna. 2013. Effects of spreading olive mill wastewater on soil properties and crops, a review. Agricultural Water Management 119:43–53. doi: 10.1016/j.agwat.2012.12.009.
- Barekat, S., and N. Soltanizadeh. 2017a. Effects of ultrasound on microstructure and enzyme penetration in beef Longissimus lumborum muscle. Food and Bioprocess Technology 11:680–93.
- Barekat, S., and N. Soltanizadeh. 2017b. Improvement of meat tenderness by simultaneous application of high-intensity ultrasonic radiation and papain treatment. Innovative Food Science & Emerging Technologies 39:223–9. doi: 10.1016/j.ifset.2016.12.009.
- Beckett, S. 2008. The science of chocolate. London, UK: The Royal Society of Chemistry. doi: 10.1039/9781847558053.
- Beckett, S. T. 2009. Non-conventional machines and processes, 4th Ed. Page Industrial Chocolate Manufacture and Use. Boston, MA: Springer. doi: 10.1002/9781444301588.ch17.
- Belca, L. M., A. Ručigaj, D. Teslič, and M. Krajnc. 2019. The use of ultrasound in the crystallization process of an active pharmaceutical ingredient. Ultrasonics Sonochemistry 58:104642. doi: 10.1016/J.ULTSONCH.2019.104642.
- Bhargava, N., R. S. Mor, K. Kumar, and V. S. Sharanagat. 2021. Advances in application of ultrasound in food processing: A review. Ultrasonics Sonochemistry 70:105293. doi: 10.1016/j.ultsonch.2020.105293.
- Bi, W., W. Ge, X. Li, L. Du, G. Zhao, H. Wang, and X. Qu. 2017. Effects of ultrasonic pretreatment and glycosylation on functional properties of casein grafted with glucose. Journal of Food Processing and Preservation 41 (5):e13177. doi: 10.1111/jfpp.13177.
- Bi, X., X. Wang, Y. Chen, L. Chen, Y. Xing, and Z. Che. 2020. Effects of combination treatments of lysozyme and high power ultrasound on the Salmonella typhimurium inactivation and quality of liquid whole egg. Ultrasonics Sonochemistry 60:104763. doi: 10.1016/j.ultsonch.2019.104763.
- Bimakr, M., R. A. Rahman, F. Saleena Taip, N. M. Adzahan, and Z. Islam Sarker. 2013. Ultrasound-assisted extraction of valuable compounds from winter melon (Benincasa hispida) seeds. International Food Research Journal 20:331–338.
- Bjarnsholt, T., K. Buhlin, Y. F. Dufrêne, M. Gomelsky, A. Moroni, M. Ramstedt, K. P. Rumbaugh, T. Schulte, L. Sun, B. Åkerlund, et al. 2018. Biofilm formation – What we can learn from recent developments. Journal of Internal Medicine 284 (4):332–45. doi: 10.1111/joim.12782.
- Boateng, E. F., and M. M. Nasiru. 2019. Applications of ultrasound in meat processing technology: A review. Food Science and Technology 7 (2):11–5. doi: 10.13189/fst.2019.070201.
- Cai, L., W. Zhang, A. Cao, M. Cao, and J. Li. 2019. Effects of ultrasonics combined with far infrared or microwave thawing on protein denaturation and moisture migration of Sciaenops ocellatus (red drum). Ultrasonics – Sonochemistry 55:96–104. doi: 10.1016/j.ultsonch.2019.03.017.
- Camara, H W. D., H. Doan, and A. Lohi. 2020. In-situ ultrasound-assisted control of polymeric membrane fouling. Ultrasonics 108:106206. doi: 10.1016/j.ultras.2020.106206.
- Cao, X., C. Cai, Y. Wang, and X. Zheng. 2018. The inactivation kinetics of polyphenol oxidase and peroxidase in bayberry juice during thermal and ultrasound treatments. Innovative Food Science & Emerging Technologies 45:169–78. doi: 10.1016/j.ifset.2017.09.018.
- Cappelletti, M., G. Ferrentino, and S. Spilimbergo. 2014. Supercritical carbon dioxide combined with high power ultrasound: An effective method for the pasteurization of coconut water. The Journal of Supercritical Fluids 92:257–63. doi: 10.1016/j.supflu.2014.06.010.
- Caraveo, O., A. D. Alarcon-Rojo, A. Renteria, E. Santellano, and L. Paniwnyk. 2015. Physicochemical and microbiological characteristics of beef treated with high-intensity ultrasound and stored at 4 °C. Journal of the Science of Food and Agriculture 95 (12):2487–93. doi: 10.1002/jsfa.6979.
- Cengiz, M. F., M. Başlar, O. Basançelebi, and M. Kılıçlı. 2018. Reduction of pesticide residues from tomatoes by low intensity electrical current and ultrasound applications. Food Chemistry 267:60–6. doi: 10.1016/j.foodchem.2017.08.031.
- Cervantes-Elizarrarás, A., J. Piloni-Martini, E. Ramírez-Moreno, E. Alanís-García, N. Güemes-Vera, C. A. Gómez-Aldapa, Q. Y. Zafra-Rojas, and N. D. S. Cruz-Cansino. 2017. Enzymatic inactivation and antioxidant properties of blackberry juice after thermoultrasound: Optimization using response surface methodology. Ultrasonics Sonochemistry 34:371–9. doi: 10.1016/j.ultsonch.2016.06.009.
- Chandrapala, J., C. Oliver, S. Kentish, and M. Ashokkumar. 2012. Ultrasonics in food processing. Ultrasonics Sonochemistry 19 (5):975–83. doi: 10.1016/j.ultsonch.2012.01.010.
- Chandrapala, J., B. Zisu, M. Palmer, S. Kentish, and M. Ashokkumar. 2011. Effects of ultrasound on the thermal and structural characteristics of proteins in reconstituted whey protein concentrate. Ultrasonics Sonochemistry 18 (5):951–7. doi: 10.1016/j.ultsonch.2010.12.016.
- Chang, H. J., Q. Wang, C. H. Tang, and G. H. Zhou. 2015. Effects of ultrasound treatment on connective tissue collagen and meat quality of beef semitendinosus muscle. Journal of Food Quality 38 (4):256–67. doi: 10.1111/jfq.12141.
- Chang, R., H. Lu, X. Bian, Y. Tian, and Z. Jin. 2021. Ultrasound assisted annealing production of resistant starches type 3 from fractionated debranched starch: Structural characterization and in-vitro digestibility. Food Hydrocolloids 110:106141. doi: 10.1016/j.foodhyd.2020.106141.
- Chavan, P., P. Sharma, S. R. Sharma, T. C. Mittal, and A. K. Jaiswal. 2022. Application of high-intensity ultrasound to improve food processing efficiency: A review. Foods 11 (1):122. doi: 10.3390/foods11010122.
- Chemat, F., and M. K. Khan, Zill-e-Huma. 2011. Applications of ultrasound in food technology: Processing, preservation and extraction. Ultrasonics Sonochemistry 18 (4):813–35. doi: 10.1016/j.ultsonch.2010.11.023.
- Chen, J., Y. Wang, J. Liu, and X. Xu. 2020. Preparation, characterization, physicochemical property and potential application of porous starch: A review. International Journal of Biological Macromolecules 148:1169–81. doi: 10.1016/J.IJBIOMAC.2020.02.055.
- Chen, W., X. Ma, W. Wang, R. Lv, M. Guo, T. Ding, X. Ye, S. Miao, and D. Liu. 2019. Preparation of modified whey protein isolate with gum acacia by ultrasound maillard reaction. Food Hydrocolloids. 95:298–307. doi: 10.1016/j.foodhyd.2018.10.030.
- Cheng, X. F., M. Zhang, and B. Adhikari. 2013. The inactivation kinetics of polyphenol oxidase in mushroom (Agaricus bisporus) during thermal and thermosonic treatments. Ultrasonics Sonochemistry 20 (2):674–9. doi: 10.1016/j.ultsonch.2012.09.012.
- Cheng, X. F., M. Zhang, B. Adhikari, M. N. Islam, and B. G. Xu. 2014. Effect of ultrasound irradiation on some freezing parameters of ultrasound-assisted immersion freezing of strawberries. International Journal of Refrigeration 44:49–55. doi: 10.1016/j.ijrefrig.2014.04.017.
- Cheng, X., M. Zhang, and B. Adhikari. 2014. Effects of ultrasound-assisted thawing on the quality of edamames [Glycine max (L.) Merrill]. Food Science and Biotechnology 23 (4):1095–102. doi: 10.1007/s10068-014-0150-0.
- Chia, S. R., K. W. Chew, P. L. Show, M. Sivakumar, T. C. Ling, and Y. Tao. 2019. Isolation of protein from Chlorella sorokiniana CY1 using liquid biphasic flotation assisted with sonication through sugaring-out effect. Journal of Oceanology and Limnology 37 (3):898–908. doi: 10.1007/s00343-019-8246-2.
- Chisti, Y. 2003. Sonobioreactors: Using ultrasound for enhanced microbial productivity. Trends in Biotechnology 21 (2):89–93. doi: 10.1016/S0167-7799(02)00033-1.
- Ciggin, A. S., E. S. Sarica, S. Doğruel, and D. Orhon. 2021. Impact of ultrasonic pretreatment on Fenton-based oxidation of olive mill wastewater – Towards a sustainable treatment scheme. Journal of Cleaner Production 313:127948. doi: 10.1016/j.jclepro.2021.127948.
- Comandini, P., G. Blanda, M. C. Soto-Caballero, V. Sala, U. Tylewicz, H. Mujica-Paz, A. Valdez Fragoso, and T. Gallina Toschi. 2013. Effects of power ultrasound on immersion freezing parameters of potatoes. Innovative Food Science & Emerging Technologies 18:120–5. doi: 10.1016/j.ifset.2013.01.009.
- Condón, S., P. Mañas, and G. Cebrián. 2011. Manothermosonication for microbial inactivation. In Food engineering series, 287–319. doi: 10.1007/978-1-4419-7472-3_11.
- Contreras, M., J. Benedito, and J. Bon. 2017. Intensification of heat transfer during mild thermal treatment of dry-cured ham by using airborne ultrasound. Ultrasonics – Sonochemistry 41:206–212. 10.1016/j.ultsonch.2017.09.019.
- Contreras-Lopez, G., A. Carnero-Hernandez, M. Huerta-Jimenez, A. D. Alarcon-Rojo, I. Garcia-Galicia, and L. M. Carrillo-López. 2020. High-intensity ultrasound applied on cured pork: Sensory and physicochemical characteristics. Food Science & Nutrition 8 (2):786–95. doi: 10.1002/FSN3.1321.
- Costa, M. G. M., T. V. Fonteles, A. L. T. de Jesus, F. D. L. Almeida, M. R. A. de Miranda, F A. N. Fernandes, and S. Rodrigues. 2013. High-intensity ultrasound processing of pineapple juice. Food and Bioprocess Technology 6 (4):997–1006. doi: 10.1007/s11947-011-0746-9.
- Coussios, C. C., C. H. Farny, G. ter Haar, and R. A. Roy. 2007. Role of acoustic cavitation in the delivery and monitoring of cancer treatment by high-intensity focused ultrasound (HIFU). International Journal of Hyperthermia: The Official Journal of European Society for Hyperthermic Oncology, North American Hyperthermia Group 23 (2):105–20. doi: 10.1080/02656730701194131.
- Cruz, R. M. S., M. C. Vieira, and C. L. M. Silva. 2006. Effect of heat and thermosonication treatments on peroxidase inactivation kinetics in watercress (Nasturtium officinale). Journal of Food Engineering 72 (1):8–15. doi: 10.1016/j.jfoodeng.2004.11.007.
- Cui, R., and F. Zhu. 2021. Ultrasound modified polysaccharides: A review of structure, physicochemical properties, biological activities and food applications. Trends in Food Science & Technology 107:491–508. doi: 10.1016/j.tifs.2020.11.018.
- Dabbour, M., R. He, B. Mintah, J. Xiang, and H. Ma. 2019. Changes in functionalities, conformational characteristics and antioxidative capacities of sunflower protein by controlled enzymolysis and ultrasonication action. Ultrasonics Sonochemistry 58:104625. doi: 10.1016/j.ultsonch.2019.104625.
- Dalvi-Isfahan, M., N. Hamdami, E. Xanthakis, and A. Le-Bail. 2017. Review on the control of ice nucleation by ultrasound waves, electric and magnetic fields. Journal of Food Engineering 195:222–34. doi: 10.1016/j.jfoodeng.2016.10.001.
- Debabrata, P., and M. Sivakumar. 2018. Sonochemical degradation of endocrine-disrupting organochlorine pesticide Dicofol: Investigations on the transformation pathways of dechlorination and the influencing operating parameters. Chemosphere 204:101–8. doi: 10.1016/j.chemosphere.2018.04.014.
- Deeth, H. C., and C. H. Fitz-Gerald. 2009. Lipolytic enzymes and hydrolytic rancidity. In Advanced dairy chemistry, eds. P. F Fox and P. L. H. McSweeney, 481–556. Boston: Springer. doi: 10.1007/0-387-28813-9_15.
- Delgado, A. E., L. Zheng, and D. Sun. 2009. Influence of ultrasound on freezing rate of immersion-frozen apples, 263–70. doi: 10.1007/s11947-008-0111-9.
- Delgado-Povedano, M. M., and M. D. Luque de Castro. 2015. A review on enzyme and ultrasound: A controversial but fruitful relationship. Analytica Chimica Acta 889:1–21. doi: 10.1016/j.aca.2015.05.004.
- Deora, N. S., N. N. Misra, A. Deswal, H. N. Mishra, P. J. Cullen, and B. K. Tiwari. 2013. Ultrasound for improved crystallisation in food processing. Food Engineering Reviews 5 (1):36–44. doi: 10.1007/s12393-012-9061-0.
- Dong, Y., Y. Xu, P. Li, C. Wang, Y. Cao, and J. Yu. 2017. Antibiofilm effect of ultrasound combined with microbubbles against Staphylococcus epidermidis biofilm. International Journal of Medical Microbiology: IJMM 307 (6):321–8. doi: 10.1016/j.ijmm.2017.06.001.
- Du, X., H. Li, M. Nuerjiang, S. Shi, B. Kong, Q. Liu, and X. Xia. 2021. Application of ultrasound treatment in chicken gizzards tenderization: Effects on muscle fiber and connective tissue. Ultrasonics Sonochemistry 79:105786. doi: 10.1016/J.ULTSONCH.2021.105786.
- Falsafi, S. R., Y. Maghsoudlou, H. Rostamabadi, M. M. Rostamabadi, H. Hamedi, and S. M. H. Hosseini. 2019. Preparation of physically modified oat starch with different sonication treatments. Food Hydrocolloids 89:311–20. doi: 10.1016/j.foodhyd.2018.10.046.
- Fan, D., L. Huang, B. Li, J. Huang, J. Zhao, B. Yan, W. Zhou, W. Zhang, and H. Zhang. 2016. Acoustic intensity in ultrasound field and ultrasound-assisted gelling of surimi. LWT 75:497–504. doi: 10.1016/j.lwt.2016.08.002.
- Fan, L., F. Hou, A. I. Muhammad, L. V. Ruiling, R. B. Watharkar, M. Guo, T. Ding, and D. Liu. 2019. Synergistic inactivation and mechanism of thermal and ultrasound treatments against Bacillus subtilis spores. Food Research International (Ottawa, Ont.) 116:1094–102. doi: 10.1016/j.foodres.2018.09.052.
- Fan, X. D., W. L. Zhang, H. Y. Xiao, T. Q. Qiu, and J. G. Jiang. 2015. Effects of ultrasound combined with ozone on the degradation of organophosphorus pesticide residues on lettuce. RSC Advances 5 (57):45622–30. doi: 10.1039/C5RA03024B.
- Ferrentino, G., and S. Spilimbergo. 2015. High pressure carbon dioxide combined with high power ultrasound pasteurization of fresh cut carrot. Journal of Supercritical Fluids 105:1–9. doi: 10.1016/j.supflu.2014.12.014.
- Filomena-Ambrosio, A., M. X. Quintanilla-Carvajal, I. Hernando, M. Hernández-Carrión, and I. Sotelo-Díaz, Ana-Puig. 2016. Changes of the water-holding capacity and microstructure of panga and Tilapia surimi gels using different stabilizers and processing methods. Food Science and Technology International = Ciencia y tecnologia de los alimentos internacional 22 (1):68–78., doi: 10.1177/1082013214568876.
- Fink, R., M. Oder, E. Stražar, and S. Filip. 2017. Efficacy of cleaning methods for the removal of Bacillus cereus biofilm from polyurethane conveyor belts in bakeries. Food Control 80:267–72. doi: 10.1016/j.foodcont.2017.05.009.
- Flores-Jiménez, N. T., J. A. Ulloa, J. E. U. Silvas, J. C. R. Ramírez, P. R. Ulloa, P. U. B. Rosales, Y. S. Carrillo, and R. G. Leyva. 2019. Effect of high-intensity ultrasound on the compositional, physicochemical, biochemical, functional and structural properties of canola (Brassica napus L.) protein isolate. Food Research International (Ottawa, Ont.) 121:947–56. doi: 10.1016/J.FOODRES.2019.01.025.
- Fonteles, T. V., M. G. M. Costa, A. L. T. de Jesus, M. R. A. de Miranda, F A. N. Fernandes, and S. Rodrigues. 2012. Power ultrasound processing of cantaloupe melon juice: Effects on quality parameters. Food Research International 48 (1):41–8. doi: 10.1016/j.foodres.2012.02.013.
- Gambuteanu, C., and P. Alexe. 2015. Comparison of thawing assisted by low-intensity ultrasound on technological properties of pork Longissimus dorsi muscle. Journal of Food Science and Technology 52 (4):2130–8. doi: 10.1007/s13197-013-1204-7.
- Gao, S., Y. Hemar, M. Ashokkumar, S. Paturel, and G. D. Lewis. 2014. Inactivation of bacteria and yeast using high-frequency ultrasound treatment. Water Research 60:93–104. doi: 10.1016/j.watres.2014.04.038.
- Gavahian, M., Y. H. Chu, and A. Mousavi Khaneghah. 2019. Recent advances in orange oil extraction: An opportunity for the valorisation of orange peel waste a review. International Journal of Food Science & Technology 54 (4):925–32. doi: 10.1111/ijfs.13987.
- Gavahian, M., G. N. Mathad, R. Pandiselvam, J. Lin, and D.-W. Sun. 2021. Emerging technologies to obtain pectin from food processing by-products: A strategy for enhancing resource efficiency. Trends in Food Science & Technology 115:42–54. doi: 10.1016/j.tifs.2021.06.018.
- Geng, M., Z. Wang, L. Qin, A. Taha, L. Du, X. Xu, S. Pan, and H. Hu. 2022. Effect of ultrasound and coagulant types on properties of β-carotene bulk emulsion gels stabilized by soy protein. Food Hydrocolloids 123:107146. doi: 10.1016/j.foodhyd.2021.107146.
- Georganas, A., E. Giamouri, A. C. Pappas, G. Papadomichelakis, F. Galliou, T. Manios, E. Tsiplakou, K. Fegeros, and G. Zervas. 2020. Bioactive compounds in food waste: A review on the transformation of food waste to animal feed. Foods 9 (3):291–18. doi: 10.3390/foods9030291.
- Gera, N., and S. Doores. 2011. Kinetics and mechanism of bacterial inactivation by ultrasound waves and sonoprotective effect of milk components. Journal of Food Science 76 (2):M111–9. doi: 10.1111/j.1750-3841.2010.02007.x.
- Gevari, M. T., T. Abbasiasl, S. Niazi, M. Ghorbani, and A. Koşar. 2020. Direct and indirect thermal applications of hydrodynamic and acoustic cavitation: A review. Applied Thermal Engineering 171:115065. doi: 10.1016/j.applthermaleng.2020.115065.
- Ghafoor, K., T. Hui, and Y. H. Choi. 2011. Optimization of ultrasonic‐assisted extraction of total anthocyanins from grape peel using response surface methodology. Journal of Food Biochemistry 35 (3):735–46. doi: 10.1111/j.1745-4514.2010.00413.x.
- Gholamhosseinpour, A., S. M. B. Hashemi, L. Raoufi Jahromi, and A. H. Sourki. 2020. Conventional heating, ultrasound and microwave treatments of milk: Fermentation efficiency and biological activities. International Dairy Journal 110:104809. doi: 10.1016/j.idairyj.2020.104809.
- Gómez-Cruz, I., C. Cara, M. del, M. Contreras, and I. Romero. 2020. Recovery of bioactive compounds from exhausted olive pomace. Proceedings 83:9. doi: 10.3390/iecbm2020-08582.
- Gómez-Salazar, J. A., D. A. Ochoa-Montes, A. Cerón-García, C. Ozuna, and M. E. Sosa-Morales. 2018. Effect of acid marination assisted by power ultrasound on the quality of rabbit meat. Journal of Food Quality 2018:1–6. doi: 10.1155/2018/5754930.
- Gonzalez-Gonzalez, L., A. D. Alarcon-Rojo, L. M. Carrillo-Lopez, I. A. Garcia-Galicia, M. Huerta-Jimenez, and L. Paniwnyk. 2020. Does ultrasound equally improve the quality of beef? An insight into Longissimus lumborum, Infraspinatus and Cleidooccipitalis. Meat Science 160:107963. doi: 10.1016/j.meatsci.2019.107963.
- González-González, L., L. Luna-Rodríguez, L. M. Carrillo-López, A. D. Alarcón-Rojo, I. García-Galicia, and R. Reyes-Villagrana. 2017. Ultrasound as an alternative to conventional marination: Acceptability and mass transfer. Journal of Food Quality 2017:1–8. doi: 10.1155/2017/8675720.
- Gracin, L., A. R. Jambrak, H. Juretić, S. Dobrović, H. Juretic´b, J. Juretic´b, S. Dobrovic´b, D. Dobrovic´b, I. B. Barukčicá, M. G. Grozdanovicá, et al. 2015. Influence of high power ultrasound on Brettanomyces and lactic acid bacteria in wine in continuous flow treatment. Applied Acoustics 103:143–7. doi: 10.1016/j.apacoust.2015.05.005.
- Guo, L., Y. Sun, Y. Zhu, B. Wang, L. Xu, M. Huang, Y. Li, and J. Sun. 2020. The antibacterial mechanism of ultrasound in combination with sodium hypochlorite in the control of Escherichia coli. Food Research International (Ottawa, Ont.) 129:108887. doi: 10.1016/j.foodres.2019.108887.
- Guo, M., L. Zhang, Q. He, S. A. Arabi, H. Zhao, W. Chen, X. Ye, and D. Liu. 2020. Synergistic antibacterial effects of ultrasound and thyme essential oils nanoemulsion against Escherichia coli O157:H7. Ultrasonics Sonochemistry 66:104988. doi: 10.1016/j.ultsonch.2020.104988.
- Guo, Y., B. Wu, X. Guo, D. Liu, P. Wu, H. Ma, and Z. Pan. 2021. Ultrasonication and thermosonication blanching treatments of carrot at varying frequencies: Effects on peroxidase inactivation mechanisms and quality characterization evaluation. Food Chemistry 343:128524. doi: 10.1016/j.foodchem.2020.128524.
- Habinshuti, I., T. H. Mu, and M. Zhang. 2021. Structural, antioxidant, aroma, and sensory characteristics of Maillard reaction products from sweet potato protein hydrolysates as influenced by different ultrasound-assisted enzymatic treatments. Food Chemistry 361:130090. doi: 10.1016/J.FOODCHEM.2021.130090.
- He, Q., M. Guo, T. Z. Jin, S. A. Arabi, and D. Liu. 2021. Ultrasound improves the decontamination effect of thyme essential oil nanoemulsions against Escherichia coli O157: H7 on cherry tomatoes. International Journal of Food Microbiology 337:108936. doi: 10.1016/j.ijfoodmicro.2020.108936.
- He, Q., D. Liu, M. Ashokkumar, X. Ye, T. Z. Jin, and M. Guo. 2021. Antibacterial mechanism of ultrasound against Escherichia coli: Alterations in membrane microstructures and properties. Ultrasonics Sonochemistry 73:105509. doi: 10.1016/j.ultsonch.2021.105509.
- Hernández-Falcón, T. A., A. Monter-Arciniega, N. d S. Cruz-Cansino, E. Alanís-García, G. M. Rodríguez-Serrano, A. Castañeda-Ovando, M. García-Garibay, E. Ramírez-Moreno, and J. Jaimez-Ordaz. 2018. Effect of thermoultrasound on aflatoxin M1 levels, physicochemical and microbiological properties of milk during storage. Ultrasonics Sonochemistry 48:396–403. doi: 10.1016/J.ULTSONCH.2018.06.018.
- Higaki, K., S. Ueno, T. Koyano, and K. Sato. 2001. Effects of ultrasonic irradiation on crystallization behavior of tripalmitoylglycerol and cocoa butter. Journal of the American Oil Chemists’ Society 78 (5):513–8. doi: 10.1007/s11746-001-0295-y.
- Higuera-Barraza, O. A., C. L. Del Toro-Sanchez, S. Ruiz-Cruz, and E. Márquez-Ríos. 2016. Effects of high-energy ultrasound on the functional properties of proteins. Ultrasonics Sonochemistry 31:558–62. doi: 10.1016/j.ultsonch.2016.02.007.
- Hu, A., S. Jiao, J. Zheng, L. Li, Y. Fan, L. Chen, and Z. Zhang. 2015. Ultrasonic frequency effect on corn starch and its cavitation. LWT – Food Science and Technology 60 (2):941–7. doi: 10.1016/j.lwt.2014.10.048.
- Hu, H., X. Fan, Z. Zhou, X. Xu, G. Fan, L. Wang, X. Huang, S. Pan, and L. Zhu. 2013. Acid-induced gelation behavior of soybean protein isolate with high intensity ultrasonic pre-treatments. Ultrasonics Sonochemistry 20 (1):187–95. doi: 10.1016/j.ultsonch.2012.07.011.
- Hu, H., E. C. Y. Li-Chan, L. Wan, M. Tian, and S. Pan. 2013. The effect of high intensity ultrasonic pre-treatment on the properties of soybean protein isolate gel induced by calcium sulfate. Food Hydrocolloids 32 (2):303–11. doi: 10.1016/j.foodhyd.2013.01.016.
- Hu, H., J. Wu, E. C. Y. Li-Chan, L. Zhu, F. Zhang, X. Xu, G. Fan, L. Wang, X. Huang, and S. Pan. 2013. Effects of ultrasound on structural and physical properties of soy protein isolate (SPI) dispersions. Food Hydrocolloids 30 (2):647–55. doi: 10.1016/j.foodhyd.2012.08.001.
- Hua, I., and U. Pfalzer-Thompson. 2001. Ultrasonic irradiation of carbofuran: Decomposition kinetics and reactor characterization. Water Research 35 (6):1445–52. doi: 10.1016/S0043-1354(00)00398-5.
- Huang, G., S. Chen, Y. Tang, C. Dai, L. Sun, H. Ma, and R. He. 2019. Stimulation of low intensity ultrasound on fermentation of skim milk medium for yield of yoghurt peptides by Lactobacillus paracasei. Ultrasonics Sonochemistry 51:315–24. doi: 10.1016/J.ULTSONCH.2018.09.033.
- Huang, H., K. C. Kwok, and H. H. Liang. 2008. Inhibitory activity and conformation changes of soybean trypsin inhibitors induced by ultrasound. Ultrasonics Sonochemistry 15 (5):724–30. doi: 10.1016/j.ultsonch.2007.10.007.
- Huezo, L., A. Shah, and F. C. M. Jr. 2019. Effects of ultrasound on fermentation of glucose to ethanol by Saccharomyces cerevisiae. Fermentation 5 (1):16. doi: 10.3390/fermentation5010016.
- Illera, A. E., M. T. Sanz, O. Benito-Román, S. Varona, S. Beltrán, R. Melgosa, and A. G. Solaesa. 2018. Effect of thermosonication batch treatment on enzyme inactivation kinetics and other quality parameters of cloudy apple juice. Innovative Food Science & Emerging Technologies 47:71–80. doi: 10.1016/j.ifset.2018.02.001.
- Inguglia, E. S., C. M. Burgess, J. P. Kerry, and B. K. Tiwari. 2019. Ultrasound-assisted marination: Role of frequencies and treatment time on the quality of sodium-reduced poultry meat. Foods 8 (10):473. doi: 10.3390/foods8100473.
- Iqbal, A., A. Murtaza, K. Marszałek, M. A. Iqbal, M. F. J. Chughtai, W. Hu, F. J. Barba, J. Bi, X. Liu, and X. Xu. 2020. Inactivation and structural changes of polyphenol oxidase in quince (Cydonia oblonga Miller) juice subjected to ultrasonic treatment. Journal of the Science of Food and Agriculture 100 (5):2065–73. doi: 10.1002/jsfa.10229.
- Islam, M. N., M. Zhang, and B. Adhikari. 2014. The inactivation of enzymes by ultrasound: A review of potential mechanisms. Food Reviews International 30 (1):1–21. doi: 10.1080/87559129.2013.853772.
- Jabbar, S., M. Abid, B. Hu, M. M. Hashim, S. Lei, T. Wu, and X. Zeng. 2015. Exploring the potential of thermosonication in carrot juice processing. Journal of Food Science and Technology 52 (11):7002–13. doi: 10.1007/s13197-015-1847-7.
- James, C., G. Purnell, and S. J. James. 2015. A review of novel and innovative food freezing technologies. Food and Bioprocess Technology 8:1616–34. doi: 10.1007/s11947-015-1542-8.
- Jankowska, M., B. Łozowicka, and P. Kaczyński. 2019. Comprehensive toxicological study over 160 processing factors of pesticides in selected fruit and vegetables after water, mechanical and thermal processing treatments and their application to human health risk assessment. The Science of the Total Environment 652:1156–67. doi: 10.1016/j.scitotenv.2018.10.324.
- Jayasooriya, S. D., B. R. Bhandari, P. Torley, B R. D. Arcy, B. R. Bhandari, P. Torley, and B R. D. A. Effect. 2007. Effect of high power ultrasound waves on properties of meat: A review effect of high power ultrasound waves on 2912. International Journal of Food Properties 7: 301–19. doi: 10.1081/JFP-120030039.
- Jiang, J., C. Gong, J. Wang, S. Tian, and Y. Zhang. 2014. Effects of ultrasound pre-treatment on the amount of dissolved organic matter extracted from food waste. Bioresource Technology 155:266–71. doi: 10.1016/J.BIORTECH.2013.12.064.
- Jin, H., Q. Zhao, H. Feng, Y. Wang, J. Wang, Y. Liu, D. Han, and J. Xu. 2019. Changes on the structural and physicochemical properties of conjugates prepared by the Maillard reaction of black bean protein isolates and glucose with ultrasound pretreatment. Polymers 11 (5):848. 848. doi: 10.3390/polym11050848.
- Jin, J., H. Ma, K. Wang, A. E. G. A. Yagoub, J. Owusu, W. Qu, R. He, C. Zhou, and X. Ye. 2015. Effects of multi-frequency power ultrasound on the enzymolysis and structural characteristics of corn gluten meal. Ultrasonics Sonochemistry 24:55–64. doi: 10.1016/j.ultsonch.2014.12.013.
- Esua, O., D.-W. Sun, C. K. Ajani, J.-H. Cheng, and K. M. Keener. 2022. Modelling of inactivation kinetics of Escherichia coli and Listeria monocytogenes on grass carp treated by combining ultrasound with plasma functionalized buffer. Ultrasonics Sonochemistry 88:106086. doi: 10.1016/J.ULTSONCH.2022.106086.
- Joo, H. J., M. F. R. Mizan, M. I. Hossain, D. U. Lee, and S. D. Ha. 2020. Enhanced elimination of Salmonella typhimurium and Campylobacter jejuni on chicken skin by sequential exposure to ultrasound and peroxyacetic acid. Journal of Food Safety 40 (4):e12803. doi: 10.1111/jfs.12803.
- Kadkhodaee, R., and M. J. W. Povey. 2008. Ultrasonic inactivation of Bacillus α-amylase. I. effect of gas content and emitting face of probe. Ultrasonics Sonochemistry 15 (2):133–42. doi: 10.1016/j.ultsonch.2007.02.005.
- Kang, D. C., Y. H. Zou, Y. P. Cheng, L. J. Xing, G. H. Zhou, and W. G. Zhang. 2016. Effects of power ultrasound on oxidation and structure of beef proteins during curing processing. Ultrasonics Sonochemistry 33:47–53. doi: 10.1016/j.ultsonch.2016.04.024.
- Kang, D. c., X. q Gao, Q. f Ge, G. h Zhou, and W. g Zhang. 2017. Effects of ultrasound on the beef structure and water distribution during curing through protein degradation and modification. Ultrasonics Sonochemistry 38:317–25. doi: 10.1016/j.ultsonch.2017.03.026.
- Kashyap, D. R., P. K. Vohra, S. Chopra, and R. Tewari. 2001. Applications of pectinases in the commercial sector: A review. Bioresource Technology 77 (3):215–27. doi: 10.1016/S0960-8524(00)00118-8.
- Kiani, H., and D. Sun. 2017. Numerical simulation of heat transfer and phase change during freezing of potatoes with different shapes at the presence or absence of ultrasound irradiation. Heat and Mass Transfer 54:885–94.
- Kida, M., S. Ziembowicz, and P. Koszelnik. 2018. Removal of organochlorine pesticides (OCPs) from aqueous solutions using hydrogen peroxide, ultrasonic waves, and a hybrid process. Separation and Purification Technology 192:457–64. doi: 10.1016/j.seppur.2017.10.046.
- Kidak, R., and N. H. Ince. 2006. Ultrasonic destruction of phenol and substituted phenols: A review of current research. Ultrasonics Sonochemistry 13 (3):195–9. doi: 10.1016/j.ultsonch.2005.11.004.
- Kjartansson, G. T., S. Zivanovic, K. Kristbergsson, and J. Weiss. 2006. Sonication-assisted extraction of chitin from shells of fresh water prawns (Macrobrachium rosenbergii). Journal of Agricultural and Food Chemistry 54 (9):3317–23. doi: 10.1021/jf052184c.
- Koca, N., M. Urgu, and T. E. Saatli. 2018. Novel technologies in dairy processing. In Technological approaches for novel applications in dairy processing – Google books, ed. N. Koca, 51–70. London: IntechOpen.
- Koda, S., M. Miyamoto, M. Toma, T. Matsuoka, and M. Maebayashi. 2009. Inactivation of Escherichia coli and Streptococcus mutans by ultrasound at 500 kHz. Ultrasonics Sonochemistry 16 (5):655–9. doi: 10.1016/j.ultsonch.2009.02.003.
- Koo, H., R. N. Allan, R. P. Howlin, P. Stoodley, and L. Hall-Stoodley. 2017. Targeting microbial biofilms: Current and prospective therapeutic strategies. Nature Reviews Microbiology 15 (12):740–55. doi: 10.1038/nrmicro.2017.99.
- Körzendörfer, A., J. Schäfer, J. Hinrichs, and S. Nöbel. 2019. Power ultrasound as a tool to improve the processability of protein-enriched fermented milk gels for Greek yogurt manufacture. Journal of Dairy Science 102 (9):7826–37. doi: 10.3168/jds.2019-16541.
- Kulmann de Medeiros, J., J. R. Sarkis, D. P. Jaeschke, and G. D. Mercali. 2021. Thermosonication for peroxidase inactivation in sugarcane juice. LWT 140:110730. doi: 10.1016/j.lwt.2020.110730.
- Kumar, K., A. N. Yadav, V. Kumar, P. Vyas, and H. S. Dhaliwal. 2017. Food waste: A potential bioresource for extraction of nutraceuticals and bioactive compounds. Bioresources and Bioprocessing 4 (1):1–14. doi: 10.1186/s40643-017-0148-6.
- Kyllönen, H. M., P. Pirkonen, and M. Nyström. 2005. Membrane filtration enhanced by ultrasound: A review. Desalination 181 (1-3):319–35. doi: 10.1016/j.desal.2005.06.003.
- Lauterborn, W., and R. Mettin. 2015. Acoustic cavitation: Bubble dynamics in high-power ultrasonic fields. In Power ultrasonics: Applications of high-intensity ultrasound, 37–78. doi: 10.1016/B978-1-78242-028-6.00003-X.
- Lee, J., R. C. da Silva, V. Gibon, and S. Martini. 2018. Sonocrystallization of interesterified soybean oil: Effect of saturation level and supercooling. Journal of Food Science 83 (4):902–10. doi: 10.1111/1750-3841.14084.
- Lee, N. Y., S. W. Kim, and S. D. Ha. 2014. Synergistic effects of ultrasound and sodium hypochlorite (NaOCl) on reducing Listeria monocytogenes ATCC19118 in broth, stainless steel, and iceberg lettuce. Foodborne Pathogens and Disease 11 (7):581–7. doi: 10.1089/fpd.2013.1722.
- Li, D., H. Zhao, A. I. Muhammad, L. Song, M. Guo, and D. Liu. 2020. The comparison of ultrasound-assisted thawing, air thawing and water immersion thawing on the quality of slow/fast freezing bighead carp (Aristichthys nobilis) fillets. Food Chemistry 320:126614. doi: 10.1016/j.foodchem.2020.126614.
- Li, J., J. Ahn, D. Liu, S. Chen, X. Ye, and T. Ding. 2016. Evaluation of ultrasound-induced damage to Escherichia coli and Staphylococcus aureus by flow cytometry and transmission electron microscopy. Applied and Environmental Microbiology 82 (6):1828–37. doi: 10.1128/AEM.03080-15.
- Li, J., Y. Suo, X. Liao, J. Ahn, D. Liu, S. Chen, X. Ye, and T. Ding. 2017. Analysis of Staphylococcus aureus cell viability, sublethal injury and death induced by synergistic combination of ultrasound and mild heat. Ultrasonics Sonochemistry 39:101–10. doi: 10.1016/j.ultsonch.2017.04.019.
- Li, K., Z. L. Kang, Y. Y. Zhao, X. L. Xu, and G. H. Zhou. 2014. Use of high-intensity ultrasound to improve functional properties of batter suspensions prepared from PSE-like chicken breast meat. Food and Bioprocess Technology 7 (12):3466–77. doi: 10.1007/s11947-014-1358-y.
- Li, K., Z. L. Kang, Y. F. Zou, X. L. Xu, and G. H. Zhou. 2015. Effect of ultrasound treatment on functional properties of reduced-salt chicken breast meat batter. Journal of Food Science and Technology 52 (5):2622–33. doi: 10.1007/s13197-014-1356-0.
- Li, L., A. Taha, M. Geng, Z. Zhang, H. Su, X. Xu, S. Pan, and H. Hu. 2021. Ultrasound-assisted gelation of β-carotene enriched oleogels based on Candelilla wax-nut oils: Physical properties and in-vitro digestion analysis. Ultrasonics Sonochemistry 79:105762. doi: 10.1016/j.ultsonch.2021.105762.
- Li, W., T. S. H. Leong, M. Ashokkumar, and G. J. O. Martin. 2017. A study of the effectiveness and energy efficiency of ultrasonic emulsification. Physical Chemistry Chemical Physics: PCCP 20 (1):86–96. doi: 10.1039/c7cp07133g.
- Liang, Y., W. Wang, Y. Shen, Y. Liu, and X. J. Liu. 2012. Effects of home preparation on organophosphorus pesticide residues in raw cucumber. Food Chemistry 133 (3):636–40. doi: 10.1016/j.foodchem.2012.01.016.
- Liao, X., J. Li, Y. Suo, S. Chen, X. Ye, D. Liu, and T. Ding. 2018. Multiple action sites of ultrasound on Escherichia coli and Staphylococcus aureus. Food Science and Human Wellness 7 (1):102–9. doi: 10.1016/j.fshw.2018.01.002.
- Lin, J., Y. Zhi-Wei, J. Lin, and Y. Zhi-Wei. 2020. Optimization of glycosylation modification process of ultrasound-assisted naked oat protein/β-glucan by response surface methodology. Science and Technology of Food Industry 41 (2):170–6. 41:170–176. doi: 10.13386/J.ISSN1002-0306.2020.02.027.
- Lin, L., X. Wang, C. Li, and H. Cui. 2019. Inactivation mechanism of E. coli O157:H7 under ultrasonic sterilization. Ultrasonics Sonochemistry 59:104751. doi: 10.1016/j.ultsonch.2019.104751.
- Liu, K., H. Wang, and X. Zhang. 2020. Ductile mode cutting of calcium fluoride. Ductile mode cutting of brittle materials, 179–210. Singapore: Springer. doi: 10.1007/978-981-32-9836-1_9.
- Liu, Y., S. Chen, Y. Pu, A. I. Muhammad, M. Hang, D. Liu, and T. Ye. 2019. Ultrasound-assisted thawing of mango pulp: Effect on thawing rate, sensory, and nutritional properties. Food Chemistry 286: 576–83. doi: 10.1016/j.foodchem.2019.02.059.
- Liu, Y., M. Li, Y. Liu, F. Bai, and K. Bian. 2019. Effects of pulsed ultrasound at 20 kHz on the sonochemical degradation of mycotoxins. World Mycotoxin Journal 12 (4):357–66. doi: 10.3920/WMJ2018.2431.
- Liu, Y., M. Li, Y. Liu, and K. Bian. 2019. Structures of reaction products and degradation pathways of aflatoxin B1 by ultrasound treatment. Toxins 11 (9):526. doi: 10.3390/toxins11090526.
- Lopez, P., and J. Burgos. 1995. Peroxidase stability and reactivation after heat treatment and manothermosonication. Journal of Food Science 60 (3):451–5. doi: 10.1111/j.1365-2621.1995.tb09801.x.
- Lopez, P., and J. Burgos. 1995. Lipoxygenase inactivation by manothermosonication: Effects of sonication physical parameters, pH, KC1, sugars, glycerol, and enzyme concentration. Journal of Agricultural and Food Chemistry 43 (3):620–5. doi: 10.1021/jf00051a012.
- López, P., F. J. Sala, J. L. de la Fuente, S. Condón, J. Raso, and J. Burgos. 1994. Inactivation of peroxidase, lipoxygenase, and polyphenol oxidase by manothermosonication. Journal of Agricultural and Food Chemistry 42 (2):252–6. doi: 10.1021/jf00038a005.
- Lozowicka, B., M. Jankowska, I. Hrynko, and P. Kaczynski. 2016. Removal of 16 pesticide residues from strawberries by washing with tap and ozone water, ultrasonic cleaning and boiling. Environmental Monitoring and Assessment 188 (1):19. doi: 10.1007/s10661-015-4850-6.
- Luche, J.-L. 1998. Synthetic organic sonochemistry. doi: 10.1007/978-1-4899-1910-6.
- Ma, H., Y. Lin, Y. Jin, M. Gao, H. Li, Q. Wang, S. Ge, L. Cai, Z. Huang, Q. Van Le, et al. 2021. Effect of ultrasonic pretreatment on chain elongation of saccharified residue from food waste by anaerobic fermentation. Environmental Pollution (Barking, Essex: 1987) 268 (Pt B):115936. doi: 10.1016/j.envpol.2020.115936.
- Ma, X., F. Hou, H. Zhao, D. Wang, W. Chen, S. Miao, and D. Liu. 2020. Conjugation of soy protein isolate (SPI) with pectin by ultrasound treatment. Food Hydrocolloids 108:106056. doi: 10.1016/j.foodhyd.2020.106056.
- Mahamuni, N. N., and Y. G. Adewuyi. 2010. Advanced oxidation processes (AOPs) involving ultrasound for waste water treatment: A review with emphasis on cost estimation. Ultrasonics Sonochemistry 17 (6):990–1003. doi: 10.1016/j.ultsonch.2009.09.005.
- Mahato, S., Z. Zhu, and D. Sun. 2019. Glass transitions as affected by food compositions and by conventional and novel freezing technologies: A review. Trends in Food Science & Technology 94:1–11. doi: 10.1016/j.tifs.2019.09.010.
- Majid, I., G. A. Nayik, and V. Nanda. 2015. Ultrasonication and food technology: A review. Cogent Food & Agriculture 1 (1):1071022. doi: 10.1080/23311932.2015.1071022.
- Martínez-Velasco, A., C. Lobato-Calleros, B. E. Hernández-Rodríguez, A. Román-Guerrero, J. Alvarez-Ramirez, and E. J. Vernon-Carter. 2018. High intensity ultrasound treatment of faba bean (Vicia faba L.) protein: Effect on surface properties, foaming ability and structural changes. Ultrasonics Sonochemistry 44:97–105. doi: 10.1016/j.ultsonch.2018.02.007.
- Mason, T. J., and C. Pétrier. 2004. Ultrasound processes. . In Advanced oxidation processes for water and wastewater treatment, ed. S. Parsons, 185–208. London: IWA Publishing.
- McClements, D. J. 1995. Advances in the application of ultrasound in food analysis and processing. Trends in Food Science & Technology 6 (9):293–9. doi: 10.1016/S0924-2244(00)89139-6.
- McDonnell, C. K., P. Allen, C. Morin, and J. G. Lyng. 2014. The effect of ultrasonic salting on protein and water-protein interactions in meat. Food Chemistry 147:245–51. doi: 10.1016/j.foodchem.2013.09.125.
- Medina-Meza, I. G., P. Boioli, and G. V. Barbosa-Cánovas. 2016. Assessment of the effects of ultrasonics and pulsed electric fields on nutritional and rheological properties of raspberry and blueberry purees. Food and Bioprocess Technology 9 (3):520–31. doi: 10.1007/s11947-015-1642-5.
- Millan-Sango, D., A. McElhatton, and V. P. Valdramidis. 2015. Determination of the efficacy of ultrasound in combination with essential oil of oregano for the decontamination of Escherichia coli on inoculated lettuce leaves. Food Research International 67:145–54. doi: 10.1016/j.foodres.2014.11.001.
- Monroy, Y., S. Rivero, and M. A. García. 2018. Microstructural and techno-functional properties of cassava starch modified by ultrasound. Ultrasonics Sonochemistry 42:795–804. doi: 10.1016/J.ULTSONCH.2017.12.048.
- Morales, R., K. D. Martínez, V. M. Pizones Ruiz-Henestrosa, and A. M. R. Pilosof. 2015. Modification of foaming properties of soy protein isolate by high ultrasound intensity: Particle size effect. Ultrasonics Sonochemistry 26:48–55. doi: 10.1016/j.ultsonch.2015.01.011.
- Mortazavia, S. M., A. M. Sania, and S. Mohsenib. 2015. Destruction of AFT by ultrasound treatment. Journal of Applied Environmental and Biological Sciences 4:198–202.
- Muthoosamy, K., and S. Manickam. 2017. State of the art and recent advances in the ultrasound-assisted synthesis, exfoliation and functionalization of graphene derivatives. Ultrasonics Sonochemistry 39:478–93. doi: 10.1016/j.ultsonch.2017.05.019.
- Naddeo, V., M F. N. Secondes, L. Borea, S. W. Hasan, F. Ballesteros, and V. Belgiorno. 2020. Removal of contaminants of emerging concern from real wastewater by an innovative hybrid membrane process – UltraSound, Adsorption, and Membrane ultrafiltration (USAMe®). Ultrasonics Sonochemistry 68:105237. doi: 10.1016/J.ULTSONCH.2020.105237.
- Naji, O., R. A. Al-Juboori, L. Bowtell, A. Alpatova, and N. Ghaffour. 2020. Direct contact ultrasound for fouling control and flux enhancement in air-gap membrane distillation. Ultrasonics Sonochemistry 61:104816. doi: 10.1016/j.ultsonch.2019.104816.
- Nalajala, V. S., and V. S. Moholkar. 2011. Investigations in the physical mechanism of sonocrystallization. Ultrasonics Sonochemistry 18 (1):345–55. doi: 10.1016/J.ULTSONCH.2010.06.016.
- Nowacka, M., M. Dadan, and U. Tylewicz. 2021. Current applications of ultrasound in fruit and vegetables osmotic dehydration processes. Applied Sciences 11 (3):1269–22. doi: 10.3390/app11031269.
- Nowacka, M., and M. Wedzik. 2016. Effect of ultrasound treatment on microstructure, colour and carotenoid content in fresh and dried carrot tissue. Applied Acoustics 103:163–71. doi: 10.1016/j.apacoust.2015.06.011.
- Nowak, K. W., M. Zielinska, and K. M. Waszkielis. 2019. The effect of ultrasound and freezing/thawing treatment on the physical properties of blueberries. Food Science and Biotechnology 28 (3):741–9. doi: 10.1007/s10068-018-0528-5.
- Ojha, K. S., D. F. Keenan, A. Bright, J. P. Kerry, and B. K. Tiwari. 2016. Ultrasound assisted diffusion of sodium salt replacer and effect on physicochemical properties of pork meat. International Journal of Food Science & Technology 51 (1):37–45. doi: 10.1111/ijfs.13001.
- Ojha, K. S., T. J. Mason, C. P. O'Donnell, J. P. Kerry, and B. K. Tiwari. 2017. Ultrasound technology for food fermentation applications. Ultrasonics Sonochemistry 34:410–7. doi: 10.1016/j.ultsonch.2016.06.001.
- Okonkwo, V. C., E. M. Kwofie, O. I. Mba, and M. O. Ngadi. 2021. Impact of thermo-sonication on quality indices of starch-based sauces. Ultrasonics Sonochemistry 73:105473. doi: 10.1016/J.ULTSONCH.2021.105473.
- Oliveira, H. M., V. S. Correia, M. A. Segundo, A. J. M. Fonseca, and A. R. J. Cabrita. 2017. Does ultrasound improve the activity of alpha amylase? A comparative study towards a tailor-made enzymatic hydrolysis of starch. LWT 84:674–85. doi: 10.1016/j.lwt.2017.06.035.
- Ortuño, C., M. T. Martínez-Pastor, A. Mulet, and J. Benedito. 2013. Application of high power ultrasound in the supercritical carbon dioxide inactivation of Saccharomyces cerevisiae. Food Research International 51 (2):474–81. doi: 10.1016/j.foodres.2013.01.041.
- O'Sullivan, J., M. Park, and J. Beevers. 2016. The effect of ultrasound upon the physicochemical and emulsifying properties of wheat and soy protein isolates. Journal of Cereal Science 69:77–84. doi: 10.1016/j.jcs.2016.02.013.
- Oztekin, R., and D. T. Sponza. 2021. Treatment of wastewaters from the olive mill industry wastewaters by sonication process at different conditions. Asian Journal of Applied Chemistry Research 8 (4):7–53. doi: 10.9734/ajacr/2021/v8i430197.
- Christen, P., and A. López-Munguía. 1994. Enzymes and food flavor. Food Biotechnology 8 (2-3):167–90. doi: 10.1080/08905439409549874.
- Paraskeva, P., and E. Diamadopoulos. 2006. Technologies for olive mill wastewater (OMW) treatment: A review. Journal of Chemical Technology & Biotechnology 81 (9):1475–85. doi: 10.1002/jctb.1553.
- Park, J. S., and J. W. Ha. 2019. Ultrasound treatment combined with fumaric acid for inactivating food-borne pathogens in apple juice and its mechanisms. Food Microbiology 84:103277. doi: 10.1016/j.fm.2019.103277.
- Patil, A. L., P. N. Patil, and P. R. Gogate. 2014. Degradation of imidacloprid containing wastewaters using ultrasound based treatment strategies. Ultrasonics Sonochemistry 21 (5):1778–86. doi: 10.1016/j.ultsonch.2014.02.029.
- Pawar, S. V., and V. K. Rathod. 2020. Role of ultrasound in assisted fermentation technologies for process enhancements. Preparative Biochemistry & Biotechnology. 50 (6):627–34. doi: 10.1080/10826068.2020.1725773. PMID: 32065573
- Pérez-Andrés, J. M., C. M. G. Charoux, P. J. Cullen, and B. K. Tiwari. 2018. Chemical modifications of lipids and proteins by nonthermal food processing technologies. Journal of Agricultural and Food Chemistry 66 (20):5041–54. doi: 10.1021/acs.jafc.7b06055.
- Qiu, L., M. Zhang, B. Chitrakar, and B. Bhandari. 2020. Application of power ultrasound in freezing and thawing processes: Effect on process efficiency and product quality. Ultrasonics Sonochemistry 68:105230. doi: 10.1016/j.ultsonch.2020.105230.
- Raut-Jadhav, S., D. V. Pinjari, D. R. Saini, S. H. Sonawane, and A. B. Pandit. 2016. Intensification of degradation of methomyl (carbamate group pesticide) by using the combination of ultrasonic cavitation and process intensifying additives. Ultrasonics Sonochemistry 31:135–42. doi: 10.1016/j.ultsonch.2015.12.015.
- Raviyan, P., Z. Zhang, and H. Feng. 2005. Ultrasonication for tomato pectinmethylesterase inactivation: Effect of cavitation intensity and temperature on inactivation. Journal of Food Engineering 70 (2):189–96. doi: 10.1016/j.jfoodeng.2004.09.028.
- Razola-Díaz, M. D. C., E. J. Guerra-Hernández, C. Rodríguez-Pérez, A. M. Gómez-Caravaca, B. García-Villanova, and V. Verardo. 2021. Optimization of ultrasound-assisted extraction via sonotrode of phenolic compounds from orange by-products. Foods 10 (5):1120. doi: 10.3390/foods10051120.
- Riesz, P., and T. Kondo. 1992. Free radical formation induced by ultrasound and its biological implications. Free Radical Biology & Medicine 13 (3):247–70. doi: 10.1016/0891-5849(92)90021-8.
- Robinson, D. S. 1991. Peroxidases and catalases in foods. Oxidative Enzymes in Foods, 1–45.
- Arancon, R. N. 2013. Market and trade of coconut products. Experts’ Consultation on Coconut Sector Development in the Asia and the Pacific.
- Ruan, S., J. Luo, Y. Li, Y. Wang, S. Huang, F. Lu, and H. Ma. 2020. Ultrasound-assisted liquid-state fermentation of soybean meal with Bacillus subtilis: Effects on peptides content, ACE inhibitory activity and biomass. Process Biochemistry 91:73–82. doi: 10.1016/j.procbio.2019.11.035.
- Rubio, F., E. D. Blandford, and L. J. Bond. 2016. Survey of advanced nuclear technologies for potential applications of sonoprocessing. Ultrasonics 71:211–22. doi: 10.1016/j.ultras.2016.06.017.
- Ruecroft, G. 2007. Ultrasound, crystallisation & drug formulation. Specialty Chemicals Magazine 27 (5): 60–2.
- Sabnis, S. S., S. D. Singh, and P. R. Gogate. 2022. Improvements in azithromycin recrystallization using ultrasound for size reduction. Ultrasonics Sonochemistry 83:105922. doi: 10.1016/J.ULTSONCH.2022.105922.
- Saeeduddin, M., M. Abid, S. Jabbar, T. Wu, M. M. Hashim, F. N. Awad, B. Hu, S. Lei, and X. Zeng. 2015. Quality assessment of pear juice under ultrasound and commercial pasteurization processing conditions. LWT - Food Science and Technology 64 (1):452–8. doi: 10.1016/j.lwt.2015.05.005.
- Saleem, R., and R. Ahmad. 2016. Effect of ultrasonication on secondary structure and heat induced gelation of chicken myofibrils. Journal of Food Science and Technology 53 (8):3340–8. doi: 10.1007/s13197-016-2311-z.
- Santos, M. V., Y. Ma, Z. Caplan, and D. M. Barbano. 2003. Sensory threshold of off-flavors caused by proteolysis and lipolysis in milk. Journal of Dairy Science 86 (5):1601–7. doi: 10.3168/jds.S0022-0302(03)73745-X.
- Savchenko, M., M. Hurtado, M. T. Lopez-Lopez, G. Rus, L. Álvarez de Cienfuegos, J. Melchor, and J. A. Gavira. 2022. Lysozyme crystallization in hydrogel media under ultrasound irradiation. Ultrasonics Sonochemistry 88:106096. doi: 10.1016/J.ULTSONCH.2022.106096.
- Schneider, Y., S. Zahn, and H. Rohm. 2008. Power requirements of the high-frequency generator in ultrasonic cutting of foods. Journal of Food Engineering 86 (1):61–7. doi: 10.1016/j.jfoodeng.2007.09.024.
- Schramm, J. D., and I. Hua. 2001. Ultrasonic irradiation of dichlorvos: Decomposition mechanism. Water Research 35 (3):665–74. doi: 10.1016/S0043-1354(00)00304-3.
- Sharma, P., V. K. Gaur, S. H. Kim, and A. Pandey. 2020. Microbial strategies for bio-transforming food waste into resources. Bioresource Technology 299:122580. doi: 10.1016/j.biortech.2019.122580.
- Sharma, P., V. K. Gaur, R. Sirohi, S. Varjani, S. Hyoun Kim, and J. W. C. Wong. 2021. Sustainable processing of food waste for production of bio-based products for circular bioeconomy. Bioresource Technology 325:124684. doi: 10.1016/j.biortech.2021.124684.
- Sheng, L., Y. Wang, J. Chen, J. Zou, Q. Wang, and M. Ma. 2018. Influence of high-intensity ultrasound on foaming and structural properties of egg white. Food Research International (Ottawa, Ont.) 108:604–10. doi: 10.1016/j.foodres.2018.04.007.
- Shi, H., X. Zhang, X. Chen, R. Fang, Y. Zou, D. Wang, and W. Xu. 2020. How ultrasound combined with potassium alginate marination tenderizes old chicken breast meat: Possible mechanisms from tissue to protein. Food Chemistry 328:127144. doi: 10.1016/J.FOODCHEM.2020.127144.
- Shi, Z., S. Zhong, W. Yan, M. Liu, Z. Yang, and X. Qiao. 2019. The effects of ultrasonic treatment on the freezing rate, physicochemical quality, and microstructure of the back muscle of grass carp (Ctenopharyngodon idella). LWT 111:301–8. doi: 10.1016/j.lwt.2019.04.071.
- Shriwas, A. K., and P. R. Gogate. 2011. Ultrasonic degradation of methyl Parathion in aqueous solutions: Intensification using additives and scale up aspects. Separation and Purification Technology 79 (1):1–7. doi: 10.1016/j.seppur.2011.02.034.
- da Silva, T. L. T., S. Danthine, and S. Martini. 2021. Palm-based fat crystallized at different temperatures with and without high-intensity ultrasound in batch and in a scraped surface heat exchanger. LWT 138:110593. doi: 10.1016/j.lwt.2020.110593.
- Silva, T. L. T. d., M. Marsh, V. Gibon, and S. Martini. 2020. Sonocrystallization as a tool to reduce oil migration by changing physical properties of a palm kernel fat. Journal of Food Science 85 (4):964–71. doi: 10.1111/1750-3841.15099.
- Singh, S., S. K. Singh, I. Chowdhury, and R. Singh. 2017. Understanding the mechanism of bacterial biofilms resistance to antimicrobial agents. The Open Microbiology Journal 11:53–62. doi: 10.2174/1874285801711010053.
- Singla, M., and N. Sit. 2021.Application of ultrasound in combination with other technologies in food processing: A review. Ultrasonics Sonochemistry 73:105506. doi: 10.1016/j.ultsonch.2021.105506.
- Siró, I., C. Vén, C. Balla, G. Jónás, I. Zeke, and L. Friedrich. 2009. Application of an ultrasonic assisted curing technique for improving the diffusion of sodium chloride in porcine meat. Journal of Food Engineering 91 (2):353–62. doi: 10.1016/j.jfoodeng.2008.09.015.
- Słowik-Borowiec, M., and E. Szpyrka. 2020. Selected food processing techniques as a factor for pesticide residue removal in apple fruit. Environmental Science and Pollution Research International 27 (2):2361–73. doi: 10.1007/s11356-019-06943-9.
- Stadnik, J., and Z. J. Dolatowski. 2011. Influence of sonication on Warner-Bratzler shear force, colour and myoglobin of beef (M. semimembranosus). 553–9. doi: 10.1007/s00217-011-1550-5.
- Su, G., X. Zheng, J. Zou, G I. N. Waterhouse, and D. Sun-Waterhouse. 2021. Insight into the advantages of premixing yeast-wheat gluten and combining ultrasound and transglutaminase pretreatments in producing umami enzymatic protein hydrolysates. Food Chemistry 342:128317. doi: 10.1016/J.FOODCHEM.2020.128317.
- Su, Y., M. Zhang, B. Bhandari, and W. Zhang. 2018. Enhancement of water removing and the quality of fried purple-fleshed sweet potato in the vacuum frying by combined power ultrasound and microwave technology. Ultrasonics Sonochemistry 44:368–79. doi: 10.1016/j.ultsonch.2018.02.049.
- Sukhatskiy, Y. V., Z. O. Znak, and O. I. Zin. 2020. Cavitation and its combinations with other advanced oxidation processes in phenol wastewater treatment: A review. Voprosy khimii i khimicheskoi tekhnologii.
- Sun, Q., F. Sun, X. Xia, H. Xu, and B. Kong. 2019. The comparison of ultrasound-assisted immersion freezing, air freezing and immersion freezing on the muscle quality and physicochemical properties of common carp (Cyprinus carpio) during freezing storage. Ultrasonics Sonochemistry 51:281–91. doi: 10.1016/j.ultsonch.2018.10.006.
- Suzuki, A. H., J. Lee, S. G. Padilla, and S. Martini. 2010. Altering functional properties of fats using power ultrasound. Journal of Food Science 75 (4):E208–E214. doi: 10.1111/j.1750-3841.2010.01572.x.
- Taha, A., E. Ahmed, A. Ismaiel, M. Ashokkumar, X. Xu, S. Pan, and H. Hu. 2020. Ultrasonic emulsification: An overview on the preparation of different emulsifiers-stabilized emulsions. Trends in Food Science & Technology 105:363–77. doi: 10.1016/j.tifs.2020.09.024.
- Taheri, M. E., E. Salimi, K. Saragas, J. Novakovic, E. M. Barampouti, S. Mai, D. Malamis, K. Moustakas, and M. Loizidou. 2021. Effect of pretreatment techniques on enzymatic hydrolysis of food waste. Biomass Conversion and Biorefinery 11 (2):219–26. doi: 10.1007/s13399-020-00729-7.
- Taylor, P. 2015. Enhancement of food processes by ultrasound : A enhancement of food processes by ultrasound : A review. Critical Reviews in Food Science and Nutrition 55:570–94. doi: 10.1080/10408398.2012.667849.
- Taylor, P., B. Xu, M. Zhang, B. Bhandari, X. Cheng, B. Xu, M. Zhang, B. Bhandari, and X. Cheng. 2014. Influence of ultrasound-assisted osmotic dehydration and freezing on the water state, cell structure, and quality of radish (Raphanus sativus L.) cylinders influence of ultrasound-assisted osmotic dehydrati. Drying Technology : An International Journal 37–41. doi: 10.1080/07373937.2014.947427.
- Terefe, N. S., M. Gamage, K. Vilkhu, L. Simons, R. Mawson, and C. Versteeg. 2009. The kinetics of inactivation of pectin methylesterase and polygalacturonase in tomato juice by thermosonication. Food Chemistry 117 (1):20–7. doi: 10.1016/j.foodchem.2009.03.067.
- Tervo, J. T., R. Mettin, and W. Lauterborn. 2006. Bubble cluster dynamics in acoustic cavitation. Acta Acustica united with Acustica 92:178–80.
- Thakur, B. R., and P. E. Nelson. 1997. Inactivation of lipoxygenase in whole soy flour suspension by ultrasonic cavitation. Food/Nahrung 41 (5):299–301. doi: 10.1002/food.19970410510.
- Thangavadivel, K., M. Megharaj, R. S. C. Smart, P. J. Lesniewski, and R. Naidu. 2009. Application of high frequency ultrasound in the destruction of DDT in contaminated sand and water. Journal of Hazardous Materials 168 (2-3):1380–6. doi: 10.1016/j.jhazmat.2009.03.024.
- Thanh Nguyen, T., Y. Asakura, S. Koda, and K. Yasuda. 2017. Dependence of cavitation, chemical effect, and mechanical effect thresholds on ultrasonic frequency. Ultrasonics Sonochemistry 39:301–6. doi: 10.1016/J.ULTSONCH.2017.04.037.
- Tian, Y., Z. Chen, Z. Zhu, and D. W. Sun. 2020. Effects of tissue pre-degassing followed by ultrasound-assisted freezing on freezing efficiency and quality attributes of radishes. Ultrasonics Sonochemistry 67:105162. doi: 10.1016/J.ULTSONCH.2020.105162.
- Tian, Z. M., M. X. Wan, S. P. Wang, and J. Q. Kang. 2004. Effects of ultrasound and additives on the function and structure of trypsin. Ultrasonics Sonochemistry 11 (6):399–404. doi: 10.1016/j.ultsonch.2003.09.004.
- Tolker-Nielsen, T. 2015. Biofilm development. Microbiology Spectrum 3 (2):MB. doi: 10.1128/microbiolspec.mb-0001-2014.
- Torres-León, C., M. L. Chávez-González, A. Hernández-Almanza, G. A. Martínez-Medina, N. Ramírez-Guzmán, L. Londoño-Hernández, and C. N. Aguilar. 2021. Recent advances on the microbiological and enzymatic processing for conversion of food wastes to valuable bioproducts. Current Opinion in Food Science 38:40–5. doi: 10.1016/j.cofs.2020.11.002.
- Tsikrika, K., B. S. Chu, D. H. Bremner, and M. A. Lemos. 2018. The effect of different frequencies of ultrasound on the activity of horseradish peroxidase. LWT 89:591–5. doi: 10.1016/j.lwt.2017.11.021.
- Tu, J., M. Zhang, B. Xu, and H. Liu. 2015a. Effects of different freezing methods on the quality and microstructure of lotus (Nelumbo nucifera) root. International Journal of Refrigeration 52:59–65. doi: 10.1016/j.ijrefrig.2014.12.015.
- Tu, J., M. Zhang, B. Xu, and H. Liu. 2015b. Effect of physicochemical properties on freezing suitability of Lotus (Nelumbo nucifera) root. International Journal of Refrigeration 50:1–9. doi: 10.1016/j.ijrefrig.2014.10.006.
- Vercet, A., J. Burgos, S. Crelier, and P. Lopez-Buesa. 2001. Inactivation of proteases and lipases by ultrasound. Innovative Food Science & Emerging Technologies 2 (2):139–50. doi: 10.1016/S1466-8564(00)00037-0.
- Vercet, A., J. Burgos, and P. Lopez-Buesa. 2002. Manothermosonication of heat-resistant lipase and protease from Pseudomonas fluorescens: Effect of pH and sonication parameters. The Journal of Dairy Research 69 (2):243–54. doi: 10.1017/S0022029902005460.
- Vercet, A., P. Lopez, and J. Burgos. 1997. Inactivation of heat-resistant lipase and protease from Pseudomonas fluorescens by manothermosonication. Journal of Dairy Science 80 (1):29–36. doi: 10.3168/jds.S0022-0302(97)75909-5.
- Vercet, A., C. Sánchez, J. Burgos, L. Montañés, and P. Lopez Buesa. 2002. The effects of manothermosonication on tomato pectic enzymes and tomato paste rheological properties. Journal of Food Engineering 53 (3):273–8. doi: 10.1016/S0260-8774(01)00165-0.
- Vyas, N., K. Manmi, Q. Wang, A. J. Jadhav, M. Barigou, R. L. Sammons, S. A. Kuehne, and A. D. Walmsley. 2019. Which parameters affect biofilm removal with acoustic cavitation? A review. Ultrasound in Medicine & Biology 45 (5):1044–55. doi: 10.1016/j.ultrasmedbio.2019.01.002.
- Wagh, A., P. Birkin, and S. Martini. 2016. High-intensity ultrasound to improve physical and functional properties of lipids. Annual Review of Food Science and Technology 7:23–41. doi: 10.1146/annurev-food-041715-033112.
- Wang, A., D. Kang, W. Zhang, C. Zhang, Y. Zou, and G. Zhou. 2017. Changes in calpain activity, protein degradation and microstructure of beef M. semitendinosus by the application of ultrasound. Food Chemistry 245:724–30. doi: 10.1016/j.foodchem.2017.12.003.
- Wang, A., D. Kang, W. Zhang, C. Zhang, Y. Zou, and G. Zhou. 2018. Changes in calpain activity, protein degradation and microstructure of beef M. semitendinosus by the application of ultrasound. Food Chemistry 245:724–30. doi: 10.1016/j.foodchem.2017.12.003.
- Wang, C. K., and Y. H. Shih. 2016. Facilitated ultrasonic irradiation in the degradation of diazinon insecticide. Sustainable Environment Research 26 (3):110–6. doi: 10.1016/j.serj.2016.04.003.
- Wang, D., F. Hou, X. Ma, W. Chen, L. Yan, T. Ding, X. Ye, and D. Liu. 2020. Study on the mechanism of ultrasound-accelerated enzymatic hydrolysis of starch: Analysis of ultrasound effect on different objects. International Journal of Biological Macromolecules 148:493–500. doi: 10.1016/J.IJBIOMAC.2020.01.064.
- Wang, D., X. Ma, L. Yan, T. Chantapakul, W. Wang, T. Ding, X. Ye, and D. Liu. 2017. Ultrasound assisted enzymatic hydrolysis of starch catalyzed by glucoamylase: Investigation on starch properties and degradation kinetics. Carbohydrate Polymers 175:47–54. doi: 10.1016/J.CARBPOL.2017.06.093.
- Wang, J., Q. Liu, B. Xie, and Z. Sun. 2020. Effect of ultrasound combined with ultraviolet treatment on microbial inactivation and quality properties of mango juice. Ultrasonics Sonochemistry 64:105000–11. doi: 10.1016/j.ultsonch.2020.105000.
- Wang, J., J. Wang, J. Ye, S. K. Vanga, and V. Raghavan. 2019. Influence of high-intensity ultrasound on bioactive compounds of strawberry juice: Profiles of ascorbic acid, phenolics, antioxidant activity and microstructure. Food Control 96:128–36. doi: 10.1016/j.foodcont.2018.09.007.
- Wang, N., X. Zhou, W. Wang, L. Wang, L. Jiang, T. Liu, and D. Yu. 2021. Effect of high intensity ultrasound on the structure and solubility of soy protein isolate-pectin complex. Ultrasonics Sonochemistry 80:105808. doi: 10.1016/j.ultsonch.2021.105808.
- Wu, J., T. V. Gamage, K. S. Vilkhu, L. K. Simons, and R. Mawson. 2008. Effect of thermosonication on quality improvement of tomato juice. Innovative Food Science & Emerging Technologies 9 (2):186–95. doi: 10.1016/j.ifset.2007.07.007.
- Xin, Y., M. Zhang, and B. Adhikari. 2014. Ultrasound assisted immersion freezing of broccoli (Brassica oleracea L. var. botrytis L.). Ultrasonics Sonochemistry 21 (5):1728–35. doi: 10.1016/j.ultsonch.2014.03.017.
- Xiong, G., L. Zhang, W. Zhang, and J. Wu. 2012. Influence of ultrasound and proteolytic enzyme inhibitors on muscle degradation, tenderness, and cooking loss of hens during aging. Czech Journal of Food Sciences 30:195–205.
- Xiong, T., W. Xiong, M. Ge, J. Xia, B. Li, and Y. Chen. 2018. Effect of high intensity ultrasound on structure and foaming properties of pea protein isolate. Food Research International (Ottawa, Ont.) 109:260–7. doi: 10.1016/j.foodres.2018.04.044.
- Xu, B. g., M. Zhang, B. Bhandari, X. f Cheng, and J. Sun. 2015. Effect of ultrasound immersion freezing on the quality attributes and water distributions of wrapped red radish. Food and Bioprocess Technology 8 (6):1366–76. doi: 10.1007/s11947-015-1496-x.
- Xu, B., J. Yuan, L. Wang, F. Lu, B. Wei, R. S. M. Azam, X. Ren, C. Zhou, H. Ma, and B. Bhandari. 2020. Effect of multi-frequency power ultrasound (MFPU) treatment on enzyme hydrolysis of casein. Ultrasonics Sonochemistry 63:104930. doi: 10.1016/j.ultsonch.2019.104930.
- Xu, B., M. Zhang, B. Bhandari, and X. Cheng. 2014. Influence of power ultrasound on ice nucleation of radish cylinders during ultrasound-assisted immersion freezing. International Journal of Refrigeration 46:1–8. doi: 10.1016/j.ijrefrig.2014.07.009.
- Xue, F., Z. Wu, J. Tong, J. Zheng, and C. Li. 2017. Effect of combination of high-intensity ultrasound treatment and dextran glycosylation on structural and interfacial properties of buckwheat protein isolates. Bioscience, Biotechnology, and Biochemistry 81 (10):1891–8. doi: 10.1080/09168451.2017.1361805.
- Xue, S., X. Xu, H. Shan, H. Wang, J. Yang, and G. Zhou. 2018. Effects of high-intensity ultrasound, high-pressure processing, and high- pressure homogenization on the physicochemical and functional properties of myofibrillar proteins. Innovative Food Science & Emerging Technologies 45:354–60. doi: 10.1016/j.ifset.2017.12.007.
- Yaldagard, M., S. A. Mortazavi, and F. Tabatabaie. 2007. The effects of ultrasound on the activity of alpha-amylase during barley germination. African Journal of Biotechnology 7:21–3.
- Yang, W., X. Kong, Y. Zheng, W. Sun, S. Chen, D. Liu, H. Zhang, H. Fang, J. Tian, and X. Ye. 2019. Controlled ultrasound treatments modify the morphology and physical properties of rice starch rather than the fine structure. Ultrasonics Sonochemistry 59:104709. doi: 10.1016/J.ULTSONCH.2019.104709.
- Yang, Y., J. Xiang, Z. Zhang, E. C. Umego, G. Huang, R. He, and H. Ma. 2020. Stimulation of in situ low intensity ultrasound on batch fermentation of Saccharomyces cerevisiae to enhance the GSH yield. Journal of Food Process Engineering 43 (10):e13489. doi: 10.1111/jfpe.13489.
- Yao, J. J., N. Y. Gao, C. Li, L. Li, and B. Xu. 2010. Mechanism and kinetics of parathion degradation under ultrasonic irradiation. Journal of Hazardous Materials 175 (1-3):138–45. doi: 10.1016/j.jhazmat.2009.09.140.
- Yeung, C. K., and S. C. Huang. 2017. Effects of ultrasound pretreatment and ageing processing on quality and tenderness of pork loin. Journal of Food and Nutrition Research 5 (11):809–16. doi: 10.12691/jfnr-5-11-3.
- Yi, C., Q. Lu, Y. Wang, Y. Wang, and B. Yang. 2018. Degradation of organic wastewater by hydrodynamic cavitation combined with acoustic cavitation. Ultrasonics Sonochemistry 43:156–65. doi: 10.1016/j.ultsonch.2018.01.013.
- Yu, H., Q. Zhong, Y. Liu, Y. Guo, Y. Xie, W. Zhou, and W. Yao. 2020. Recent advances of ultrasound-assisted Maillard reaction. Ultrasonics Sonochemistry 64:104844. doi: 10.1016/J.ULTSONCH.2019.104844.
- Yuan, S., C. Li, Y. Zhang, H. Yu, Y. Xie, Y. Guo, and W. Yao. 2021. Ultrasound as an emerging technology for the elimination of chemical contaminants in food: A review. Trends in Food Science & Technology 109:374–85. doi: 10.1016/j.tifs.2021.01.048.
- Yuan, X., X. Li, X. Zhang, Z. Mu, Z. Gao, L. Jiang, and Z. Jiang. 2018. Effect of ultrasound on structure and functional properties of laccase-catalyzed α-lactalbumin. Journal of Food Engineering 223:116–23. doi: 10.1016/j.jfoodeng.2017.12.008.
- Yukesh Kannah, R., J. Merrylin, T. Poornima Devi, S. Kavitha, P. Sivashanmugam, G. Kumar, and J. Rajesh Banu. 2020. Food waste valorization: Biofuels and value added product recovery. Bioresource Technology Reports 11:100524. doi: 10.1016/j.biteb.2020.100524.
- Zhang, M., Hui, H. Dong, L. Zhao, D. Xi Wang, and D. Meng. 2019. A review on Fenton process for organic wastewater treatment based on optimization perspective. The Science of the Total Environment 670:110–21. doi: 10.1016/j.scitotenv.2019.03.180.
- Zhang, M., X. Xia, Q. Liu, Q. Chen, and B. Kong. 2019. Changes in microstructure, quality and water distribution of porcine longissimus muscles subjected to ultrasound-assisted immersion freezing during frozen storage. Meat Science 151:24–32. #pagerange#. doi: 10.1016/j.meatsci.2019.01.002.
- Zhang, P., Z. Zhu, and D. W. Sun. 2018. Using power ultrasound to accelerate food freezing processes: Effects on freezing efficiency and food microstructure. Critical Reviews in Food Science and Nutrition 58 (16):2842–53. doi: 10.1080/10408398.2018.1482528.
- Zhang, X., X. Yue, B. Ma, X. Fu, H. Ren, and M. Ma. 2021. Ultrasonic pretreatment enhanced the glycation of ovotransferrin and improved its antibacterial activity. Food Chemistry 346:128905. doi: 10.1016/J.FOODCHEM.2020.128905.
- Zhang, Y., Y. Dai, H. Hou, X. Li, H. Dong, W. Wang, and H. Zhang. 2020. Ultrasound-assisted preparation of octenyl succinic anhydride modified starch and its influence mechanism on the quality. Food Chemistry: X 5:100077. doi: 10.1016/J.FOCHX.2020.100077.
- Zhang, Y., Y. Hou, F. Chen, Z. Xiao, J. Zhang, and X. Hu. 2011. The degradation of chlorpyrifos and diazinon in aqueous solution by ultrasonic irradiation: Effect of parameters and degradation pathway. Chemosphere 82 (8):1109–15. doi: 10.1016/j.chemosphere.2010.11.081.
- Zhang, Y., Z. Xiao, F. Chen, Y. Ge, J. Wu, and X. Hu. 2010. Degradation behavior and products of malathion and chlorpyrifos spiked in apple juice by ultrasonic treatment. Ultrasonics Sonochemistry 17 (1):72–7. doi: 10.1016/j.ultsonch.2009.06.003.
- Zhang, Y., W. Zhang, X. Liao, J. Zhang, Y. Hou, Z. Xiao, F. Chen, and X. Hu. 2010. Degradation of diazinon in apple juice by ultrasonic treatment. Ultrasonics Sonochemistry 17 (4):662–8. doi: 10.1016/j.ultsonch.2009.11.007.
- Zhang, Y., Z. Zhang, F. Chen, H. Zhang, and X. Hu. 2012. Effect of sonication on eliminating of phorate in apple juice. Ultrasonics Sonochemistry 19 (1):43–8. doi: 10.1016/j.ultsonch.2011.05.014.
- Zhang, Z., F. Xiong, Y. Wang, C. Dai, Z. Xing, M. Dabbour, B. Mintah, R. He, and H. Ma. 2019. Fermentation of Saccharomyces cerevisiae in a one liter flask coupled with an external circulation ultrasonic irradiation slot: Influence of ultrasonic mode and frequency on the bacterial growth and metabolism yield. Ultrasonics Sonochemistry 54:39–47. doi: 10.1016/j.ultsonch.2019.02.017.
- Zheng, T., X. Li, A. Taha, Y. Wei, T. Hu, P. B. Fatamorgana, Z. Zhang, F. Liu, X. Xu, S. Pan, et al. 2019. Effect of high intensity ultrasound on the structure and physicochemical properties of soy protein isolates produced by different denaturation methods. Food Hydrocolloids. 97:105216. doi: 10.1016/j.foodhyd.2019.105216.
- Zhong, Y., E. Bertoft, Z. Li, A. Blennow, and X. Liu. 2020. Amylopectin starch granule lamellar structure as deduced from unit chain length data. Food Hydrocolloids. 108:106053. doi: 10.1016/j.foodhyd.2020.106053.
- Zhou, G. H., X. L. Xu, and Y. Liu. 2010. Preservation technologies for fresh meat – A review. Meat Science 86 (1):119–28. doi: 10.1016/j.meatsci.2010.04.033.
- Zhou, M., J. Liu, Y. Zhou, X. Huang, F. Liu, S. Pan, and H. Hu. 2016. Effect of high intensity ultrasound on physicochemical and functional properties of soybean glycinin at different ionic strengths. Innovative Food Science & Emerging Technologies 34:205–13. doi: 10.1016/j.ifset.2016.02.007.
- Zhou, Q., Y. Bian, Q. Peng, F. Liu, W. Wang, and F. Chen. 2019. The effects and mechanism of using ultrasonic dishwasher to remove five pesticides from rape and grape. Food Chemistry 298:125007. doi: 10.1016/j.foodchem.2019.125007.
- Zhu, F. 2015. Impact of ultrasound on structure, physicochemical properties, modifications, and applications of starch. Trends in Food Science & Technology 43 (1):1–17. doi: 10.1016/j.tifs.2014.12.008.
- Zhu, Y., T. Zhang, D. Xu, S. Wang, Y. Yuan, S. He, and Y. Cao. 2019. The removal of pesticide residues from pakchoi (Brassica rape L. ssp. chinensis) by ultrasonic treatment. Food Control. 95:176–80. doi: 10.1016/j.foodcont.2018.07.039.
- Zhu, Z., Z. Chen, Q. Zhou, D. W. Sun, H. Chen, Y. Zhao, W. Zhou, X. Li, and H. Pan. 2018. Freezing efficiency and quality attributes as affected by voids in plant tissues during ultrasound-assisted immersion freezing. Food and Bioprocess Technology 11 (9):1615–26. doi: 10.1007/s11947-018-2103-8.
- Zhu, Z., P. Zhang, and D. Sun. 2019. Effects of multi-frequency ultrasound on freezing rates and quality attributes of potatoes. Ultrasonics Sonochemistry 60:104733. doi: 10.1016/j.ultsonch.2019.104733.
- Zhu, Z., P. Zhang, and D. W. Sun. 2020. Effects of multi-frequency ultrasound on freezing rates and quality attributes of potatoes. Ultrasonics Sonochemistry 60:104733. doi: 10.1016/j.ultsonch.2019.104733.
- Zou, Y., F. Lu, B. Yang, J. Ma, J. Yang, C. Li, X. Wang, D. Wang, and W. Xu. 2021. Effect of ultrasound assisted konjac glucomannan treatment on properties of chicken plasma protein gelation. Ultrasonics Sonochemistry 80:105821. doi: 10.1016/J.ULTSONCH.2021.105821.
- Zou, Y., H. Shi, P. Xu, D. Jiang, X. Zhang, W. Xu, and D. Wang. 2019. Combined effect of ultrasound and sodium bicarbonate marination on chicken breast tenderness and its molecular mechanism. Ultrasonics Sonochemistry 59:104735. doi: 10.1016/J.ULTSONCH.2019.104735.
- Zou, Y., W. Zhang, D. Kang, and G. Zhou. 2018. Improvement of tenderness and water holding capacity of spiced beef by the application of ultrasound during cooking. International Journal of Food Science & Technology 53 (3):828–36. doi: 10.1111/ijfs.13659.