Abstract
Bacteria are confronted with a multitude of stressors when occupying niches within the host. These stressors originate from host defense mechanisms, other bacteria during niche competition or result from physiological challenges such as nutrient limitation. To counteract these stressors, bacteria have developed a stress-induced network to mount the adaptations required for survival. These stress-induced adaptations include the release of membrane vesicles from the bacterial envelope. Membrane vesicles can provide bacteria with a plethora of immediate and ultimate benefits for coping with environmental stressors. This review addresses how membrane vesicles aid Gram-negative bacteria to cope with host-associated stress factors, focusing on vesicle biogenesis and the physiological functions. As many of the pathways, that drive vesicle biogenesis, confer we propose that shedding of membrane vesicles by Gram-negative bacteria entails an integrated part of general stress responses.
‘If you had to define stress, it would not be far off if you said it was the process of living. The process of living is the process of having stress and reacting to it’. Stanley J. Sarnoff.
1. Introduction
Being single cell organisms, bacteria are constantly exposed to a variety of stressors. During niche occupation within the host, stress can be caused by defense mechanisms (host-associated and/or bacterial) and by passive stress caused by the host physiology, e.g. due to a limited nutrient availability (Brogden Citation2005; Rohmer et al. Citation2011; Freestone Citation2013; Cobey Citation2014; Ribet and Cossart Citation2015). To cope with these stressors, bacteria have developed an integrated network of stress-induced pathways to mount adaptations that aid survival (Rowley et al. Citation2006). A frequently observed response to various stressors is the release of bacterial membrane vesicles from the cell envelope (Deatherage et al. Citation2009; Kulp et al. Citation2012; Schwechheimer and Kuehn Citation2015). These nanosized (10–300 nm) membrane vesicles are released from the envelope, consist of envelope lipids and proteins and contain periplasmic and cytoplasmic components. Membrane vesicles are implicated in many physiological and pathological processes as they can provide bacteria with means to acquire nutrients, aid in niche competition with other bacteria, provide protection against anti-bacterial components, and act as carriers of virulence factors (Kuehn and Kesty Citation2005; MacDonald and Kuehn Citation2012; Schwechheimer and Kuehn Citation2015). Despite its high physiological relevance, only few studies have addressed the kinetics and physiological activity of membrane vesicles directly in the context of infection. Hence, it remains poorly understood how bacteria utilize membrane vesicles to cope with the host environment. In this review, we address the question how membrane vesicles aid Gram-negative bacteria to endure host-associated stressors. To get a better insight in this, the following three questions will be addressed: (1) what are the processes involved in vesicle biogenesis, (2) how are these processes triggered by host-associated factors, and (3) how can membrane vesicles contribute to the mechanisms which are being used by bacteria to deal with host-associated stressors.
2. Biogenesis of membrane vesicles byGram-negative bacteria
Membrane vesicles are released from the bacterial envelope. Factors that compromise mechanisms for envelope maintenance, e.g. by affecting protein or lipid transport, are known to drive the formation of membrane vesicles (as reviewed in Schwechheimer and Kuehn (Citation2015)). As is shown in , the Gram-negative envelope is composed of an inner glycerophospholipid bilayer, a peptidoglycan layer, and an outer membrane composed of a glycerophospholipid and a lipooligosaccharide layer. The space that separates the outer and inner membrane is termed the periplasmic space (Silhavy et al. Citation2006; Henderson et al. Citation2016). The mechanisms responsible for membrane vesicle biogenesis can be categorized into three models.
Figure 1. Summary of the three major models for membrane vesicle formation by Gram-negative bacteria. Model A proposes that the release of membrane vesicles occurs when mechanisms for maintenance of membrane asymmetry are compromised. Model B entails mechanisms for vesicles biogenesis that are induced by accumulated misfolded or unfolded proteins. Model C is based on the formation of membrane vesicles as the result of LPS modifications triggered by intrinsic or extrinsic factors. For each of the models the most important factors shown to be involved in vesicle formation are indicated as well as how they are involved in phospholipid (Model A) or misfolded protein (Model B) trafficking. The gray spheres represent the stress factors that have been shown to trigger vesicle formation. This figure was created using Servier Medical Art.
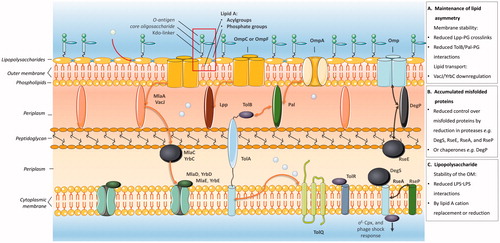
Model A proposes that vesicle formation is triggered when mechanisms for the maintenance of lipid asymmetry are compromised ( Model A).
Model B implies that membrane vesicle formation is induced by the accumulation of misfolded or unfolded proteins in the outer membrane ( Model B).
Model C entails that membrane vesicles are formed because of lipopolysaccharide (LPS) modifications induced by intrinsic and extrinsic factors ( Model C).
The most important aspects of these models will be covered in the following paragraphs, as a starting point to assess how vesicle biogenesis is affected by host-associated factors.
2.1. Model A: vesicle biogenesis induced by a compromised maintenance of envelope lipid asymmetry
Envelope stability is established by carefully organized lipid interactions and the maintenance of envelope lipid asymmetry (Silhavy et al. Citation2006; Henderson et al. Citation2016). These interactions take place between lipids of the outer membrane and the peptidoglycan layer, the peptidoglycan layer and the Tol-Pal complex, and the peptidoglycan layer and outer membrane proteins (Omps) such as Omp A and Braun’s lipoprotein (lpp), as is shown in . Modifications which affect lipid interactions in the envelope will result in the release of membrane vesicles as has been demonstrated elegantly by Deatherage et al. in Salmonella typhimurium (Deatherage et al. Citation2009). Moreover, they demonstrated that modifications, which reduce the number of outer membrane-peptidoglycan interactions during division, lead to an increased membrane vesicle formation (Deatherage et al. Citation2009). Also, decreased interactions between membrane lipids and peptidoglycan-associated proteins in the envelope have been shown to increase vesiculation. This is supported by several lines of evidence. First, the deletion of genes that encode either one of the peptidoglycan-associated proteins Oprl (the homolog of lpp), OmpA, Pal or TolA in Pseudomonas aeruginosa resulted an increased vesicle formation (Schooling and Beveridge Citation2006; Wessel et al. Citation2013). Similarly, TolA, TolQ, and Tol/Pal deletion mutants of Escherichia coli are characterized by an increased vesicle formation (Bernadac et al. Citation1998; Balsalobre et al. Citation2006; Pietras and Jagusztyn-krynicka Citation2009). Moreover, OmpA deletion mutants of E. coli, Salmonella spp., Vibrio cholera, and Acinobacter baumanni also show an increased vesicle release (Sonntag et al. Citation1978; Song et al. Citation2008; Deatherage et al. Citation2009; Moon et al. Citation2012).
Other factors that have been shown to be involved in the maintenance of the envelope lipid asymmetry are components of ABC-transporters such as the VacJ/YrbC-transporter (Silhavy et al. Citation2006; Henderson et al. Citation2016). Recently, it has been shown in a study by Roier et al. that deletion or downregulation of the VacJ/YrbC-ABC transporter results in the formation of membrane vesicles by Haemophilus influenzae and V. cholerae (Roier et al. Citation2016). For E. coli, this VacJ/YrbC-transporter homolog is known as the Mla (Maintance of outer membrane lipid asymmetry) transporter (Malinverni and Silhavy Citation2009). Studies on E. coli mutants with deletions in genes that encode Mla pathway proteins confirm that this transporter is involved in the formation of membrane vesicles (Roier et al. Citation2016). Further evidence that a compromised functionality of this transporter enhances vesiculation is provided by Martorana et al. who showed that deletion or repression of components of the Mla transporter results in outer membrane phospholipid accumulation (Martorana et al. Citation2014), which is a well-known inducer for vesicle formation (Schwechheimer and Kuehn Citation2015; Roier et al. Citation2016).
2.2. Model B: vesicle biogenesis induced by accumulated envelope components and extra-cytoplasmic stress responses
Extra-cytoplasmic stress responses collectively describe mechanisms which respond to stress in systems located in the cell envelope, but outside the cytoplasm (Rowley et al. Citation2006; Ruiz et al. Citation2006). To date, three important stress responses have been shown to affect the release of membrane vesicles: (1) the sigma factor E (σE) response, (2) the two-component regulator Cpx response, and (3) the phage shock response (McBroom and Kuehn Citation2007).
The σE and Cpx pathways, which are both activated by misfolded outer membrane proteins, generally contribute to bacterial pathogenesis and are required for the generation of resistance to a wide variety of stressors (Rowley et al. Citation2006). Genes under the control of σE and Cpx partially overlap and encode proteins that are involved in the degradation and folding of envelope polypeptides (e.g. proteases and chaperones) and proteins involved in the formation and modulation of LPS. For the σE pathway, impairments have been shown to result in the accumulation of misfolded outer membrane proteins and an increased vesicle release. This was observed for E. coli and P. aeruginosa where mutations in RpoE (which encodes the σE) and AlgU (that encodes the P. aeruginosa σE homolog, sigma factor H) promoted vesicle release (McBroom and Kuehn Citation2007; MacDonald and Kuehn Citation2013). In E. coli, increased vesiculation was also observed upon deletion of the periplasmic sensor proteases RseA, DegS, and RseP which are involved in the recognition of unfolded/misfolded or accumulated free outer membrane proteins (Alba et al. Citation2002; McBroom et al. Citation2006; Sohn et al. Citation2009; Kim Citation2015). Other important components of the σE pathway are the protease/chaperone proteins known as DegP (E. coli) and MucD (P. aeruginosa). DegP and MucD mutants strains proved unable to control the amount of unfolded membrane proteins and exhibited an increased vesiculation (Mogensen and Otzen Citation2005; McBroom and Kuehn Citation2007; Tashiro et al. Citation2009).
Vesicle formation is also affected by the Cpx stress response in a similar fashion as the σE response as deletion mutants of Cpx showed an increased vesicle formation which implies this pathway inhibits processes that induce vesiculation (McBroom and Kuehn Citation2007).
The release of membrane vesicles can also be affected by other extra-cytoplasmic stress responses such as the phage shock response that confers protection against e.g. hydrophobic organic solvents, ethanol, high temperature, a high pH, and phage infection (Rowley et al. Citation2006). For example, exposure of S. typhimurium to 10% ethanol or E. coli to an increased temperatures or T4-bacteriophage infection have all been shown to lead to increased vesicle release (Loeb and Kilner Citation1978; McBroom and Kuehn Citation2007; Manning and Kuehn Citation2011).
To conclude model B proposes a model for vesiculation in response to stressors that induce the accumulation of envelope components and/or as a consequence of inadequate coping with extra-cytoplasmic stress responses leading to accumulated envelope components.
2.3. Model C: vesicle biogenesis induced by factors that interact with or induce LPS modifications
The stability of the outer membrane is critically determined by interactions between LPS molecules on the surface of the outer membrane. The number and strength of these LPS-LPS interactions relies on the structure of LPS. Typically, LPS is composed of three domains: (1) lipid A that is anchored in the outer membrane, (2) the core domain that is linked to lipid A by the 3-deoxy-D-manno-oct-2-ulosonic acid (Kdo), and (3) the O-antigen (see Model C). The core domain and O-antigen protrude into the extracellular environment.
LPS-LPS interactions are based on salt bridges formed by cations (e.g. Mg2+ and Ca2+) between the terminal phosphates located at the lipid A domain, and anionic sites on the core region of LPS (Alexander and Rietschel Citation2001). External factors that affect these salt bridges and the LPS phenotype or conformation have been shown to affect the vesicle production. It has been posed that the mechanism by which this occurs depends on an increased membrane bending as the result of a) charge repulsion between two LPS moieties (Mashburn-Warren and Whiteley Citation2006; Mashburn-Warren et al. Citation2008; MacDonald and Kuehn Citation2013) and/or b) the incorporation of certain external factors into the outer membrane (Mashburn-Warren et al. Citation2008; Schertzer and Whiteley Citation2012).
Charge repulsion depends on the orientation and composition of LPS (e.g. lipid A phosphorylation status and composition of the O-antigen) and can be neutralized by the formation of salt bridges (Kadurugamuwa and Beveridge Citation1997; Mashburn-Warren et al. Citation2008). Moreover, the acylation status can also affect the release of membrane vesicles. Elhenawy et al. proposed that this is mediated by the conformational change of LPS from cone-shaped towards a more cylindrical shape. Consequently, the negative charged groups (e.g. the phosphate groups on lipid A) are then brought in a closer proximity, resulting in charge repulsion and increased membrane bending (Elhenawy et al. Citation2016). The factors that have been shown to induce the vesiculation as well as the consequences of the LPS/LOS composition will be discussed below in more detail.
External factors that act on LPS are cationic antimicrobial peptides and cations such as Mg2+. The cationic antimicrobial peptides will be addressed in the paragraph on antimicrobial peptide-induced vesicle release (vide infra). Cations like Mg2+ have been shown to support the stability of outer membrane and addition of MgCl2 to E. coli cultures was found to repress the production of membrane vesicles (Tashiro et al. Citation2010). Moreover, it has been shown that the LPS phenotype has a major impact on the formation of membrane vesicles. For P. aeruginosa, it has been shown that there are two types of LPS expressed, namely a short form (A-band LPS) and a longer form (B-band LPS) that has a high negative charge. This length and charge are determined by the O-antigen (Lam et al. Citation2011). Strikingly, only the B-band LPS was found to be present in naturally formed membrane vesicles. Furthermore, P. aeruginosa strains that only expressed the A-band LPS showed a diminished vesicle release (Haurat et al. Citation2015). For S. typhimurium and E. coli it has been shown that LPS lipid A modifications are controlled by the two-component system PhoPQ (Guo et al. Citation1997; Groisman Citation2001). This PhoPQ system is conserved in many Gram-negative bacterial species and senses low extracellular levels of Mg2+ and antimicrobial peptides such as polymyxin B (as reviewed by Groisman (Groisman Citation2001)). Direct evidence for the involvement of PhoPQ in vesicle biogenesis was provided by studies that demonstrated an increased vesicle formation by S. typhimurium and E. coli strains which overexpress the PhoPQ-induced components PagL and PagC (enzymes that de-acylate lipid A), respectively (Kitagawa et al. Citation2010; Elhenawy et al. Citation2016). Importantly, this was shown to occur independently of the σe and Cpx stress responses (Elhenawy et al. Citation2016).
An extensive assessment of the outer membrane vesicle release by E. coli identified many enzymes of the LPS biogenesis pathway to be involved in the formation of membrane vesicles (Kulp et al. Citation2015). Several studies show an increased vesiculation on PagL overexpression: PagL is known to remove a secondary acyl group from the 3th position (Brozek and Raetz Citation1990; Kawasaki et al. Citation2004; Trent Citation2004; Elhenawy et al. Citation2016). This is in line with the proposed model that the de-acylation pattern of lipid A alters the orientation of lipid A, which results in an increased membrane curvature (Schromm et al. Citation2000; Zimmerberg and Kozlov Citation2006; Elhenawy et al. Citation2016). However, as was suggested by Elhenawy et al. conditions that result in activation of PagL by PhoPQ perhaps also lead to the activation of other lipid A modifying enzymes. So, although de-acylation itself can result in an increased membrane vesicle release, it is possible that these effects are cancelled-out by other lipid A modifications. Moreover, de-acylated lipid A (LpxM deletion mutants) or monophosphorylated penta-acylated mutants (LpxM/LpxF double deletions mutants) did not show or only showed a slightly increased vesiculation as compared to wild-type bacteria ((Chen et al. Citation2016; Lee et al. Citation2016) and personal communication with Dr. SH. Kim and Prof. M. DeLisa, respectively).
Bacteria can modulate their LPS in response to their environment, which include changes in temperature, pH, MgCl2, NaCl, phosphate, sucrose concentrations, oxygen stress, and exposure to antimicrobial peptides (Kropinski et al. Citation1987; McGroarty and Rivera Citation1990; Makin and Beveridge Citation1996; Sabra et al. Citation2003; Henderson et al. Citation2016). Moreover, LPS modifications can also result from the activation of other pathways that affect vesiculation, such as a compromised functioning of the Tol-Pal system or ABC transporters as these mechanisms are involved in the biogenesis and/or transport of lipopolysaccharide components (Gaspar et al. Citation2000; Vines et al. Citation2005; Pietras and Jagusztyn-krynicka Citation2009). Therefore, it will be highly relevant to determine how the bacterial 'vesiculation' phenotype is shaped by the environment, not only with a focus on the LPS phenotype but also on outer membrane characteristics that comprise the accumulation of misfolded proteins and the maintenance of the lipid asymmetry.
3. Bacterial vesicle release in response tohost-associated environmental factors
The previous paragraphs summarized various studies in which deletion mutants were used to provide insights in processes at the bacterial envelope that drive the formation of membrane vesicles. The next step will be to determine how this can be integrated into physiological conditions. Essential progress is made by studies that performed transcriptome analysis of bacteria upon exposure to environmental stressors that are known to induce the release of membrane vesicles. When gene expression profiles of Neisseria meningitidis in response to several stressors were assessed, high resemblance was observed between the gene expression profiles of cysteine-deprived bacteria, bacteria exposed to oxidative stress, heat shock or iron depletion, and bacteria during interaction with host-cells (van de Waterbeemd et al. Citation2013). This indicates that pathways induced by these stressors congregate and that the formation of outer membrane vesicles can form an integrated part of general stress responses. In the following paragraphs, we will address how host-related factors such as nutrient availability and antibacterial components affect the membrane vesicle release (summarized in ).
Table 1. Host-cell associated stress factors that have been shown to induce membrane vesicle release.
3.1. Nutrient deprivation
A study by van de Waterbeemd et al. demonstrated that cysteine depletion induces growth phase transition from the logarithmic into the stationary phase and that this results in an upregulation of genes related to oxidative stress including the iron-sulfur cluster, metal ion uptake, and stress (van de Waterbeemd et al. Citation2013). Based on this, the authors proposed that cysteine depletion impairs the sulfur supply required to produce iron-sulfur complexes and that this results in oxidative stress and the formation of membrane vesicles. Nutrient depletion in general causes oxidative stress which results in the induction of extra-cytoplasmic stress responses (Model B) (Rowley et al. Citation2006). It is possible that oxidative stress affects membrane vesicle formation by modulation of the LPS biosynthesis (Model C) as the oxidative stress induced genes OxyR and SoxRS (Cabiscol et al. Citation2000) were found to induce the expression of genes involved in lipid A and peptidoglycan biosynthesis, metal ion transport, and amino-acid biosynthesis (Seo et al. Citation2015). Moreover, it was found that iron-limiting conditions induce an increased vesicle biogenesis by downregulating the VacJ/Yrb components of the VacJ/Yrb ABC transporter (Roier et al. Citation2016). Thus, nutrient deprivation can also affect processes involved in the maintenance of the lipid asymmetry (Model A). Oxidative stress is also induced by other physiological conditions than nutrient deprivation, therefore vesiculation induced by oxidative stress will be discussed in more detail in the section on physiological stressors. Whether environmental stressors trigger one common mechanism for membrane vesicle formation or whether there are different processes on the membrane that account for vesicle formation is still largely unknown.
3.2. Antimicrobial peptides and antibiotics
Increased membrane vesicle formation in response to antimicrobial peptides and antibiotics is a well-described phenomenon. The antimicrobial peptides are classified and addressed here as bacteria-derived (i.e. quinolines, quinolones, and polymyxins: cationic antibiotics produced by Paenibacillus polymyxa), host-associated (i.e. lysozyme), or cationic antibiotics (i.e. aminoglycosides). Generally, the peptides and the antibiotics that trigger the formation of membrane vesicles have been shown to be cell envelope-directed, to induce the SOS response (oxidative stress), to alter the LPS structure, or a combination of the above (Kohanski et al. Citation2010). Therefore, they can trigger the vesicle formation by one of the three models described above (A: lipid asymmetry maintenance, B: extra-cytoplasmic stress responses or C: LPS modification).
Bacteria-derived antimicrobial peptides, that have been shown to induce the release of membrane vesicles, are quinolones, quinolines, and polymyxins. Quinolones and quinolines are part of the quorum sensing system which allows bacteria to communicate and coordinate group actions. The antimicrobial quinoline PQS is the most important quorum sensing signal of P. aeruginosa and a potent inducer of membrane vesicle formation (Mashburn-Warren et al. Citation2008; Tashiro et al. Citation2010; MacDonald and Kuehn Citation2013). Interestingly, it has been demonstrated that PQS can induce its own release and the release of other quinolines and quinolones in a membrane vesicle-mediated way (Mashburn and Whiteley Citation2005). It seems likely that these signaling molecules can subsequently also trigger the release of additional/other membrane vesicles as several quinolones are structurally similar to PQS (Lecomte et al. Citation1994; Marshall and Piddock Citation1994; Mashburn-Warren et al. Citation2008). Despite this high similarity, at present only PQS is known to trigger the release of membrane vesicles and PQS was required and sufficient for membrane vesicle formation (Mashburn and Whiteley Citation2005). The mechanism behind this PQS-induced formation of membrane vesicles depends on the interaction of PQS with the lipid A region of LPS. The current hypothesis is that it increases the formation of membrane vesicles by disrupting the Mg2+ and Ca2+ salt bridges between the LPS molecules and by insertion into the outer membrane (Schertzer and Whiteley Citation2012; MacDonald and Kuehn Citation2013). Although PQS is only produced by P. aeruginosa were it forms an intrinsic factor that stimulates the formation of membrane vesicles (Mashburn and Whiteley Citation2005; Mashburn-Warren et al. Citation2008), it has been demonstrated that exogenously added PQS can also serve as an extrinsic factor for the formation of membrane vesicles by other Gram-negative bacteria (Mashburn and Whiteley Citation2005; Mashburn-Warren et al. Citation2008; Tashiro et al. Citation2010). Moreover, it also has to be noted that PQS can induce the formation of membrane vesicles by Gram-positive bacteria and even red blood cells, suggesting that PQS can also induce the release of membrane vesicles by alternative mechanisms (Schertzer and Whiteley Citation2012; Tashiro et al. Citation2010).
Polymyxin B and colistin are bacterial antimicrobial peptides that target the outer membrane by replacing Mg2+ and Ca2+ cations thereby destabilizing LPS-LPS bonds (Moore and Hancock Citation1986). As indicated earlier, such destabilization is a well-known stimulus for vesicle release. In line with this, MacDonald et al. reported that treatment of P. aeruginosa with polymyxin B resulted in an increased vesicle release (MacDonald and Kuehn Citation2013). Also, the exposure of E. coli to polymyxin B and colistin enhanced the membrane vesicle release (Manning and Kuehn Citation2011). Importantly, host-associated environmental factors may also determine the sensitivity to vesiculation for example by the activation of two-component regulators. The most prominent two-component regulator by which this has been shown to occur is PhoPQ (Poole Citation2012). As mentioned earlier binding of antimicrobial peptides by S. typhimurium and E. coli can be sensed by the PhoPQ and PhoPQ deletion mutants show an increased sensitivity to antimicrobial peptides (Guo et al. Citation1997; Trent et al. Citation2001; Dalebroux et al. Citation2014). Moreover, it was shown for S. typhimurium that low Mg2+ levels activate PhoPQ which was consequently found to promote resistance to these peptides (Guo et al. Citation1997; Groisman Citation2001; Shi et al. Citation2004). Although, as the body’s divalent cation levels are as high as 1–2 mM, it was thought that this system was not induced in the host (Fernández et al. Citation2010). A study based on S. typhimurium showed that high cation levels repress the PhoPQ system and that antimicrobial peptides, even in the presence of these cations, induce an activation (Bader et al. Citation2005). Moreover, for P. aeruginosa it has been shown that this PhoPQ system is upregulated on contact with epithelial cells (within the presence of cations) and that this resulted in PhoPQ dependent modifications of lipid A (Gellatly et al. Citation2012). To conclude, the host environment can result in the upregulation of PhoPQ and PagL dependent lipid A modifications leading to a decreased susceptibility to antimicrobial peptides. Although no direct link between vesiculation and host environment upregulation of PhoPQ has been made, it is tempting to speculate that the host environment can hereby affect the sensitivity to vesiculation.
At present the best studied host-associated antimicrobial peptide involved in membrane vesicles release is lysozyme. Lysozyme is released among others by epithelial cells and is found within the phagosome of phagocytes where it aids in bacterial degradation. Several studies have shown that exposure of P. aeruginosa and Francisella novicida to lysozyme not only results in cell lysis but also in the release of outer membrane vesicles. It acts as an antimicrobial substance by mimicking cationic antimicrobial peptides by its enzymatic muramidase activity (McCaig et al. Citation2013; Metruccio et al. Citation2016). As a muramidase, it interacts with the peptidoglycan structure and hydrolyzes the glycosidic bond between the N-acetylmuramic acid and N-acetylglucosamine residues of the peptidoglycan backbone. Additionally, lysozymes’ cationic peptide can also disrupt the outer membrane (Marshall and Piddock Citation1994; Davis and Weiser Citation2011). And although there is currently no consensus on the mechanism by which this occurs, it has been suggested that lysozyme induces the release of membrane vesicles in a similar fashion as gentamicin by the disruption of LPS-LPS bonds, as will be described below (Metruccio et al. Citation2016).
Several antibiotics, like gentamicin, amikacin, ciprofloxacin, mitomycin C and D-cycloserin have also been shown to induce vesicle formation. Gentamicin is known to inhibit the bacterial protein synthesis and the consensus is that it gains access to the cell via holes in the outer membrane that are created by membrane destabilization. This membrane destabilization occurs when gentamicin binds sites on LPS that are normally occupied by the Mg2+ and Ca2+ cations. This subsequently destabilizes the salt bridges between the LPS molecules resulting in disruptions of the outer membrane and results in an increased vesicle formation (Martin and Beveridge Citation1986). Moreover, treatment with another aminoglycoside amikacin has also been shown to induce vesiculation as it triggers the formation of blebs on the outer membrane by destabilization of LPS-LPS bonds (Walker and Beveridge Citation1988). Treatment of P. aeruginosa with ciprofloxacin, an antibiotic that leads to DNA damage and the activation of the SOS stress response, also enhanced the release of membrane vesicles (Maredia et al. Citation2012). This SOS stress response was required for the vesicle release as a mutant strain deficient in a protein critical for the induction of this stress response, showed a compromised vesicle release. A similar phenomenon is observed for several other antibiotics including mitomycin C and D-cycloserin. Mitomycin C is also thought to activate the SOS stress response in Shigella dysenteriae and has been shown to induce vesiculation (Kogoma et al. Citation1979; Dutta et al. Citation2004; Gray et al. Citation2014).
Bacterial modification of the outer membrane in response to environmental factors such as antimicrobial peptides is an important strategy for survival. For example, a lipid A de-acylation is known to lead to a decreased susceptibility to cationic antimicrobial peptides. Also, some commensal bacteria that express the lipid A phosphatase LpxF displayed an enhanced resistance to antimicrobial peptides (Cullen et al. Citation2015). Therefore, to fully understand how environmental factors affect the release of membrane vesicles, it will be of the essence to link how bacterial modification of the outer membrane associates with the release of membrane vesicles.
3.3. Physiological stressors
Besides the stressors mentioned above, it has been reported that physiological barriers such as oxidative stress, temperature, and oxygen tension can trigger stress responses and the release of bacterial membrane vesicles.
Oxidative stress has been shown to be a potent inducer of membrane vesicle release as hydrogen peroxide triggered the membrane vesicle release from N. meningitis (van de Waterbeemd et al. Citation2013) and P. aeruginosa (MacDonald and Kuehn Citation2013). As was mentioned above in the paragraph on nutrient deprivation, the exact mechanism behind this is still unclear. Yet it is known that oxidative stress, caused e.g. by the release of reactive oxygen species by host immune cells (Nathan and Cunningham-Bussel Citation2013), can trigger the extra-cytoplasmic stress response and may affect the biogenesis of LPS (Rowley et al. Citation2006). Also, it is well-known that changes in temperature can alter the lipid composition and thereby affect the release of membrane vesicles (Sabra et al. Citation2002). This has been shown to occur in a species-dependent manner. For example, in E. coli exposure to increasing temperatures increased vesiculation (McBroom and Kuehn Citation2007), while in P. aeruginosa the release of membrane vesicles was not affected by changes in temperature, although the composition of the vesicles changed under these conditions (Makin and Beveridge Citation1996; MacDonald and Kuehn Citation2013).
Another important factor that can affect the release of outer membrane vesicles is oxygen stress. Sabra et al. show that oxygen limitation markedly reduced the release of extracellular proteins by P. aeruginosa (Sabra et al. Citation2002). In contrast, increased oxygen tension enhanced the vesicle release and the vesicle B-band LPS content of the vesicles (Sabra et al. Citation2003).
3.4. Other host-associated factors
Finally, it has been reported that neuropeptides can also trigger the release of bacterial membrane vesicles which has been demonstrated for Salmonella enterica serovar Typhi upon exposure to norepinephrine (Karavolos et al. Citation2011). As norepinephrine is already known to increase bacterial pathogenicity including by the enhancement of bacterial adhesion, motility and toxin release (Lyte et al. Citation1996; Lyte et al. Citation1997; Vlisidou et al. Citation2004; Nakano et al. Citation2007; Bearson and Bearson Citation2008), it is possible that the release of membrane vesicles in response to norepinephrine contributes to this pathogenicity. Bacteria mostly come in contact with epinephrine and norepinephrine in the bloodstream and in the gut respectively (Hughes and Sperandio Citation2008; Boyanova et al. Citation2017), but it also has been shown that macrophages produce these peptides (Flierl et al. Citation2009).
4. Physiological roles of bacterial membrane vesicles
During exposure to acute or chronic environmental stressors, early sensing of and adaptation to these stressors are key for bacterial survival. The release of membrane vesicles has been shown to be sensitive to various stressors that can be encountered during infection as part of the host response or during niche occupation. In the next section, we will discuss several ways how bacterial membrane vesicles can provide bacteria with a competitive advantage when exposed to acute and chronic host-associated stressors.
4.1. Protection against innate and adaptive immunity
Bacterial invasion of the host initiates an immune response that generally involves the innate immune and complement system and may engage the adaptive immune system (Manning and Kuehn Citation2011). The physiological activity of membrane vesicles in this respect either consists of providing direct protection or by suppression of the immune response. An example of bacterial membrane vesicle-mediated protection against host factors is the protection of Porphyromonas gingivalis and Neisseria gonorrhoeae from human serum which was established by absorption of bactericidal factors (Grenier and Bélanger Citation1991; Pettit and Judd Citation1992; Grenier et al. Citation1995). Moreover, it has been demonstrated for a variety of bacteria that their membrane vesicles can also protect against complement (Tan et al. Citation2007). For example, protection against complement factor C3 can be mediated by the outer membrane protein P5, which can be found in membrane vesicles from H. influenzae (Sharpe et al. Citation2011; Rosadini et al. Citation2014). Protection against complement factor C3 has also been demonstrated for lipooligosaccharide and ubiquitous surface protein Usp A1/A2 on M. catarrhalis vesicles (Nordström et al. Citation2005; Tan et al. Citation2007), and IgG binding protein SbI on vesicles from S. aureus (Burman et al. Citation2008). Moreover, Vidakovics et al. demonstrated that M. catarrhalis also utilizes membrane vesicles to escape from adaptive immunity. This study shows that membrane vesicles can directly prevent the uptake and destruction of M. catarrhalis by tonsillar B cells and that this occurs in a superantigen MID dependent fashion (Perez Vidakovics et al. Citation2010).
It is well-known that bacteria suppress the immune response by modifying the LPS lipid A composition towards a less immunogenic/immunosilent form to promote bacterial survival during host niche occupation or invasion (Raetz et al. Citation2007; Needham et al. Citation2013). Interestingly, there seems to be a link between the LPS alterations that lead to this reduced immunogenicity and the release of membrane vesicles. The consequences for the physiological activity will be discussed below.
LPS is a major constituent of the bacterial envelope and of membrane vesicles. The structure of LPS critically determines whether it can be considered a virulence factor/toxin or whether is involved in the establishment and maintenance of immune homeostasis (Alexander and Rietschel Citation2001; Netea et al. Citation2002; Montminy et al. Citation2006; Munford and Varley Citation2006). Important herein are the number of acyl chains and the phosphate groups on the lipid-A region of LPS. Hexa-acylated lipid A is known to exert the strongest agonistic activity and penta- or tetra-acylated in general have a lower agonistic activity but may even have an antagonistic activity (Steimle et al. Citation2016).
It has been shown that both the background as well as the environment are determinants for the structure of the LPS (Guo et al. Citation1998; Alexander and Rietschel Citation2001; Beutler and Rietschel Citation2003; Park et al. Citation2009; Maeshima and Fernandez Citation2013). S. typhimurium mutants that over-express PagL exhibit a phenotype that is characterized by an increased production of vesicles which are highly enriched in de-acylated lipid A (Elhenawy et al. Citation2016). Although it was not tested in this study, it is highly likely that the acylation phenotype has consequences for the immunogenicity of these vesicles, as de-acylated lipid A has strongly reduced immunostimulatory properties (Kawasaki et al. Citation2004; Kawano et al. Citation2010). Besides the acylation pattern, the phosphate groups on lipid A also determine the immunogenicity of LPS (Steimle et al. Citation2016). Most lipid A structures contain two phosphate groups, which facilitate cation bridges and determine the stability of the outer membrane by facilitating these LPS-LPS bonds (Marshall and Piddock, Citation1994; Mashburn-Warren and Whiteley Citation2006; Mashburn-Warren et al. Citation2008). But as these groups also render bacteria susceptible to cationic antimicrobial peptides, a deletion or loss can therefore protect bacteria from these peptides. A deletion or loss of phosphate groups has also been shown to weaken the agonistic properties of LPS (Guo et al. Citation1998; Matsuura Citation2013; Needham and Trent Citation2013; Cullen et al. Citation2015). At present it is known that 4’dephosphorylated-penta-acylated lipid A under natural conditions only leads to a slightly increased vesiculation (as was described above in ‘Model C’) ((Chen et al. Citation2016; Lee et al. Citation2016), and personal communication Dr. SH.Kim and Prof. M. DeLisa, respectively).
This is also reflected by the LPS phenotype of commensals. To achieve intestinal immune homeostasis a microbiota composition that is characterized by a low LPS endotoxicity is required (Lebeer et al. Citation2010; Cullen et al. Citation2015; Steimle et al. Citation2016; Vatanen et al. Citation2016). A highly relevant study in this context is a study by Cullen et al. that shows that intestinal commensal bacteria accomplish this by LpxF mediated lipid A dephosphorylation (Cullen et al. Citation2015). Mass-spectrometric analysis established that the lipid A of human gut bacteria typically is 4’dephosphorylated-penta-acylated (Cullen et al. Citation2015). That this is reflected in lipid A composition of membrane vesicles from commensals is supported by a study by Elhenawy et al. which demonstrates that membrane vesicles from the common human gut commensal Bacteroides thetaoitaomicron were characterized by a 4’dephosphorylated penta-acylated lipid A moiety (Elhenawy et al. Citation2014). This implies a similar LPS phenotype for the outer membrane and outer membrane vesicles. Finally, it is key to mention that this lipid A phenotype also renders a phenotype that is highly resistant to cationic microbial peptides, suggesting that this phenotype may also affect the susceptibility to antimicrobial peptide induced vesiculation.
As membrane vesicles which contain a less immunogenic LPS may have therapeutic vaccine potential, the immune responses to these ‘detoxified’ membrane vesicles have been studied extensively (Baker et al. Citation2014; Henderson et al. Citation2016). Studies have shown that membrane vesicles with penta-/tetra-acylated and/or mono-/dephosphorylated LPS are characterized by a reduced toxicity (as compared to hexa-acylated bisphosphorylated LPS) (van de Waterbeemd et al. Citation2010; Ho et al. Citation2011; Baker et al. Citation2014; Moreno and Rumbo Citation2014; Lee et al. Citation2016). An elegant study by Kang et al. demonstrated that this may also have implication for regulation of immunity by commensals. This study, that characterized both the gut microbiome and the vesicle composition, showed that not all commensals release membrane vesicles and that the release of these vesicles predominantly occurs by bacteria that fall under the genera Bacteroidetes, Akkermansia, and Bifidobacteria. But more importantly they also show that membrane vesicles from the commensal Akkermansia muciniphila can provide protection against inflammation induced by E. coli-derived membrane vesicles and against DSS-induced colitis (Kang et al. Citation2013).
To conclude, structural alterations such as a decreased lipid A acylation or the loss of phosphate groups may render a phenotype that releases vesicles with weaker agonistic or even antagonistic properties that may serve to protect from immune recognition. Future research will be required (1) to assess the vesicle release by commensals and pathogens and (2) to determine whether vesicles from commensals are more immunoquiescent in character.
4.2. Protection from antimicrobial peptides and antibiotics
Within the host niche there may be a high level of competition amongst bacteria, and bacteria have adopted various strategies which aid in this competition. The release of bacterial antimicrobial peptides (bacteriocins) (Hibbing et al. Citation2010), including quinolones and quinolines is one of them. As was mentioned above, the quinoline PQS is a potent inducer for the formation of membrane vesicles. As PQS is known for its bacteriolytic properties, it has been suggested that PQS binding triggers the formation of membrane vesicles to ‘shake off’ PQS and other bacteriocins to prevent further damage. Although little is known on how membrane vesicle directly protect bacteria from the harmful effects of antimicrobial peptides, this theory is supported by data presented by Marsland and colleagues (Citation2014) who showed that upon the addition of the PQS, 60–80% of this peptide was immediately removed by membrane vesicle formation (Mashburn and Whiteley Citation2005).
Direct membrane vesicle-mediated protection against antimicrobial peptides and antibiotics has been demonstrated for polymyxin B and colistin (Manning and Kuehn Citation2011; MacDonald and Kuehn Citation2013). Manning et al. demonstrated that treatment of E. coli with polymyxin B and colistin induced the release of membrane vesicles which subsequently protected bacteria by adsorption of the peptides (Manning and Kuehn Citation2011). As it has been reported that antimicrobial peptides can bind the lipid A region of LPS it is highly likely that this adsorption occurs by the binding to membrane vesicle-associated LPS (Brogden Citation2005; Steimle et al. Citation2016). This is supported by the finding that vesicles from the E. coli ETEC strain, which contains an altered LPS structure rendering it resistance to polymyxin B, were unable to provide polymyxin B-sensitive strains with membrane vesicle-dependent protection (Manning and Kuehn Citation2011). Bacterial vesicles can also transfer enzymes which protect against antibiotics as has been shown in a study by Schaar et al. (Citation2011). They showed that exposure of M. catarrhalis to amoxicillin induces the release of β-lactamase-containing membrane vesicles that yielded protection against amoxicillin. The packing of β-lactamase into membrane vesicles was also observed for P. aeruginosa and Streptococcus aureus (Ciofu et al. Citation2000; Lee et al. Citation2009; Schaar et al. Citation2011).
To conclude, at present it is recognized that membrane vesicles can provide protection against several antimicrobial peptides and antibiotics.
4.3. Nutrient acquisition
Besides active defense strategies there are also other properties of membrane vesicles that contribute to bacterial survival and overcome host defense strategies to prevent bacterial niche occupation. A potent host strategy herein is the creation of an environment devoid of crucial nutrients such as iron. In response, bacteria have developed several ways to obtain iron from their environment (as reviewed by Schaible et al. (Schaible and Kaufmann Citation2004)). However, as discussed before, iron and cysteine starvation are two well-known factors that trigger bacterial membrane vesicle release. Interestingly, there is compelling evidence demonstrating that membrane vesicles can contain factors (e.g. siderophores) that can bind iron and aid in the acquisition of iron. Membrane vesicles of N. meningitis have been shown to carry proteins for iron binding and uptake (Lappann et al. Citation2013). The presence of several proteins involved in the acquisition of iron has also been demonstrated for Moraxella membrane vesicles and these vesicles have been found to carry i.e. transferrin-binding protein B and CopB (Aebi et al. Citation1996; Schaar et al. Citation2011). Moreover, P. gingivalis-derived membrane vesicles have been shown to contain the heme binding protein FetB and several gingipains which are also involved in iron acquisition (Veith et al. Citation2014). The release of siderophore-containing membrane vesicles has been demonstrated for Klebsiella pneumoniae, P. aeruginosa and Mycobacterium tuberculosis (Prados-Rosales et al. Citation2011; Lee et al. Citation2012; Maredia et al. Citation2012).
5. Conclusion: different threats, same trick
Bacteria are constantly exposed to a variety of stressors and many of them are now known to trigger the release of membrane vesicles from the outer membrane. Although mechanisms behind the membrane vesicle biogenesis are more and more understood, a general notion on the position of membrane vesicles in stress responses still needs to be unraveled. Yet, it is becoming clear that there are several aspects in the mechanisms for biogenesis which are common. An elegant example hereof that was addressed in this review, is a recent study by Roier et al. that reveals a mechanism for the membrane vesicle biogenesis that is highly conserved among Gram-negative bacteria. This mechanism depends on the VacJ/YrbC ABC transporter and was found to be sensitive to iron depletion (Roier et al. Citation2016). Another prominent example of a highly-conserved mechanism for the release of membrane vesicles is the modification of LPS and the release of membrane vesicles in response to environmental factors that bind LPS.
From a physiological perspective, membrane vesicles can aid in the acquisition of nutrients but also serve to protect from antimicrobial peptides, antibiotics, and innate and adaptive immune attacks. Protection from antimicrobial peptides is provided in two ways. Directly, when these peptides bind the bacterial outer membrane, the formation of membrane vesicles enables bacteria to ‘shake off’ threatening antimicrobial peptides to prevent further damage. Released membrane vesicles can act as decoys and provide further protection against these peptides. Finally, the structure of LPS is not static, but subject to constant remodeling and bacteria can modulate their LPS in response to environmental changes. Bacteria that modulate their LPS towards a less pro-inflammatory form also have been shown to release membrane vesicles with diminished pro-inflammatory characteristics. Moreover, it has been shown that membrane vesicles can provide as decoys to escape innate and adaptive immune responses. Hereby membrane vesicles may also help bacteria to shape their environment.
There is substantial evidence that indicates there is a high similarity between the different stress response pathways that are induced by environmental stressors like nutrient stress, oxidative stress, and interaction with the host (van de Waterbeemd et al. Citation2013; Kulp et al. Citation2015). An improved insight on how these stress responses affect the bacterial vesiculation phenotype as well as how they affect the composition and immunogenicity of the vesicles will be key in understanding how commensals and pathogens can conquer host defense mechanism during infection/colonization. This is particularly important as the release of membrane vesicles is also associated with virulence, tolerance, the acquisition of nutrients, and antibiotic resistance. This knowledge is of paramount therapeutic value, not only can it benefit the development of detoxified membrane vesicles for vaccination purposes, it may also help to prevent phenotypic alterations that result in increased (membrane-vesicle mediated) bacterial resilience to treatment regimens.
Disclosure statement
No potential conflict of interest was reported by the authors.
References
- Aebi C, Stone B, Beucher M, Cope LD, Maciver I, Thomas SE, McCracken GH Jr, Sparling PF, Hansen EJ. 1996. Expression of the CopB outer membrane protein by Moraxella catarrhalis is regulated by iron and affects iron acquisition from transferrin and lactoferrin. Infect Immun. 64:2024–2030.
- Alba BM, Leeds JA, Onufryk C, Lu CZ, Gross CA. 2002. DegS and YaeL participate sequentially in the cleavage of RseA to activate the σE-dependent extracytoplasmic stress response. Genes Dev. 16:2156–2168.
- Alexander C, Rietschel ET. 2001. Bacterial lipopolysaccharides and innate immunity. J Endotoxin Res. 7:167–202.
- Bader MW, Sanowar S, Daley ME, Schneider AR, Cho U, Xu W, Klevit RE, Le Moual H, Miller SI. 2005. Recognition of antimicrobial peptides by a bacterial sensor kinase. Cell. 122:461–472.
- Baker JL, Chen L, Rosenthal JA, Putnam D, DeLisa MP. 2014. Microbial biosynthesis of designer outer membrane vesicles. Curr Opin Biotechnol. 29:76–84.
- Balsalobre C, Silván JM, Berglund S, Mizunoe Y, Uhlin BE, Wai SN. 2006. Release of the type I secreted alpha-haemolysin via outer membrane vesicles from Escherichia coli. Mol Microbiol. 59:99–112.
- Bearson BL, Bearson SMD. 2008. The role of the QseC quorum-sensing sensor kinase in colonization and norepinephrine-enhanced motility of Salmonella enterica serovar Typhimurium. Microb Pathog. 44:271–278.
- Bernadac A, Gavioli M, Lazzaroni JC, Raina S, Lloubès R. 1998. Escherichia coli tol-pal mutants form outer membrane vesicles. J Bacteriol. 180:4872–4878.
- Beutler B, Rietschel ET. 2003. Timeline: innate immune sensing and its roots: the story of endotoxin. Nat Rev Immunol. 3:169–176.
- Boyanova L. 2017. Stress hormone epinephrine (adrenaline) and norepinephrine (noradrenaline) effects on the anaerobic bacteria. Anaerobe. 44:13–19.
- Brogden KA. 2005. Antimicrobial peptides: pore formers or metabolic inhibitors in bacteria? Nat Rev Microbiol. 3:238–250.
- Brozek KA, Raetz CR. 1990. Biosynthesis of lipid A in Escherichia coli. Acyl carrier protein-dependent incorporation of laurate and myristate. J Biol Chem. 265:15410–15417.
- Burman JD, Leung E, Atkins KL, O’Seaghdha MN, Lango L, Bernadó P, Bagby S, Svergun DI, Foster TJ, Isenman DE, et al. 2008. Interaction of human complement with Sbi, a staphylococcal immunoglobulin-binding protein: indications of a novel mechanism of complement evasion by Staphylococcus aureus. J Biol Chem. 283:17579–17593.
- Cabiscol E, Tamarit J, Ros J. 2000. Oxidative stress in bacteria and protein damage by reactive oxygen species. Int Microbiol. 3:3–8.
- Chen L, Valentine JL, Huang C-J, Endicott CE, Moeller TD, Rasmussen JA, Fletcher JR, Boll JM, Rosenthal JA, Dobruchowska J, et al. 2016. Outer membrane vesicles displaying engineered glycotopes elicit protective antibodies. Proc Natl Acad Sci USA. 113:E3609–E3618.
- Ciofu O, Beveridge TJ, Kadurugamuwa J, Walther-Rasmussen J, Høiby N. 2000. Chromosomal beta-lactamase is packaged into membrane vesicles and secreted from Pseudomonas aeruginosa. J Antimicrob Chemother. 45:9–13.
- Cobey S. 2014. Pathogen evolution and the immunological niche. Ann NY Acad Sci. 1320:1–15.
- Cullen TW, Schofield WB, Barry NA, Putnam EE, Rundell EA, Trent MS, Degnan PH, Booth CJ, Yu H, Goodman AL. 2015. Gut microbiota. Antimicrobial peptide resistance mediates resilience of prominent gut commensals during inflammation. Science. 347:170–175.
- Dalebroux ZD, Matamouros S, Whittington D, Bishop RE, Miller SI. 2014. PhoPQ regulates acidic glycerophospholipid content of the Salmonella Typhimurium outer membrane. Proc Natl Acad Sci USA. 111:1963–1968.
- Davis KM, Weiser JN. 2011. Modifications to the peptidoglycan backbone help bacteria to establish infection. Infect Immun. 79:562–570.
- Deatherage BL, Lara JC, Bergsbaken T, Barrett SLR, Lara S, Cookson BT. 2009. Biogenesis of bacterial membrane vesicles. Mol Microbiol. 72:1395–1407.
- Dutta S, Iida K, Takade A, Meno Y, Nair GB, Yoshida S. 2004. Release of Shiga toxin by membrane vesicles in Shigella dysenteriae serotype 1 strains and in vitro effects of antimicrobials on toxin production and release. Microbiol Immunol. 48:965–969.
- Elhenawy W, Bording-Jorgensen M, Valguarnera E, Haurat MF, Wine E, Feldman MF. 2016. LPS remodeling triggers formation of outer membrane vesicles in Salmonella. Mbio. 7:e00940-16.
- Elhenawy W, Debelyy MO, Feldman MF. 2014. Preferential packing of acidic glycosidases and proteases into bacteroides outer membrane vesicles. Mbio. 5:e00909–e00914.
- Fernández L, Gooderham WJ, Bains M, McPhee JB, Wiegand I, Hancock REW. 2010. Adaptive resistance to the ‘last hope’ antibiotics polymyxin B and colistin in Pseudomonas aeruginosa is mediated by the novel two-component regulatory system ParR-ParS. Antimicrob Agents Chemother. 54:3372–3382.
- Flierl MA, Rittirsch D, Nadeau BA, Sarma JV, Day DE, Lentsch AB, Huber-Lang MS, Ward PA. 2009. Upregulation of phagocyte-derived catecholamines augments the acute inflammatory response. PLoS One. 4:e4414.
- Freestone P. 2013. Communication between bacteria and their hosts. Scientifica. 2013:361073.
- Gaspar JA, Thomas JA, Marolda CL, Valvano MA. 2000. Surface expression of O-specific lipopolysaccharide in Escherichia coli requires the function of the TolA protein. Mol Microbiol. 38:262–275.
- Gellatly SL, Needham B, Madera L, Trent MS, Hancock REW. 2012. The Pseudomonas aeruginosa PhoP-PhoQ two-component regulatory system is induced upon interaction with epithelial cells and controls cytotoxicity and inflammation. Infect Immun. 80:3122–3131.
- Gray MD, Lampel KA, Strockbine NA, Fernandez RE, Melton-Celsa AR, Maurelli AT. 2014. Clinical isolates of Shiga toxin 1a-producing Shigella flexneri with an epidemiological link to recent travel to Hispañiola. Emerg Infect Dis. 20:1669–1677.
- Grenier D, Bélanger M. 1991. Protective effect of Porphyromonas gingivalis outer membrane vesicles against bactericidal activity of human serum. Infect Immun. 59:3004–3008.
- Grenier D, Bertrand J, Mayrand D. 1995. Porphyromonas gingivalis outer membrane vesicles promote bacterial resistance to chlorhexidine. Oral Microbiol Immunol. 10:319–320.
- Groisman EA. 2001. The pleiotropic two-component regulatory system PhoP-PhoQ. J Bacteriol. 183:1835–1842.
- Guo L, Lim KB, Gunn JS, Bainbridge B, Darveau RP, Hackett M, Miller SI. 1997. Regulation of lipid A modifications by Salmonella typhimurium virulence genes phoP-phoQ. Science. 276:250–253.
- Guo L, Lim KB, Poduje CM, Daniel M, Gunn JS, Hackett M, Miller SI. 1998. Lipid A acylation and bacterial resistance against vertebrate antimicrobial peptides. Cell. 95:189–198.
- Haurat MF, Elhenawy W, Feldman MF. 2015. Prokaryotic membrane vesicles: new insights on biogenesis and biological roles. Biol Chem. 396:95–109.
- Henderson JC, Zimmerman SM, Crofts AA, Boll JM, Kuhns LG, Herrera CM, Trent MS. 2016. The power of asymmetry: architecture and assembly of the gram-negative outer membrane lipid bilayer. Annu Rev Microbiol. 70:255–278.
- Hibbing ME, Fuqua C, Parsek MR, Peterson SB. 2010. Bacterial competition: surviving and thriving in the microbial jungle. Nat Rev Microbiol. 8:15–25.
- Ho D, Kim S, Kang W, Seok Y, Lee S, Lee S, You S, Lee HK, Chang KT, Shin EC. 2011. Adjuvant effect of bacterial outer membrane vesicles with penta-acylated lipopolysaccharide on antigen-specific T cell priming. Vaccine. 29:8293–8301.
- Hughes DT, Sperandio V. 2008. Inter-kingdom signalling: communication between bacteria and their hosts. Nat Rev Microbiol. 6:111–120.
- Kadurugamuwa JL, Beveridge TJ. 1997. Natural release of virulence factors in membrane vesicles by Pseudomonas aeruginosa and the effect of aminoglycoside antibiotics on their release. J Antimicrob Chemother. 40:615–621.
- Kang CS, Ban M, Choi EJ, Moon HG, Jeon JS, Kim DK, Park SK, Jeon SG, Roh TY, Myung SJ, et al. 2013. Extracellular vesicles derived from gut microbiota, especially Akkermansia muciniphila, protect the progression of dextran sulfate sodium-induced colitis. PLoS One. 8(10):e76520. doi: 10.1371/journal.pone.0076520.
- Karavolos MH, Bulmer DM, Spencer H, Rampioni G, Schmalen I, Baker S, Pickard D, Gray J, Fookes M, Winzer K, et al. 2011. Salmonella Typhi sense host neuroendocrine stress hormones and release the toxin haemolysin E. EMBO Rep. 12:252–258.
- Kawano M, Manabe T, Kawasaki K. 2010. Salmonella enterica serovar Typhimurium lipopolysaccharide deacylation enhances its intracellular growth within macrophages. FEBS Lett. 584:207–212.
- Kawasaki K, Ernst RK, Miller SI. 2004. 3-O-deacylation of lipid A by PagL, a PhoP/PhoQ-regulated deacylase of Salmonella typhimurium, modulates signaling through Toll-like receptor 4. J Biol Chem. 279:20044–20048.
- Kim DY. 2015. Two stress sensor proteins for the expression of sigmaE regulon: DegS and RseB. J Microbiol. 53:306–310.
- Kitagawa R, Takaya A, Ohya M, Mizunoe Y, Takade A, Yoshida S, Isogai E, Yamamoto T. 2010. Biogenesis of Salmonella enterica serovar typhimurium membrane vesicles provoked by induction of PagC. J Bacteriol. 192:5645–5656.
- Kogoma T, Torrey TA, Connaughton MJ. 1979. Induction of UV-resistant DNA replication in escherichia coli: induced stable DNA replication as an SOS function. Mol Gen Genet. 176:1–9.
- Kohanski MA, Dwyer DJ, Collins JJ. 2010. How antibiotics kill bacteria: from targets to networks. Nat Rev Microbiol. 8:423–435.
- Kropinski AM, Lewis V, Berry D. 1987. Effect of growth temperature on the lipids, outer membrane proteins, and lipopolysaccharides of Pseudomonas aeruginosa PAO. J Bacteriol. 169:1960–1966.
- Kuehn MJ, Kesty NC. 2005. Bacterial outer membrane vesicles and the host-pathogen interaction. Genes Dev. 19:2645–2655.
- Kulp A, Kuehn MJ, Annu Rev M. 2012. Biological functions and biogenesis of secreted bacterial outer membrane vesicles. Ann Rev Microbiol. 64:163–164.
- Kulp AJ, Sun B, Ai T, Manning AJ, Orench-rivera N. 2015. Genome-wide assessment of outer membrane vesicle production in Escherichia coli. PLoS One. 10:e0139200.
- Kwon DH, Lu CD. 2006. Polyamines induce resistance to cationic peptide, aminoglycoside, and quinolone antibiotics in Pseudomonas aeruginosa PAO1. Antimicrob Agents Chemother. 50:1615–1622.
- Lam JS, Taylor VL, Islam ST, Hao Y, Kocíncová D. 2011. Genetic and functional diversity of Pseudomonas aeruginosa lipopolysaccharide. Front Microbiol. 2:118.
- Lappann M, Otto A, Becher D, Vogel U. 2013. Comparative proteome analysis of spontaneous outer membrane vesicles and purified outer membranes of Neisseria meningitidis. J Bacteriol. 195:4425–4435.
- Lebeer S, Vanderleyden J, Keersmaecker SCJD. 2010. Host interactions of probiotic bacterial surface molecules: comparison with commensals and pathogens. Nat Rev Microbiol. 8:171–184.
- Lecomte S, Baron MH, Chenon MT, Coupry C, Moreau NJ. 1994. Effect of magnesium complexation by fluoroquinolones on their antibacterial properties. Antimicrob Agents Chemother. 38:2810–2816.
- Lee E-Y, Choi D-Y, Kim D-K, Kim J-W, Park JO, Kim S, Kim SH, Desiderio DM, Kim YK, Kim KP, Gho YS. 2009. Gram-positive bacteria produce membrane vesicles: proteomics-based characterization of Staphylococcus aureus-derived membrane vesicles. Proteomics. 9:5425–5436.
- Lee JC, Lee EJ, Lee JH, Jun SH, Choi CW, Kim SI, Kang SS, Hyun S. 2012. Klebsiella pneumoniae secretes outer membrane vesicles that induce the innate immune response. FEMS Microbiol Lett. 331:17–24.
- Lee T-Y, Kim C-U, Bae E-H, Seo S-H, Jeong DG, Yoon S-W, Chang KT, Kim YS, Kim SH, Kim DJ. 2016. Outer membrane vesicles harboring modified lipid A moiety augment the efficacy of an influenza vaccine exhibiting reduced endotoxicity in a mouse model. Vaccine. 35:586–595.
- Loeb MR, Kilner J. 1978. Release of a special fraction of the outer membrane from both growing and phage T4-infected Escherichia coli B. Biochim Biophys Acta. 514:117–127.
- Lyte M, Arulanandam BP, Frank CD. 1996. Production of Shiga-like toxins by Escherichia coli O157: H7 can be influenced by the neuroendocrine hormone norepinephrine. J Lab Clin Med. 128:392–398.
- Lyte M, Erickson AK, Arulanandam BP, Frank CD, Crawford MA, Francis DH. 1997. Norepinephrine-induced expression of the K99 pilus adhesin of enterotoxigenic Escherichia coli. Biochem Biophys Res Commun. 232:682–686.
- MacDonald IA. 2013. Characterizing stress-induced outer membrane vesicle production in Pseudomonas aeruginosa [dissertation]. Durham (NC): Department of Molecular Genetics and Microbiology, Duke University.
- MacDonald IA, Kuehn MJ. 2012. Offense and defense: microbial membrane vesicles play both ways. Res Microbiol. 163:607–618.
- MacDonald IA, Kuehn MJ. 2013. Stress-induced outer membrane vesicle production by Pseudomonas aeruginosa. J Bacteriol. 195(13):2971–2981.
- Macfarlene ELA, Kwasnicka A, Hancock REW. 2000. Role of Pseudomonas aeruginosa Phop-PhoQ in resistance to antimicrobial cationic peptides and aminoglycosides. Microbiology. 146:2543–2554.
- Maeshima N, Fernandez RC. 2013. Recognition of lipid A variants by the TLR4-MD-2 receptor complex. Front Cell Infect Microbiol. 3:3.
- Makin SA, Beveridge TJ. 1996. Pseudomonas aeruginosa PAO1 ceases to express serotype-specific lipopolysaccharide at 45 degrees C. J Bacteriol. 178:3350–3352.
- Malinverni JC, Silhavy TJ. 2009. An ABC transport system that maintains lipid asymmetry in the Gram-negative outer membrane. Proc Natl Acad Sci USA. 106:8009–8014.
- Manning AJ, Kuehn MJMJ. 2011. Contribution of bacterial outer membrane vesicles to innate bacterial defense. BMC Microbiol. 11:258.
- Maredia R, Devineni N, Lentz P, Dallo SF, Yu J, Guentzel N, Chambers J, Arulanandam B, Haskins WE, Weitao T. 2012. Vesiculation from Pseudomonas aeruginosa under SOS. Sci World J. 2012:402919.
- Marshall AJ, Piddock LJ. 1994. Interaction of divalent cations, quinolones and bacteria. J Antimicrob Chemother. 34:465–483.
- Marsland BJ, Gollwitzer ES. 2014. Host-microorganism interactions in lung diseases. Nat Rev Immunol. 14:827–835.
- Martin NL, Beveridge TJ. 1986. Gentamicin interaction with Pseudomonas aeruginosa cell envelope. Antimicrob Agents Chemother. 29:1079–1087.
- Martorana AAM, Motta S, Di Silvestre D, Falchi F, Dehò G, Mauri P, Sperandeo P, Polissi A. 2014. Dissecting Escherichia coli outer membrane biogenesis using differential proteomics. PLoS One. 9:e100941. doi: 10.1371/journal.pone.0100941.
- Mashburn LM, Whiteley M. 2005. Membrane vesicles traffic signals and facilitate group activities in a prokaryote. Nature. 437:422–425.
- Mashburn-Warren L, Howe J, Garidel P, Richter W, Steiniger F, Roessle M, Brandenburg K, Whiteley M. 2008. Interaction of quorum signals with outer membrane lipids: insights into prokaryotic membrane vesicle formation. Mol Microbiol. 69:491–502.
- Mashburn-Warren LM, Whiteley M. 2006. Special delivery: vesicle trafficking in prokaryotes. Mol Microbiol. 61:839–846.
- Matsuura M. 2013. Structural modifications of bacterial lipopolysaccharide that facilitate Gram-negative bacteria evasion of host innate immunity. Front Immunol. 4:1–9.
- McBroom AJ, Johnson AP, Vemulapalli S, Kuehn MJ. 2006. Outer membrane vesicle production by Escherichia coli is independent of membrane instability. J Bacteriol. 188:5385–5392.
- McBroom AJ, Kuehn MJ. 2007. Release of outer membrane vesicles by Gram-negative bacteria is a novel envelope stress response. Mol Microbiol. 63:545–558.
- McCaig WD, Koller A, Thanassi DG. 2013. Production of outer membrane vesicles and outer membrane tubes by Francisella novicida. J Bacteriol. 195:1120–1132.
- McGroarty EJ, Rivera M. 1990. Growth-dependent alterations in production of serotype-specific and common antigen lipopolysaccharides in Pseudomonas aeruginosa PAO1. Infect Immun. 58:1030–1037.
- Metruccio MME, Evans DJ, Gabriel MM, Kadurugamuwa JL, Fleiszig SMJ. 2016. Pseudomonas aeruginosa outer membrane vesicles triggered by human mucosal fluid and lysozyme can prime host tissue surfaces for bacterial adhesion. Front Microbiol. 7:871.
- Mogensen JE, Otzen DE. 2005. Interactions between folding factors and bacterial outer membrane proteins. Mol Microbiol. 57:326–346.
- Montminy SW, Khan N, McGrath S, Walkowicz MJ, Sharp F, Conlon JE, Fukase K, Kusumoto S, Sweet C, Miyake K, et al. 2006. Virulence factors of Yersinia pestis are overcome by a strong lipopolysaccharide response. Nat Immunol. 7:1066–1073.
- Moon DC, Choi CH, Lee JH, Choi C-W, Kim H-Y, Park JS, Kim SI, Lee JC. 2012. Acinetobacter baumannii outer membrane protein A modulates the biogenesis of outer membrane vesicles. J Microbiol. 50:155–160.
- Moore RA, Hancock REW. 1986. Involvement of outer membrane of Pseudomonas cepacia in aminoglycoside and polymyxin resistance. Antimicrob Agents Chemother. 30:923–926.
- Moreno G, Rumbo M. 2014. Outer membrane vesicles obtained from Bordetella pertussis Tohama expressing the lipid A deacylase PagL as a novel acellular vaccine candidate. Vaccine. 29:1649–1656.
- Munford RS, Varley AW. 2006. Shield as signal: lipopolysaccharides and the evolution of immunity to Gram-negative bacteria. PLoS Pathog. 2:e67.
- Nakano M, Takahashi A, Sakai Y, Nakaya Y. 2007. Modulation of pathogenicity with norepinephrine related to the type III secretion system of Vibrio parahaemolyticus. J Infect Dis. 195:1353–1360.
- Nathan C, Cunningham-Bussel A. 2013. Beyond oxidative stress: an immunologist’s guide to reactive oxygen species. Nat Rev Immunol. 13:349–361.
- Needham BD, Carroll SM, Giles DK, Georgiou G, Whiteley M, Trent MS. 2013. Modulating the innate immune response by combinatorial engineering of endotoxin. Proc Natl Acad Sci USA. 110:1464–1469.
- Needham BD, Trent MS. 2013. Fortifying the barrier: the impact of lipid A remodelling on bacterial pathogenesis. Nat Rev Microbiol. 11:467–481.
- Netea MG, van Deuren M, Kullberg BJ, Cavaillon J-M, Van der Meer JWM. 2002. Does the shape of lipid A determine the interaction of LPS with Toll-like receptors? Trends Immunol. 23:135–139.
- Nordström T, Blom AM, Tan TT, Forsgren A, Riesbeck K. 2005. Ionic binding of C3 to the human pathogen Moraxella catarrhalis is a unique mechanism for combating innate immunity. J Immunol. 175:3628–3636.
- Park BS, Song DH, Kim HM, Choi B-S, Lee H, Lee J-O. 2009. The structural basis of lipopolysaccharide recognition by the TLR4-MD-2 complex. Nature. 458:1191–1195.
- Perez Vidakovics MLA, Jendholm J, Mörgelin M, Månsson A, Larsson C, Cardell L-O, Riesbeck K. 2010. B cell activation by outer membrane vesicles: a novel virulence mechanism. PLoS Pathog. 6:e1000724.
- Pettit RK, Judd RC. 1992. The interaction of naturally elaborated blebs from serum-susceptible and serum-resistant strains of Neisseria gonorrhoeae with normal human serum. Mol Microbiol. 6:729–734.
- Pietras Z, Jagusztyn-krynicka K. 2009. Peptidoglycan-associated lipoprotein (Pal) of Gram-negative bacteria: function, structure, role in pathogenesis and potential application in immunoprophylaxis. FEMS Microbiol Lett. 298:1–11.
- Poole K. 2012. Bacterial stress responses as determinants of antimicrobial resistance. J Antimicrob Chemother. 67:2069–2089.
- Prados-Rosales R, Baena A, Martinez LR, Luque-Garcia J, Kalscheuer R, Veeraraghavan U, Camara C, Nosanchuk JD, Besra GS, Chen B, et al. 2011. Mycobacteria release active membrane vesicles that modulate immune responses in a TLR2-dependent manner in mice. J Clin Invest. 121:1471–1483.
- Raetz CRH, Reynolds CM, Trent MS, Bishop RE. 2007. Lipid A modification systems in gram-negative bacteria. Annu Rev Biochem. 76:295–329.
- Ribet D, Cossart P. 2015. How bacterial pathogens colonize their hosts and invade deeper tissues. Microbes Infect. 17:173–183.
- Rohmer L, Hocquet D, Miller SI. 2011. Are pathogenic bacteria just looking for food? Metabolism and microbial pathogenesis. Trends Microbiol. 19:341–348.
- Roier S, Zingl FG, Cakar F, Durakovic S, Kohl P, Eichmann TO, Klug L, Gadermaier B, Weinzerl K, Prassl R, et al. 2016. A novel mechanism for the biogenesis of outer membrane vesicles in Gram-negative bacteria. Nat Commun. 7:10515.
- Rosadini CV, Ram S, Akerley BJ. 2014. Outer membrane protein P5 is required for resistance of nontypeable Haemophilus influenzae to both the classical and alternative complement pathways. Infect Immun. 82:640–649.
- Rowley G, Spector M, Kormanec J, Roberts M. 2006. Pushing the envelope: extracytoplasmic stress responses in bacterial pathogens. Nat Rev Microbiol. 4:383–394.
- Ruiz N, Kahne D, Silhavy TJ. 2006. Advances in understanding bacterial outer-membrane biogenesis. Nat Rev Microbiol. 4:57–66.
- Sabra W, Kim EJ, Zeng AP. 2002. Physiological responses of Pseudomonas aeruginosa PAO1 to oxidative stress in controlled microaerobic and aerobic cultures. Microbiology. 148:3195–3202.
- Sabra W, Lunsdorf H, Zeng AP. 2003. Alterations in the formation of lipopolysaccharide and membrane vesicles on the surface of Pseudomonas aeruginosa PAO1 under oxygen stress conditions. Microbiology. 149:2789–2795.
- Schaar V, De Vries SPW, Perez Vidakovics MLA, Bootsma HJ, Larsson L, Hermans PWM, Bjartell A, Mörgelin M, Riesbeck K. 2011. Multicomponent Moraxella catarrhalis outer membrane vesicles induce an inflammatory response and are internalized by human epithelial cells. Cell Microbiol. 13:432–449.
- Schaar V, Nordström T, Mörgelin M, Riesbeck K. 2011. Moraxella catarrhalis outer membrane vesicles carry β-lactamase and promote survival of Streptococcus pneumoniae and Haemophilus influenzae by inactivating amoxicillin. Antimicrob Agents Chemother. 55:3845–3853.
- Schaible UE, Kaufmann SH. 2004. Iron and microbial infection. Nat Rev Microbiol. 2:946–953.
- Schertzer JW, Whiteley M. 2012. A bilayer-couple model of bacterial outer membrane vesicle. Mbio. 3:1–7.
- Schooling SR, Beveridge TJ. 2006. Membrane vesicles: an overlooked component of the matrices of biofilms. J Bacteriol. 188:5945–5957.
- Schromm AB, Brandenburg K, Loppnow H, Moran AP, Koch MHJ, Rietschel ET, Seydel U. 2000. Biological activities of lipopolysaccharides are determined by the shape of their lipid A portion. Eur J Biochem. 267:2008–2013.
- Schwechheimer C, Kuehn MJ. 2015. Outer-membrane vesicles from Gram-negative bacteria: biogenesis and functions. Nat Rev Microbiol. 13:605–619.
- Seo SW, Kim D, Szubin R, Palsson BO. 2015. Genome-wide reconstruction of OxyR and SoxRS transcriptional regulatory networks under oxidative stress in Escherichia coli K-12 MG1655. Cell Rep. 12:1289–1299.
- Sharpe SW, Kuehn MJ, Mason KM. 2011. Elicitation of epithelial cell-derived immune effectors by outer membrane vesicles of nontypeable haemophilus influenzae. Infect Immun. 79:4361–4369.
- Shi Y, Cromie MJ, Hsu F-F, Turk J, Groisman EA. 2004. PhoP-regulated Salmonella resistance to the antimicrobial peptides magainin 2 and polymyxin B. Mol Microbiol. 53:229–241.
- Silhavy TJ, Ruiz N, Kahne D. 2006. Advances in understanding bacterial outer-membrane biogenesis. Nat Rev Microbiol. 4:57–66.
- Sohn J, Grant R. a, Sauer RT. 2009. OMP peptides activate the DegS stress-sensor protease by a relief of inhibition mechanism. Structure. 17:1411–1421.
- Song T, Mika F, Lindmark B, Liu Z, Schild S, Bishop A, Zhu J, Camilli A, Johansson J, Vogel J, Wai SN. 2008. A new Vibrio cholerae sRNA modulates colonization and affects release of outer membrane vesicles. Mol Microbiol. 70:100–111.
- Sonntag I, Schwarz H, Hirota Y, Henning U. 1978. Cell envelope and shape of Escherichia coli: multiple mutants missing the outer membrane lipoprotein and other major outer membrane proteins. J Bacteriol. 136:280–285.
- Steimle A, Autenrieth IB, Frick JS. 2016. Structure and function: lipid A modifications in commensals and pathogens. Int J Med Microbiol. 306:290–301.
- Tan TT, Mörgelin M, Forsgren A, Riesbeck K. 2007. Haemophilus influenzae Survival during complement-mediated attacks is promoted by Moraxella catarrhalis outer membrane vesicles. J Infect Dis. 195:1661–1670.
- Tashiro Y, Ichikawa S, Nakajima-Kambe T, Uchiyama H, Nomura N. 2010. Pseudomonas quinolone signal affects membrane vesicle production in not only gram-negative but also gram-positive bacteria. Microbes Environ. 25:120–125.
- Tashiro Y, Sakai R, Toyofuku M, Sawada I, Nakajima-Kambe T, Uchiyama H, Nomura N. 2009. Outer membrane machinery and alginate synthesis regulators control membrane vesicle production in Pseudomonas aeruginosa. J Bacteriol. 191:7509–7519.
- Trent MS. 2004. Biosynthesis, transport, and modification of lipid A. Biochem Cell Biol. 82:71–86.
- Trent MS, Pabich W, Raetz CR, Miller SI. 2001. A PhoP/PhoQ-induced Lipase (PagL) that catalyzes 3-O-deacylation of lipid A precursors in membranes of Salmonella typhimurium. J Biol Chem. 276:9083–9092.
- van de Waterbeemd B, Zomer G, van den IJssel J, van Keulen L, Eppink MH, van der Ley P, van der Pol LA. 2013. Cysteine depletion causes oxidative stress and triggers outer membrane vesicle release by neisseria meningitidis; implications for vaccine development. PLoS One. 8:e543314.
- van de Waterbeemd B, Streefland M, Van Der LP, Zomer B, Van DH, Martens D, Wijffels R, van der Pol L. 2010. Improved OMV vaccine against Neisseria meningitidis using genetically engineered strains and a detergent-free purification process. Vaccine. 28:4810–4816.
- Vatanen T, Kostic AD, d’Hennezel E, Siljander H, Franzosa EA, Yassour M, Kolde R, Vlamakis H, Arthur TD, Hämäläinen AM, et al. 2016. Variation in microbiome LPS immunogenicity contributes to autoimmunity in humans. Cell. 165:842–853.
- Veith PD, Chen YY, Gorasia DG, Chen D, Glew MD, O’Brien-Simpson NM, Cecil JD, Holden JA, Reynolds EC. 2014. Porphyromonas gingivalis outer membrane vesicles exclusively contain outer membrane and periplasmic proteins and carry a cargo enriched with virulence factors. J Proteome Res. 13:2420–2432.
- Vines ED, Marolda CL, Balachandran A, Valvano MA. 2005. Defective O-antigen polymerization in tolA and pal mutants of Escherichia coli in response to extracytoplasmic stress. J Bacteriol. 187:3359–3368.
- Vlisidou I, Lyte M, van Diemen PM, Hawes P, Monaghan P, Wallis TS, Stevens MP. 2004. The Neuroendocrine stress hormone norepinephrine augments Escherichia coli O157: H7-induced enteritis and adherence in a bovine ligated ileal loop model of infection. Infect Immun. 72:5446–5451.
- Walker SG, Beveridge TJ. 1988. Amikacin disrupts the cell envelope of Pseudomonas aeruginosa ATCC 9027. Can J Microbiol. 34:12–18.
- Wessel AK, Liew J, Kwon T, Marcotte EM, Whiteley M. 2013. Role of Pseudomonas aeruginosa peptidoglycan-associated outer membrane proteins in vesicle formation. J Bacteriol. 195:213–219.
- Zimmerberg J, Kozlov MM. 2006. How proteins produce cellular membrane curvature. Nat Rev Mol Cell Biol. 7:9–19.