Abstract
Considering increasing number of pathogens resistant towards commonly used antibiotics as well as antiseptics, there is a pressing need for antimicrobial approaches that are capable of inactivating pathogens efficiently without the risk of inducing resistances. In this regard, an alternative approach is the antimicrobial photodynamic therapy (aPDT). The antimicrobial effect of aPDT is based on the principle that visible light activates a per se non-toxic molecule, the so-called photosensitizer (PS), resulting in generation of reactive oxygen species that kill bacteria unselectively via an oxidative burst. During the last 10–20 years, there has been extensive in vitro research on novel PS as well as light sources, which is now to be translated into clinics. In this review, we aim to provide an overview about the history of aPDT, its fundamental photochemical and photophysical mechanisms as well as photosensitizers and light sources that are currently applied for aPDT in vitro. Furthermore, the potential of resistances towards aPDT is extensively discussed and implications for proper comparison of in vitro studies regarding aPDT as well as for potential application fields in clinical practice are given. Overall, this review shall provide an outlook on future research directions needed for successful translation of promising in vitro results in aPDT towards clinical practice.
Biofilm infections and antimicrobial resistance as a major clinical challenge
Bacteria persist in structured biofilm ecosystems and rarely in cultures of single species that are traditionally used by microbiologists to study the behavior of microorganisms in vitro (Davey and O’Toole Citation2000). In biofilms, microorganisms are remarkably less susceptible towards antimicrobials as compared to their planktonic counterparts (Mah and O’Toole Citation2001; Stewart and Costerton Citation2001). The mechanisms of tolerance and resistance in biofilms include slow penetration of antimicrobials through the biofilm matrix, altered microenvironment within the biofilm, different stress response of bacterial cells, and the formation of a sub-population of so-called persister cells (Chambless et al. Citation2006). Considering that biofilm infections are caused by a diversity of microbial species, potential resistances within the biofilm can easily be transferred among different species by horizontal gene transfer (Lewis Citation2001; Burmølle et al. Citation2006; Madsen et al. Citation2012; Wolcott et al. Citation2013).
It has been estimated that more than 60% of all microbial infections are caused by biofilms, also with reference to hospital-acquired infections, where sterile conditions are often compromised (Lewis Citation2001; Busscher et al. Citation2012). Frequent biofilm infections include dental infections (caused by dental plaque), dermal infections, urinary tract infections, middle-ear infections, endocarditis and implant- or catheter-associated infections, whereby at least the latter potentially can lead to serious, i.e. lethal, outcomes for patients (Lewis Citation2001; Zimmerli et al. Citation2004; Choy et al. Citation2008; Marsh et al. Citation2011).
Nowadays, several pathogens (especially Pseudomonas aeruginosa, Staphylococcus aureus, Escherichia coli, Klebsiella pneumoniae, Enterococcus faecalis, and Acinetobacter baumannii) have been reported to be increasingly resistant to a wide range of antibiotics (Laxminarayan et al. Citation2016; Renwick et al. Citation2016). Some of them, e.g. methicillin-resistant S. aureus (MRSA) strains and the multi-resistant extended spectrum beta-lactamase (ESBL)-producing bacteria have become established pathogens in hospitals (McCarthy et al. Citation2010; Holmes et al. Citation2016; Laxminarayan et al. Citation2016).
After the discovery of penicillin in 1928, the golden era of antibiotics began in the 1940s and lasted until the end of 1960s with the development of different classes of antibiotics including aminoglycosides, tetracyclines, chloramphenicols, macrolides, glycopeptides, oxazolidinones, ansamycins, quinolones, and streptogramins (Aminov Citation2010; Renwick et al. Citation2016). However, as mentioned above, pathogens became resistant against most of these antibiotics and from the 1970s until the present, a lack of new antibiotics threatens to result in a stand-off with human pathogens and a return to the pre-antibiotic era (Aminov Citation2010; Lewis Citation2012; Laxminarayan et al. Citation2016; Renwick et al. Citation2016). This also relates to antifungal drug resistance, which can occur with all drug classes, and involves acquired resistance in susceptible strains and selection of inherently less susceptible species (Perlin et al. Citation2017). Candida and Aspergillus species such as Candida glabrata, Candida auris, and Aspergillus fumigatus have been reported to increasingly acquire resistance against major anitifungal drugs, such as azoles and echinocandins (Perlin et al. Citation2017). It should also be kept in mind that resistance against disinfectants like chlorhexidine digluconate may correlate with antibiotic resistance as previously reported for Staphylococci (Meyer and Cookson Citation2010; Horner et al. Citation2012; Kampf Citation2016). Such a correlation between disinfectant resistance and antibiotic resistance makes the widespread use of disinfectants questionable (Kampf Citation2016).
A recent review on antimicrobial resistance, which was sponsored by the UK Department of Health and commissioned in 2014 by the UK Prime Minister reported that the number of deaths attributable to global antibiotic resistance will increase to 10 million per year until 2050 compared to the current 700 000 annual deaths (Renwick et al. Citation2016). Furthermore, this report stressed the reluctance of pharmaceutical companies to invest into the development of new antibiotics.
Therefore, there is an urgent need for alternative treatment methods against resistant microorganisms. These methods should also be designed to prevent the emergence of antibiotic resistance after use, e.g. acting according to a multi-target mode of action instead of the key-lock principle known from conventional antibiotics.
Consequently, in a recent review in Lancet Infectious Diseases, several alternatives for antibiotics were summarized and discussed from a medical and economical point of view (Czaplewski et al. Citation2016). The alternatives mentioned there included antibodies, probiotics, phage lysins, different classes of bacteriophages, immune stimulation (e.g. phenyl butyrate and vitamin D), vaccines, antimicrobial, anti-biofilm, and innate host defense peptides, etc. to name a few. The authors concluded that without sufficient funding, these novel treatment modalities to replace or supplement antibiotics will not become available in clinical practice, as funding currently is the key limiting factor that is stalling a global response to antibiotic resistance (Czaplewski et al. Citation2016).
Interestingly, in this review, the use of antimicrobial photodynamic therapy (aPDT) as an alternative approach instead of antibiotics and antiseptics was not mentioned (Czaplewski et al. Citation2016), although this term reveals 2287 citations in PubMed (National Centre for Biotechnology Information; status as of November 8 2017). This fact emphasizes that the potential for aPDT unfortunately has been underestimated or even been neglected. In addition, leading scientists in the field of aPDT have addressed this topic in a personal view article in Lancet Infectious Diseases, where the authors promoted to give funding not only for conventional antimicrobials but also for light-based approaches highlighting the potential of aPDT as an alternative antimicrobial approach in this upcoming era of resistance (Wainwright, Maisch, et al. Citation2017). The present review aims to provide a concise and critical overview about the current status quo of research on aPDT as well as on knowledge gaps and to offer an outlook on future research directions for translation of aPDT towards clinical practice.
Antimicrobial photodynamic therapy – what we know
History
The principle of photodynamic therapy (PDT) was accidentally discovered in 1900 by the medical student, Oscar Raab in Munich, who was involved in a study on the toxicity of the dye acridine red on Paramecium spp. as part of his doctoral thesis (Raab Citation1900).
The results of his experiments using acridine red in small concentrations were inconsistent and not reproducible, despite many replicates. However, he and his supervisor Hermann von Tappeiner recognized that the observed toxicity was dependent on the time of the day and the amount of daylight, since this had been the only changing parameter (Szeimies Citation2005). Later on, von Tappeiner applied this approach with Albert Jesionek clinically for treatment of skin carcinomas (Jesionek and Tappeiner Citation1905) and coined the term “photodynamic phenomenon” (Tappeiner Citation1909). Jodlbauer and von Tappeiner in 1904 and Huber in 1905 were the first to describe the successful photodynamic inactivation of bacteria (Jodlbauer and Tappeiner Citation1904; Huber Citation1905).
While anti-cancer PDT is clinical reality now for 25 years at least, e.g. in the treatment of actinic keratosis or basal cell carcinoma, antimicrobial applications of the photodynamic process have only been rediscovered in response to the emergence of the first drug-resistant infections in the healthcare sector during the early 1990s (Wainwright, Maisch, et al. Citation2017; Wainwright Citation2017a).
Fundamental mechanisms
The principle of aPDT is based on the combination of three compounds, a per se non-toxic molecule, the so-called photosensitizer (PS), light of a spectral range appropriate for excitation of the PS (typically from the visible to near infrared spectrum) and molecular oxygen (Wainwright Citation1998; Wainwright, Maisch, et al. Citation2017). shows the Jablonski diagram depicting the mechanism of aPDT: after absorption of a photon (A), the PS transfers from its ground singlet state (S0) to an excited singlet state (Sn). Subsequently, the PS can lose energy by emitting fluorescence (F) or heat (H) via internal conversion, thereby returning to S0, or it can be converted to a longer-living excited triplet state PS (T1) by so-called inter-system crossing (ISC). From this state, it can return to its ground state (S0) either by phosphorescence emission (P) or by two mechanisms generating reactive oxygen species (ROS), as follows: in type I mechanism, charge (i.e. electrons) is transferred to surrounding substrates leading to formation of superoxide radicals (O2−•) that can undergo dismutation into hydrogen peroxide (H2O2), the precursor of the highly reactive free hydroxyl radicals (HO•), which are formed via Fenton-like reactions. In contrast, in type II mechanism, energy (but no charge) is transferred directly to the ground state molecular oxygen (3O2), leading to emergence of singlet oxygen (1O2), which is nothing else than energized molecular oxygen (+0.98 eV = +1.57 × 10−19 J). After such a cycle of absorption and generation of ROS, the PS is in its ground state (S0) again and therefore ready for absorption of a new photon to generate more ROS. Accordingly, one PS molecule can generate thousands of molecules of 1O2 before being destroyed. The ratio of both photodynamic mechanisms is unique for each PS and depending on the respective chemical structure, whereby the singlet oxygen quantum yield ΦΔ describes the amount of type II mechanism (Maisch et al. Citation2007; Alves et al. Citation2014; Baptista et al. Citation2017; Wainwright, Maisch, et al. Citation2017).
Figure 1. Adapted Jablonski diagram showing the photochemical and photophysical mechanisms of antimicrobial photodynamic therapy (aPDT). S0: ground singlet state of the PS molecule; Sn: excited singlet state of the PS molecule; T1: triplet excited state of the PS molecule; A: absorption of light; F: fluorescence emission; H: heat generation (internal conversion); ISC: inter-system crossing; P: phosphorescence emission; 3O2: ground state oxygen; 1O2: singlet oxygen; O2−•: superoxide anion; HO•: hydroxyl radical; H2O2: hydrogen peroxide.
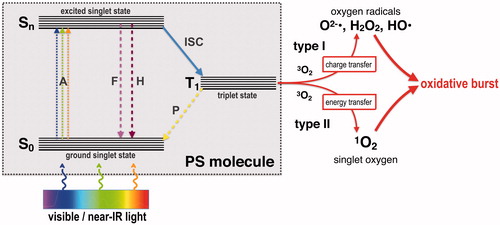
Photosensitizers
Since the discovery of the photodynamic process in the beginning of the 20th century, the development of appropriate “light-activatable dyes” was initiated for use as PS. Even before, several dyes had already been synthetized in Germany by the end of the 19th century (from the 1860s) in the context of a booming German textile industry (López-Muñoz et al. Citation2005). Within the last 20 years, several approaches were done to optimize the photodynamic properties of PS by modifying the chemical structure or by developing new classes of PS (Wainwright, Maisch, et al. Citation2017). In the following, main classes of PS used for aPDT are described shortly (chemical structures of the most used compounds are shown in ). For a more detailed description of all the distinct PS classes, the reader may be referred to the excellent review of Heidi Abrahamse and Michael R. Hamblin (Abrahamse and Hamblin Citation2016).
Figure 2. Selected main PS employed for aPDT. A: Methylene Blue; B: Toluidine Blue; C: TMPyP; D: chlorin-e6; E: fullerene C60; F: SAPYR.
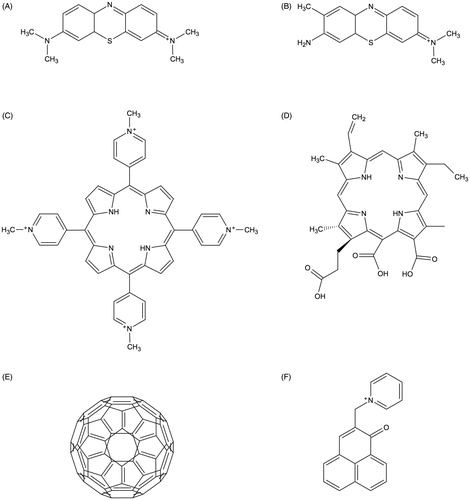
Phenothiazinium derivatives
The first phenothiazinium dye (Methylene Blue) was developed by Heinrich Caro in the 1870 s (Caro Citation1878). These dyes are composed of a three-ring π-system with auxochromic side groups, carry a single positive charge and exhibit a singlet oxygen quantum yield below 0.5, therefore acting mainly according to type I mechanism (Wilkinson et al. Citation1993). They show strong absorption in the red spectrum (≈ 600–680 nm), which is advantageous for their application as PS due to the better tissue penetration of light from longer wavelengths (Felgenträger et al. Citation2013). Examples for classic phenothiazinium-based PS employed in aPDT are Methylene Blue [3,7-bis(dimethylamino)-phenothiazin-5-ium chloride] or Toluidine Blue [3-amino-7-(dimethylamino)-2-methyl- phenothiazin-5-ium chloride], which have been continuously refined during the last 20 years by several chemical modifications (e.g. New Methylene Blue, Di-Methyl-Methylene Blue) (Wainwright et al. Citation1998; Wainwright Citation2000; Gollmer et al. Citation2015).
Impact in the field: first clinically approved PS (dentistry), standard PS in vitro
Porphyrin, chlorin, and phthalocyanine derivatives
Porphyrins, chlorins, and phthalocyanines are structurally comparable heterocyclic-macrocyclic compounds consisting of four pyrrole cycles (porphyrins, phthalocyanines) or three pyrrole and one pyrroline subunits (chlorins). Porphyrins and chlorins carry up to eight positive charges and act predominantly according to type II mechanism with singlet oxygen quantum yields between 0.5 and 0.8 (Fernandez et al. Citation1997). Furthermore, they exhibit an intense absorption maximum at ≈ 405 nm (Soret band) and smaller peaks at wavelengths longer than 500 nm (Q bands) (Gouterman Citation1961).
Many of these compounds are occurring in nature; for instance, it is well known that several oral bacteria form endogenous porphyrins in their metabolism, that is why they can be successfully inactivated by irradiation with visible light only in vitro (Cieplik, Späth, et al. Citation2014; Pummer et al. Citation2017). Examples for chemical modifications of porphyrins that are commonly used as PS are TMPyP [5,10,15,20-tetrakis(1-methyl-4-pyridinium)-porphyrin tetra-(p-toluenesulfonate], a four-fold positively charged derivative (Collins et al. Citation2010), and XF-73 [5,15-bis-[4–(3-trimethylammoniopropyloxy)-phenyl]-porphyrin chloride], a porphyrin derivative with two positive charges (Maisch et al. Citation2005). With regard to chlorins, mainly cationic derivatives of chlorin-e6 are used for aPDT, e.g. Photodithazine®, which is a commercially available chlorin-e6 derivative with two positive charges (Dovigo et al. Citation2013). In contrast to porphyrins and chlorins, phthalocyanines are hydrophobic and uncharged, wherefore they have to be combined with methods for solubilisation, e.g. encapsulation in nano-elmusions, to be used as effective PS for aPDT (Ribeiro et al. Citation2015).
Impact in the field: TMPyP widely used as standard PS in vitro
Xanthene derivatives
Anionic xanthene dyes like Eosin Y, Erythrosine and Rose Bengal are derived from fluorescein and show intense absorption in the green spectrum (≈ 480–550 nm) (DeRosa Citation2002; Goulart et al. Citation2010). Although they act mainly according to type II mechanism (singlet oxygen quantum yields ranging between 0.6 and 0.8) (Wilkinson et al. Citation1993), their negative charge impedes with successful use as PS for aPDT (Cieplik, Tabenski, et al. Citation2014).
Impact in the field: sparse studies in more recent years
Fullerene derivatives
Fullerenes are soccer-ball shaped cage molecules made exclusively from carbon atoms, with fullerene C60 being the most studied compound, which has been functionalized in multiple ways by adding positively charged moieties (Mizuno et al. Citation2011; Sharma et al. Citation2011). They show strong absorption in the UV and visible range due to their extended π-conjugated system of molecular orbitals and react predominantly according to both type I and type II mechanism depending on chemical modifications and solvents (Mroz et al. Citation2007).
Impact in the field: unique class of PS, sparse studies on effect on biofilms yet.
Phenalenone derivatives
Phenalenone chromophors are biosynthesized by plants to defend against pathogens using sun to generate singlet oxygen (Flors and Nonell Citation2006). Santi Nonell et al. were the first to report about PNS [1H-Phenalen-1-one-2-sulfonic acid] as an “extremely efficient singlet molecular oxygen sensitizer for aequeous media” reacting almost quantitatively according to type II mechanism (Nonell et al. Citation1993). From then, PNS was used as a standard-PS for photophysical measurements but was not applied for aPDT due to its negative charge. Later, several positively charged and water-soluble phenalen-1-one derivatives were introduced with SAPYR as their lead compound (carrying a positively charged pyridinium-methyl moiety) (Cieplik et al. Citation2013). These phenalen-1-one derivatives exhibit singlet oxygen quantum yield above 0.9 and absorb in the UV/blue spectrum (≈ 320–430 nm) (Späth et al. Citation2014; Tabenski et al. Citation2016).
Impact in the field: SAPYR as first water-soluble exclusive type-II PS.
Riboflavin derivatives
Riboflavin, i.e. vitamin B2, has already been known to generate singlet oxygen upon UVA irradiation (Regensburger et al. Citation2012). However, hydrophilicity was very poor due to the lack of positive charges leading to unexceptional antimicrobial efficacy (Makdoumi and Bäckman Citation2016). Recently, riboflavin derivatives substituted with up to eight positive charges showed considerable improvement in photodynamic efficacy (Maisch et al. Citation2014). These novel riboflavin derivatives show absorption in the UVA/blue spectrum (≈ 350–470 nm) and singlet oxygen quantum yields between 0.7 and 0.8 (Maisch et al. Citation2014).
Impact in the field: safe in humans, as decomposition of flavin molecules is ubiquitous
Curcumin derivatives
Curcumin [1E,6E-1,7-bis(4-hydroxy-3-methoxyphenyl)-1,6- heptadiene-3,5-dione] is a naturally occurring intense yellow dye isolated from the rootstocks of Curcuma longa and approved as food additive E100. Due to its poor hydrophilicity, several chemical modifications of curcumin carrying positive charges have been developed recently (Tortik et al. Citation2014; Spaeth et al. Citation2017). Curcumin and its derivatives act nearly quantitative according to type I mechanism and exhibit absorption in the UV/blue spectrum (≈ 300–500 nm) (Araújo et al. Citation2014).
Impact in the field: curcumin approved as food additive, novel positively charged derivatives with enhanced water solubility
Light sources
For light-activation of a PS, three main light sources have been described for the field of aPDT in the literature so far: lasers (e.g. argon, diode, or neodymium doped: yttrium, aluminum, and garnet [Nd:YAG] lasers), light-emitting diodes (LEDs) and gas-discharge lamps (e.g. quartz-tungsten-halogen or xenon-discharge lamps) (Wilson and Patterson Citation2008; Nagata et al. Citation2012). Nagata et al. and Wilson and Patterson have detailed the advantages and disadvantages of these light sources (Wilson and Patterson Citation2008; Nagata et al. Citation2012):
Lasers are mainly applied due to their monochromaticity rather than for their other properties (i.e. coherence, parallel beam propagation, narrow spacial intensity). Therefore, it can be easily coupled into a single optical fiber and mounted on different light delivery devices. Compared to lasers, LEDs deliver a slightly wider emission spectrum and their costs are lower (Wilson and Patterson Citation2008). In contrast to lasers and LEDs, halogen lamps have the advantage that they can be spectrally filtered to match any PS; however, they cannot be efficiently coupled into optical fiber bundles or liquid light guides, and they cause more heating as compared to lasers and LEDs (Nagata et al. Citation2012). Heating caused by a given light source must be considered for any clinical application of aPDT in the human body. Depending on the irradiation period and the respective light source used, the energy dose applied can cause a temperature increase, potentially leading to tissue damages (Spranley et al. Citation2012). For example, irradiation of teeth with a halogen lamp for 45–60 s increased the temperature of dentin by around 5 °C (Weerakoon et al. Citation2002), while an increase in temperature of 5.5 °C is known to have adverse effects on the dental pulp (Nammour et al. Citation2010; Nagata et al. Citation2012).
All in all, for irradiation of a given PS, the spectral emission and the intensity of the respective light source as well as the mode of light-delivery (via optical fiber or directly) are more important than the type of the light source itself, e.g. laser, LED, or gas-discharge lamp.
Antimicrobial efficacy of aPDT in vitro
The antimicrobial efficacy of aPDT employing many distinct PS had been investigated in a very large number of in vitro studies since the 1990s. Thereby, many of these studies showed promising results, often achieving inactivation rates of more than 5 log10 steps of CFU (colony forming units), which is defined as a disinfecting effect according to infection control guidelines (Boyce and Pittet Citation2002).
In this instance, it must be considered that many of these studies have been conducted for inactivation of planktonic cultures, i.e. free-floating bacterial cells, which is a convenient method for high-throughput antimicrobial screening of many compounds, e.g. in order to evaluate whether slight chemical modifications of a given PS can enhance antimicrobial efficacy (Kiesslich et al. Citation2013).
However, for evaluating the efficacy of a given antimicrobial approach it is mandatory to study biofilms, as it is well known that bacteria embedded in biofilms exhibit very different properties as compared to their planktonic counterparts like an up to 1000-fold higher tolerance towards antimicrobials (Marsh Citation2004; Marsh et al. Citation2011). Besides all the other factors responsible for this higher tolerance of bacteria in biofilms, especially the biofilm matrix (extracellular polymeric substance; EPS) may protect the bacteria against aPDT-mediated killing in two-fold way by preventing penetration of the PS throughout the biofilm (interactions of positively charged PS molecules with negatively charged EPS residues, redox processes, and π–π-interactions of aromatic surfaces) and by hampering light propagation in deeper layers of the biofilm (Mah and O’Toole Citation2001; Stewart and Costerton Citation2001).
For an overview of the antimicrobial efficacy of aPDT for inactivation of bacterial biofilms in vitro, the reader may be kindly referred to a recent review of our group, where antimicrobial efficacy rates of distinct PS are elaborated according to the respective classes of PS in detail (Cieplik, Tabenski, et al. Citation2014).
With regard to fungi, it is also well known that fungal biofilms are much more tolerant than planktonic fungal cultures (Lyon et al. Citation2011). For aPDT, the majority of studies has focused on Candida biofilms (Baltazar et al. Citation2015). Since the antifungal action of aPDT seems to be strain-dependent (Lyon et al. Citation2011), there is need to investigate the efficacy of aPDT also for biofilms of other major fungal pathogens (Baltazar et al. Citation2015). For more detailed insights into the effects of aPDT on fungal cells, it may also be referred to recent reviews in the literature (Lyon et al. Citation2011; Pinton et al. Citation2012; Baltazar et al. Citation2015). Interestingly, mixed bacterial-fungal biofilms differ from purely bacterial or fungal biofilms because fungi represent biotic surfaces to which bacteria can adhere (Seneviratne et al. Citation2007). Such mixed biofilms may be more resistant than biofilms formed solely from bacteria or fungi, e.g. due to a more complex EPS composition (Wargo and Hogan Citation2006).
Appropriate comparison of distinct aPDT systems in vitro
When the antimicrobial efficacy of distinct PS is to be compared, it is crucial to adjust the respective irradiation parameters. Hereby, the distinct absorption and emission characteristics of given PS and their corresponding light sources must be considered. As according to the photodynamic principle one PS molecule is excited by the absorption of one photon, adjusting the number of photons absorbed by a PS molecule upon irradiation by its corresponding light source seems appropriate to compare the antimicrobial photodynamic efficacy rates of distinct PS per excited molecule (Cieplik et al. Citation2015). The number of absorbed photons per second is given by the following equation:
Table
Adjusting numbers of absorbed photons is the only way to take characteristics of both, the PS and the light source, into account, while other ways for adjustment of irradiation parameters (e.g. energy doses) only consider the characteristics of the light source, but not the PS. Therefore, applying this formula ensures that the biological efficacy of distinct PS can be compared independent of their photo-physical absorption characteristics (Cieplik et al. Citation2015, Citation2016).
Putative main target structures of aPDT
A particular advantage of aPDT is its non-selective action, focusing on multiple molecular targets like proteins, lipids, and nucleic acids (Almeida et al. Citation2015). Usually, oxidative damage of two crucial cellular components, i.e. cytoplasmic membranes and DNA, is proposed to be responsible for aPDT-mediated killing of bacteria (Hamblin and Hasan Citation2004; Alves et al. Citation2014). These oxidative targets of aPDT mostly depend on the respective cellular localization of the given PS as well as on the maximal time-limited diffusion length of the emerging ROS. For instance, due to its short lifetime of 3–4 µs, the diffusion length of 1O2 is not longer than 0.3 µm and depending on the surrounding medium (Baier et al. Citation2005; Maisch et al. Citation2007). Therefore, oxidative damage usually takes place in close vicinity to the respective PS molecule, for which there are three options in general (Alves et al. Citation2014):
A PS just stays in close proximity to a bacterial cell without any effective binding, which may limit the oxidative damage to outer structures like the cell wall or the cytoplasmic membrane, or
A PS actively binds to the bacterial cell via electrostatic attraction, hydrogen bonds, or van-der-Waals interactions, potentially leading to a similar damage pattern limited to outer structures, or
A PS enters a bacterial cell by binding and subsequent diffusion or active transport into the cytoplasm, resulting in damage of intracellular components like cytoplasmic proteins or DNA.
Localization of a PS may be dependent on the chemical structure of the respective PS (e.g. molecular size, charge, lipophilicity), its concentration, solvent as well as the cell wall structure of the targeted bacteria (e.g. Gram-staining characteristics) (Alves et al. Citation2014). However, these binding mechanisms and the cellular localizations of given PS are not fully understood yet and reports are contradictory (Ragàs et al. 2010; Preuß et al. Citation2013; Alves et al. Citation2014; Mang et al. Citation2016; Gollmer et al. Citation2017). For instance, while Ragas et al. reported a dual localization of the porphyrin-PS TMPyP inside (bound to DNA) and outside (attached to the cell wall) in Escherichia coli based on spectroscopic and photophysical measurements (Ragàs et al. Citation2010), Preuß et al. found no uptake of TMPyP in E. coli as measured by fluorescence lifetime imaging technique (Preuß et al. Citation2013). In accordance to the latter study, Gollmer et al. revealed by highly-sensitive fluorescence microscopy that accumulation of TMPyP in the cytoplasm and the cell wall is minor in S. aureus and that this PS may only enter the cell after preceding cell wall damage (Gollmer et al. Citation2017). On the contrary, the phenothiazinium dye Methylene Blue seems to locate intracellularly because its photodynamic efficacy can be increased by addition of efflux pump inhibitors (Tegos et al. Citation2008).
Nevertheless, for damage of DNA, a PS has to build up high-intracellular concentrations (Cadet et al. Citation2010). Accordingly, it seems reasonable that main targets are the external structures like cell walls and cytoplasmic membranes rather than DNA (Almeida et al. Citation2015). Accordingly, from classic studies on the extremophile bacterium Deinococcus radiodurans, it is known that this species can be easily inactivated by aPDT, although it is known for its very efficient DNA repair mechanisms allowing to withstand even very high amounts of ionizing radiation (Schäfer et al. Citation1998; Nitzan and Ashkenazi Citation1999). In contrast, nucleic acids may only be affected when bacterial cell walls and cytoplasmic membranes have already been destroyed by the oxidative burst generated by aPDT treatment (Almeida et al. Citation2015). Attachment of the positively charged PS to the negatively charged cell wall and membrane structures of bacteria seems more probable than actual uptake of the PS. However, this is dependent on the chemical structure of the respective PS, its predominant mechanism of action and probably also on the targeted bacterial species. For further insights on cellular targets of aPDT in bacteria, the reader may be referred to the excellent review of Alves et al. (Citation2014).
Potential for development of resistances towards aPDT
In general, development of resistances in pathogens is more likely for approaches that aim at one specific target structure according to the so-called key-lock principle like antibiotics or most antiseptics (McDonnell and Russell Citation1999; Maillard Citation2002). In this instance, it is easy for pathogens to overcome the antimicrobial challenge by punctual mutations, expression of efflux pumps, or up-regulation of defense-associated enzymes. As stated above, aPDT is a non-selective approach with multiple targets in focus. Therefore, it has been assessed to be very improbable that bacteria could develop resistance towards aPDT (Maisch Citation2015a; Wainwright, Maisch, et al. Citation2017).
Bacterial response towards oxidative stress generated by aPDT
Oxidative stress is somehow ubiquitous in bacterial life, either formed intracellularly in bacterial metabolism or due to interaction with host cells or cells from other bacterial species. While molecular oxygen (O2) is rather unreactive, it can cross typical biological membranes very quickly (Imlay Citation2013). Its reactivity derives from the metabolic formation of ROS due to a four-electron reduction series with the addition of consecutive electrons generating superoxide anions (O2−•), hydrogen peroxide (H2O2) and free hydroxyl radicals (•OH) (Gerschman et al. Citation1954; Imlay Citation2013). These ROS are known to be able to oxidize various biomolecules and cause substantial damage. The condition of accumulation of ROS inside a cell is called oxidative stress (Imlay Citation2013). Bacteria have developed defense mechanisms for detoxification of these ROS by highly specific and effective enzymatic pathways like those catalyzed by superoxide dismutase (Sod), catalase, peroxidase as well as enzymes of the glutathione system (Miller and Britigan Citation1997; Pomposiello and Demple Citation2002; Staerck et al. Citation2017).
Theoretically, bacteria may be able to adapt towards ROS generated from type I mechanism in aPDT by up-regulating expression of these enzymes (Maisch Citation2015a; Kashef and Hamblin Citation2017). Nakonieczna et al. observed that Sod activity as well as transcript levels of sodA and sodM increased in clinical isolates of S. aureus after aPDT with protoporphyrin IX, but only in strains that were found susceptible to aPDT. This emphasizes that Sod activity may not directly affect the vulnerability of S. aureus towards aPDT (Nakonieczna et al. Citation2010). As oxidative damage only occurs at the site of the localization of a PS, the distance between the oxidative burst (most probably occurring at the cell envelope as described above) and these intracellularly located defense enzymes may be too long, wherefore these enzymes may not be able to help bacteria to a significant extent to survive aPDT challenge (Maisch Citation2015a).
In contrast, against singlet oxygen (1O2) generated from type II mechanism of aPDT, there is no specific enzyme-mediated defense system known in bacteria. Furthermore, it is very improbable that bacteria could develop such a system as 1O2 is no oxygen radical, but just energized molecular oxygen (+0.98 eV). However, it is known from plants, that chemical quenchers of 1O2 (mainly carotenoids, particularly β-carotene, and α-tocopherol) are formed during photosynthesis when more light energy is absorbed than can be used (Krieger-Liszkay Citation2005; Ramel et al. Citation2012). Further research on this phenomenon in bacteria may be worthwhile.
Expression of efflux pumps
Another major mechanism of bacterial resistance is given by transmembrane efflux systems, which lead to a decrease of the intracellular concentrations of noxious compounds by extrusion in the extracellular environment (Alekshun and Levy Citation2007). This may also be an issue for resistance towards aPDT (Tegos and Hamblin Citation2006; Tegos et al. Citation2008) as it has been shown that efflux pumps reduce killing efficacy of aPDT with Methylene Blue by decreasing intracellular concentrations of Methylene Blue and associated intracellular ROS (Tegos et al. Citation2008; Rineh et al. Citation2017). However, although many classes of PS have been tested so far (Wainwright, Maisch, et al. Citation2017), only phenothiazinium compounds like Methylene Blue have been found to be potential substrates for efflux pumps in bacteria (Tegos and Hamblin Citation2006), but also in Candida albicans (Prates et al. Citation2011). This reduced antimicrobial photodynamic efficacy due to efflux and can nonetheless be overcome by adjunctive application of specific efflux pump inhibitors, as it has been shown for planktonic cultures (Tegos et al. Citation2008; Kishen et al. Citation2010) and biofilms (de Aguiar Coletti et al. Citation2017) in vitro as well as in an in vivo murine wound infection model (Rineh et al. Citation2017, Citation2018).
Studies on induction of resistance towards aPDT
Development of resistance is generally studied by applying sublethal doses of given treatment modalities and subsequent culture of the surviving bacteria in repeated cycles (Alves et al. Citation2014). There have been very few studies so far that tried to induce resistances in pathogens towards aPDT by such repeated passages of treatment and re-growth (Lauro et al. Citation2002; Pedigo et al. Citation2009; Giuliani et al. Citation2010; Tavares et al. Citation2010). Lauro et al. showed that Aggregatibacter actinomycetemcomitans and Peptostreptococcus micros were not able to develop detectable resistance towards aPDT with porphycene-polylysine conjugates as PS after 10 repeated cycles of partial inactivation followed by subsequent culture of outliving bacteria (Lauro et al. Citation2002). Further, this aPDT treatment did also not modify the susceptibility towards various classes of antibiotics (Lauro et al. Citation2002). Pedigo et al. investigated the potential of E. coli and antibiotic-sensitive and resistant strains of S. aureus (MSSA and MSRA) to develop resistance towards repeated aPDT treatment with MB as a PS. For both species, no differences were observed when tested through 11 (E. coli) or even 25 passages (MSSA, MRSA), respectively (Pedigo et al. Citation2009). Tavares et al. came to the same result with strains of Vibrio fischeri and E. coli after 10 cycles of aPDT with the tricationic porphyrin derivative Tri-Py+-Me-PF (Tavares et al. Citation2010). Likewise, Giuliani et al. found no development of resistance even after 20 daily passages of aPDT using the tetracationic Zn(II)-phthalocyanine derivative RLP068/Cl as PS on S. aureus, Pseudomonas aeruginosa and Candida albicans (Giuliani et al. Citation2010).
However, it must be considered that there are two fundamental limitations of these studies that should be kept in mind: First, these studies have been conducted on bacteria (or yeasts) in planktonic cultures (Lauro et al. Citation2002; Pedigo et al. Citation2009; Giuliani et al. Citation2010; Tavares et al. Citation2010). In contrast, when bacteria are in a sessile biofilm-mode, as it is their preferred way of life in vivo, the risk of development of resistances generally is higher than when growing as planktonic cultures, e.g. via horizontal gene transfer (Boles et al. Citation2004; Marsh et al. Citation2011). Besides that, it is known that bacteria acquire resistance towards a given antimicrobial when they are continuously exposed to low, i.e. sub-antimicrobial, doses for a prolonged period while aPDT is designed to be a relatively short procedure. Consequently, Kashef and Hamblin suggested that a more realistic way to investigate the potential of resistance towards aPDT would be to expose bacteria to continuous defined sub-antimicrobial levels of aPDT (Kashef and Hamblin Citation2017).
Having these limitations in mind, aPDT treatment seems not to induce resistance towards antibiotics: Cassidy et al. exposed clinical isolates of S. aureus and P. aeruginosa to sublethal aPDT with TMPyP and Methylene Blue as PS for a total of 72 h (irradiation at t = 0, 24, and 48 h) for habituation to aPDT and afterwards tested susceptibility towards various antibiotics as well as aPDT. They found no decreased susceptibility towards antibiotics nor aPDT (Cassidy et al. Citation2010).
Potential fields for clinical application of aPDT
In a very recent review, Mark Wainwright drew the example of tonsillitis, a very common disease of bacterial and/or viral origin, which is usually treated by systemic prescription of amoxicillin without clarifying its etiology beforehand. For this disease, application of a photoantimicrobial followed by irradiation for a given period, for instance by means of a fiber-optic device, may lead to sufficient antimicrobial effects without any risk of disturbance of the intestinal microbiota by systemic antibiotics or exposure of bacteria to sub-inhibitory concentrations that potentially may induce resistance (Wainwright Citation2017b). However, until now only three PS have reached clinical approval for use in humans in combination with light, i.e. phenothiazinium dyes, Methylene Blue and Toluidine Blue as well as heptacyanine dye Indocyanine Green (Wainwright, Maisch, et al. Citation2017), which rather reacts according to a photothermal than a photodynamic mechanism (Engel et al. Citation2008). Furthermore, these systems are only approved for use in dentistry as adjuvant approach for treatment of periodontal or endodontic infections (Wainwright, Maisch, et al. Citation2017).
In general, application of aPDT may work best for localized infections, preferably at easy accessible parts of the body, where light propagation is no problem (Wainwright, Maisch, et al. Citation2017; Wainwright Citation2017b). Nevertheless, with the aid of endoscopes and fiber-optic devices, most regions of the body are accessible for local application of PS and subsequent irradiation, e.g. ear, nose, oral cavity, gastrointestinal tract, urogenital tract or lungs (Wainwright, Maisch, et al. Citation2017). In the following paragraphs, two major fields for clinical application of aPDT are summarised:
Oral infections
In dentistry, there may be several conditions, where application of aPDT could be useful, either for restricting the use of antibiotics or for improving treatment outcomes (Gursoy et al. Citation2013): in periodontology, systemic use of antibiotics is a common treatment modality for severe cases of periodontitis in order to ensure favorable treatment outcomes (Slots Citation2004). As this is discussed more controversially nowadays (Preus et al. Citation2014), there has recently been an increasing interest in alternative approaches like aPDT (Gursoy et al. Citation2013). There have been some clinical studies on the effects of aPDT on the healing outcomes when applied as an adjunct to mechanical treatment of severe chronic (Tabenski et al. Citation2016) or aggressive periodontitis (Arweiler et al. Citation2014). Most of these clinical trials indicate substantially better clinical short-term healing outcomes with adjunctive aPDT in terms of reduced bleeding on probing and reduction of probing pocket depths as compared with mechanical treatment alone (Sgolastra et al. Citation2013; Sculean et al. Citation2015). Altogether, the clinical data about aPDT in periodontics is still limited and the outcomes are to some extent controversial as well (Sculean et al. Citation2015). Accordingly, in a recent systematic review, it was concluded that evidence supporting clinical long-term benefits of aPDT as an adjunct for treatment of periodontitis is not sufficient yet (Sgolastra et al. Citation2013). In contrast, it was recently concluded in a systematic review that use of aPDT in combination with mechanical debridement definitely brings significant improvements in patients suffering from peri-implantitis (Sivaramakrishnan and Sridharan Citation2018).
In endodontics, aPDT may be a valuable add-on for disinfection of heavily infected root canals or in view of so-called “single-visit endodontics” (Chrepa et al. Citation2014). Garcez et al. reported that adjunctive aPDT leads to a significant further reduction of bacterial load in root canals (Garcez et al. Citation2010). In addition, histopathological studies in dogs indicated more favorable healing of periapical osseous lesions and less inflammation after additional treatment with aPDT (Silva et al. Citation2012; Borsatto et al. Citation2016). Likewise, in a recent randomized clinical trial, it was found that additional application of aPDT resulted in better radiographic healing of primary endodontic infections six months after endodontic treatment as compared to conventional endodontic therapy alone (de Miranda and Colombo Citation2018).
There are also some clinical studies and case reports on the efficacy of aPDT for treatment of oral fungal infections, e.g. oral candidiasis and denture stomatitis (Javed et al. Citation2014), where aPDT was found to be as effective as standard antifungal treatment (Mima et al. Citation2012; Alves et al. Citation2017). Furthermore, aPDT was also suggested as an additional antimicrobial measure for treatment of deep carious lesions when carious dentin is only partially removed (Cieplik et al. Citation2017). However, huge difference in study design and quality (e.g. lack of controls) as well as the fact that almost only phenothiazinium dyes but no other classes of PS have been used as PS in clinical studies do not allow for general conclusions on the clinical efficacy of aPDT for treatment of oral infections.
Skin infections and chronic wounds
In dermatology, aPDT may be a valuable treatment modality for all kinds of skin infections from bacterial as well as fungal origin, but especially for treatment of chronic wounds (Maisch et al. Citation2004; Brown Citation2012; Baltazar et al. Citation2015). In this context, it is noteworthy that beneficial effects of aPDT on host tissues have been proposed (but not yet definitely confirmed), such as stimulation of growth factors and immune response, potentially leading to enhanced wound healing, which may be valuable for the application of aPDT for treatment of chronic wounds or ulcers (Brown Citation2012).
In the first randomized, placebo-controlled clinical trial on aPDT in chronic wounds (chronic leg ulcers and diabetic foot ulcers), Morley et al. showed significant and broad-spectrum bacterial cell kill plus a promising trend towards accelerated wound healing employing the phenothiazinium derivative PPA904 as a PS (Morley et al. Citation2013). Likewise, when Lei et al. investigated the effects of ∂-aminolevulinic acid-mediated aPDT for treatment of chronic skin ulcers in lower limbs infected with P. aeruginosa (as compared to treatment with red light alone), they found significantly better healing outcomes in the aPDT group in terms of reduction in the mean ulcer area (Lei et al. Citation2015). Use of aPDT was even suggested to be an option for diabetic foot ulcers for prevention of amputations (Tardivo et al. Citation2014). Tardivo et al. found in a randomized clinical trial that the amputation rate in the group treated with aPDT with phenothiazinium dyes Methylene Blue and Toluidine Blue was only 2.9% the amputation rate in the control group without aPDT (aPDT: 1 amputation out of 18 patients; control: 16 amputations out of 16 patients) (Tardivo et al. Citation2014). aPDT may even prevent surgical debridement of diabetic foot ulcers and is considered as an efficient and affordable treatment method for the diabetic foot (Tardivo et al. Citation2017).
Future research directions (what we don’t know yet)
The “perfect” aPDT system
A “perfect” aPDT system should combine high antimicrobial efficacy without any serious side-effects for patients. Furthermore, its handling should be as easy as possible. For this instance, characteristics of both, the PS as well as the corresponding light source, must be considered. Nowadays, it is agreed that a PS should combine the following features (adopted and modified from (Cieplik, Tabenski, et al. Citation2014; Maisch Citation2015b)):
Hydrophilicity and positive charge(s) for high-affinity to binding or attachment towards negatively charged bacterial cell walls (Alves et al. Citation2009)
Low-molecular weight for facilitating penetration through biofilm structures (Cieplik et al. Citation2013)
High 1O2 quantum yield, reaction according to type II mechanism (Maisch et al. Citation2007; Cieplik et al. Citation2015)
High-photostability during irradiation (Engel et al. Citation2008)
No toxicity or mutagenicity in the dark towards eukaryotic cells as well as microorganisms (Soukos and Goodson Citation2011)
“Therapeutic window” or effective concentration range, where microorganisms can be killed sufficiently without damage of eukaryotic cells (Muehler et al. Citation2017)
Recently, it was further discovered by the group of Michael R. Hamblin that the antimicrobial efficacy of given PS used for aPDT (e.g. Methylene Blue, Rose Bengal) can be potentiated by the addition of simple inorganic salts (Hamblin Citation2017), in particular potassium iodide (Vecchio et al. Citation2015; Huang et al. Citation2017; Wen et al. Citation2017). The proposed mechanism for this potentiating effect of iodide is likely due to reaction of singlet oxygen with iodide and formation of iodine radicals, hydrogen peroxide, and molecular iodine (Hamblin Citation2017).
For a light source to be employed in aPDT, it is crucial that its emission spectrum fits for the absorption spectrum of the respective PS used. Another vital point is the ability of the emitted light to penetrate biological tissues (e.g. skin) to such an extent that the appropriate tissue depth can be reached to be effective, which may even be more complicated in the presence of blood. Due to less scattering and absorption of biological tissues at higher wavelengths, near-infrared light (700–2500 nm) penetrates these tissues more efficiently than visible light, while at wavelengths longer than 950 nm, this effect is weakened due to increased absorption of water and lipids (Smith et al. Citation2009). Therefore, light from a window from 650–950 nm may be regarded as optimal (Smith et al. Citation2009). Furthermore, recently Piazena et al. reported local maxima of spectral transmittance for human skin at central wavelengths of 1082 nm and 1268 nm (Piazena et al. Citation2017). Besides that, it would be particularly favorable to use a light source with beneficial effects on eukaryotic tissues. For example, recently a light source was introduced emitting visible and water-filtered infrared-A light (Al-Ahmad et al. Citation2013; Karygianni et al. Citation2014; Al-Ahmad et al. Citation2015; Al-Ahmad et al. Citation2016), which is known for its wound healing capabilities (Hoffmann et al. Citation2016). This light source is designed in such a way that the whole incoherent radiation of a 3000 Kelvin halogen bulb is filtered by passing a cuvette containing water. The water filter absorbs some bands within infrared A and most parts of infrared B (1400–3000 nm) and C (3000–1000 000 nm), which leads to elimination of the thermal burden to the surface of the tissue caused by the reaction of the filtered components with the water content of biological tissues (Fuchs et al. Citation2004; Jung and Grune Citation2012). The remaining wIRA penetrates to deeper layers of the tissue and causes therapeutically beneficial warming (+2.7 °C at a tissue depth of 2 cm), an increase of the oxygen partial pressure (+32% at a tissue depth of 2 cm) and blood circulation, and subsequently stimulates the metabolism of the cells including the immune reaction (Fuchs et al. Citation2004; Hartel et al. Citation2006; Mercer et al. Citation2008; Felbert von et al. Citation2010; Schumann et al. Citation2011).
Nevertheless, it must be considered in this regard that many PS with proven in vitro efficacy, such as phenalenones and porphyrins, exhibit their main absorption in the violet/blue spectrum (i.e. 400–500 nm) (Cieplik, Tabenski, et al. Citation2014). A potential approach to combine these PS with light from longer wavelengths with good tissue penetration capabilities may be the use of photon upconversion (UC), which refers to a process where sequential absorption of two or more photons leads to the emission of light at shorter wavelength than the excitation wavelength (Haase and Schäfer Citation2011). In recent years, high-quality UC-nanocrystals with controlled solubility, particle size, and optical properties have been synthesized (Haase and Schäfer Citation2011). Therefore, applying these UC-nanocrystals may allow for combining the benefits of effective PS with absorption in the violet/blue spectrum with the better tissue penetration properties of light from longer wavelengths (Hamblin Citation2018). Thus, linking aPDT and UC may merit further investigation.
Investigating the mechanism of aPDT
For evaluating the potential of development of resistances in pathogens towards aPDT, it is crucial to narrow down its mechanism and find the actual main target structures that are damaged or destroyed by the oxidative burst induced by aPDT. For this purpose, mostly biochemical methods and transmission electron microscopy have been employed so far because the use of fluorescence microscopy was limited by the small size of bacteria and the faint fluorescence properties, i.e. photo-bleaching, of many PS (Alves et al. Citation2014; Gollmer et al. Citation2017). However, as stated above, very recently Gollmer et al. were the first to apply novel highly-sensitive fluorescence microscopy to study the incorporation of PS inside bacteria (in this case: S. aureus) taking advantage of fluorescence enhancement of Methylene Blue and TMPyP upon entering the bacterial cytoplasm after aPDT-mediated oxidative deterioration of the cell wall and cytoplasmic membrane (Gollmer et al. Citation2017). This technique merits further investigations on distinct classes of PS, but also on more bacterial species with different Gram-staining characteristics.
Another versatile method may be the use of flow cytometry in combination with appropriate fluorescence dyes to assess distinct vital parameters of bacterial cells (Nebe-von Caron et al. Citation1998; Joux and Lebaron Citation2000). While commercially available as kits (e.g. LIVE/DEAD™ BacLight™ Bacterial Viability Kit, Molecular Probes) are often used in fluorescence microscopy, these dyes can also be employed for flow cytometry for quantitative assessment. Combinations of dyes like SYTO-9 and propidium iodide, for instance, can give information whether major damage of cytoplasmic membranes occurs due to a given treatment (Tawakoli et al. Citation2013; Cieplik, Steinwachs, et al. Citation2018). Many other fluorescent dyes are available assessing metabolic activity, membrane potential, or intracellular pH-values (Novo et al. Citation2000; Ambriz-Aviña et al. Citation2014). Flow cytometry applying many distinct fluorescent dyes, so called multi-parameter flow cytometry, therefore may be used for assessing the effects of aPDT on distinct cellular structures in a semi-quantitative approach (Novo et al. Citation2000).
These methods can be combined with the more traditional electron microscopic techniques, which allow for additional visualization. For instance, changes in the appearance of the cytoplasm (vacuolations, condensations, etc.) or of cytoplasmic membranes can be envisioned by means of transmission electron microscopy (Wood et al. Citation1999).
All in all, for deeper insights into the mechanism and damage patterns of aPDT towards bacteria distinct methods must be combined in order to resolve these complex mechanisms. Furthermore, it is mandatory to not only test one class of PS, as the sites of accumulation as well as the photo-physical properties (e.g. singlet oxygen quantum yields) of given PS can vary widely and will have huge impact on the damage patterns of aPDT.
Translation in clinical practice
While there has been a discovery void of conventional antibiotics and antiseptics during the last 20 years, lots of new classes of PS, such as phenalenones, fullerenes, or derivatives of naturally occurring porphyrins, chlorins, and curcumins, have been developed (Wainwright, Maisch, et al. Citation2017), whereby many of them have shown promising results in in vitro studies so far (Cieplik, Tabenski, et al. Citation2014; Maisch Citation2015b). However, as stated above, many of these studies have been conducted using planktonic cultures, while it is well known that bacteria organized in biofilms exhibit an up to 1000-fold higher tolerance towards antimicrobials (Marsh Citation2004; Marsh et al. Citation2011).
For example, Methylene Blue, one of the three PS with clinical approval, has shown high efficacy in studies on planktonic bacteria (Gollmer et al. Citation2015; Núñez et al. Citation2015; Cieplik et al. Citation2016), but seems to be much less effective for inactivation of biofilms (Cieplik, Tabenski, et al. Citation2014). In a recent study from our group, we compared the antimicrobial photodynamic efficacy of Methylene Blue and the phenalenone derivative SAPYR for inactivation of monospecies biofilms of Enterococcus faecalis and Actinomyces naeslundii and found killing rates of about 5 log10 steps for SAPYR while Methylene Blue exhibited no reduction at all, when the number of absorbed photons were adjusted to equal amounts for both PS (Cieplik et al. Citation2015). Therefore, the clinical potential of aPDT may still be underestimated as many more effective PS, e.g. SAPYR, are not available for clinical application yet. This should be considered, when data from clinical studies, for instance in periodontology, is assessed.
For further pre-clinical evaluation of novel aPDT systems, it may be valuable to conduct studies in more complex biofilm models, which rather simulates the biofilms found in vivo in the oral cavity, on skin surfaces, in wounds, or on catheters. Therefore, instead of the currently used in vitro biofilm models comprising up to 10–15 species (Guggenheim et al. Citation2001; Belibasakis and Thurnheer Citation2014; Tabenski et al. Citation2014; Cieplik, Steinwachs, et al. Citation2018; Cieplik, Wimmer, et al. Citation2018), microcosm biofilms cultured from patient samples (Sissons Citation1997; Exterkate et al. Citation2010; Fernandez et al. Citation2017) or biofilms grown in situ (Karygianni et al. Citation2014; Al-Ahmad et al. Citation2015; Al-Ahmad et al. Citation2016) may represent the vast complexity of naturally occurring biofilms to a greater extent. Furthermore, animal models may be valuable as well in order to evaluate the clinical efficacy of aPDT, e.g. for treatment of periodontitis (de Oliveira et al. Citation2010) or infected burn wounds (Hamblin et al. Citation2002; Demidova et al. Citation2005). Finally, randomised clinical trials are mandatory. Thus, availability of funding is crucial, but still is limited for the field of aPDT, at least when compared to research on conventional antimicrobials (Czaplewski et al. Citation2016; Wainwright, Maisch, et al. Citation2017).
For later clinical application, the costs of a given new antimicrobial approach must be considered as well in relation to conventional antimicrobials on the market. An effective aPDT system, which is too expensive for under-developed countries or even emerging economies, will precede the uncontrolled over-the-counter purchase of conventional antimicrobial drugs in these countries, which in turn is known to contribute to the development of antibiotic resistance (Morgan et al. Citation2011).
Acknowledgements
Karl-Anton Hiller is gratefully acknowledged for helpful discussions regarding this manuscript.
Disclosure statement
All authors declare that they have no conflict of interest.
Additional information
Funding
References
- Abrahamse H, Hamblin MR. 2016. New photosensitizers for photodynamic therapy. Biochem J. 473:347–364.
- Al-Ahmad A, Bucher M, Anderson AC, Tennert C, Hellwig E, Wittmer A, Vach K, Karygianni L. 2015. Antimicrobial photoinactivation using visible light plus water-filtered infrared-A (VIS + wIRA) alters in situ oral biofilms. PLoS One. 10:e0132107.
- Al-Ahmad A, Tennert C, Karygianni L, Wrbas KT, Hellwig E, Altenburger MJ. 2013. Antimicrobial photodynamic therapy using visible light plus water-filtered infrared-A (wIRA). J Med Microbiol. 62:467–473.
- Al-Ahmad A, Walankiewicz A, Hellwig E, Follo M, Tennert C, Wittmer A, Karygianni L. 2016. Photoinactivation using visible light plus water-filtered infrared-A (vis + wIRA) and chlorine e6 (Ce6) eradicates planktonic periodontal pathogens and subgingival biofilms. Front Microbiol. 7:1900.
- Alekshun MN, Levy SB. 2007. Molecular mechanisms of antibacterial multidrug resistance. Cell. 128:1037–1050.
- Almeida A, Faustino MAF, Tomé JP. 2015. Photodynamic inactivation of bacteria: finding the effective targets. Future Med Chem. 7:1221–1224.
- Alves E, Costa L, Carvalho CM, Tomé JP, Faustino MA, Neves MG, Tomé AC, Cavaleiro JA, Cunha A, Almeida A. 2009. Charge effect on the photoinactivation of Gram-negative and Gram-positive bacteria by cationic meso-substituted porphyrins. BMC Microbiol. 9:70.
- Alves E, Faustino MA, Neves MG, Cunha A, Tome J, Almeida A. 2014. An insight on bacterial cellular targets of photodynamic inactivation. Future Med Chem. 6:141–164.
- Alves F, Alonso GC, Carmello JC, de Oliveira Mima EG, Bagnato VS, Pavarina AC. 2017. Antimicrobial photodynamic therapy mediated by Photodithazine® in the treatment of denture stomatitis: a case report. Photodiagnosis Photodyn Ther. 21:168–171.
- Ambriz-Aviña V, Contreras-Garduño JA, Pedraza-Reyes M. 2014. Applications of flow cytometry to characterize bacterial physiological responses. BioMed Res Int. 2014:461941.
- Aminov RI. 2010. A brief history of the antibiotic era: lessons learned and challenges for the future. Front Microbiol. 1:134.
- Araújo NC, Fontana CR, Bagnato VS, Gerbi MEM. 2014. Photodynamic antimicrobial therapy of curcumin in biofilms and carious dentine. Lasers Med Sci. 29:629–635.
- Arweiler NB, Pietruska M, Pietruski J, Skurska A, Dolińska E, Heumann C, Auschill TM, Sculean A. 2014. Six-month results following treatment of aggressive periodontitis with antimicrobial photodynamic therapy or amoxicillin and metronidazole. Clin Oral Investig. 18:2129–2135.
- Baier J, Maier M, Engl R, Landthaler M, Bäumler W. 2005. Time-resolved investigations of singlet oxygen luminescence in water, in phosphatidylcholine, and in aqueous suspensions of phosphatidylcholine or HT29 cells. J Phys Chem B. 109:3041–3046.
- Baltazar LM, Ray A, Santos DA, Cisalpino PS, Friedman AJ, Nosanchuk JD. 2015. Antimicrobial photodynamic therapy: an effective alternative approach to control fungal infections. Front Microbiol. 6:202.
- Baptista MS, Cadet J, Di Mascio P, Ghogare AA, Greer A, Hamblin MR, Lorente C, Nunez SC, Ribeiro MS, Thomas AH, et al. 2017. Type I and type II photosensitized oxidation reactions: guidelines and mechanistic pathways. Photochem Photobiol. 93:912–919.
- Belibasakis GN, Thurnheer T. 2014. Validation of antibiotic efficacy on in vitro subgingival biofilms. J Periodontol. 85:343–348.
- Boles BR, Thoendel M, Singh PK. 2004. Self-generated diversity produces “insurance effects” in biofilm communities. Proc Natl Acad Sci USA. 101:16630–16635.
- Borsatto MC, Correa-Afonso AM, Lucisano MP, Bezerra da Silva RA, Paula-Silva FWG, Nelson-Filho P, Bezerra da Silva LA. 2016. One-session root canal treatment with antimicrobial photodynamic therapy (aPDT): an in vivo study. Int Endod J. 49:511–518.
- Boyce JM, Pittet D. 2002. Guideline for hand hygiene in health-care settings: Recommendations of the healthcare infection control practices advisory committee and the HICPAC/SHEA/APIC/IDSA hand hygiene task force. Infect Control Hosp Epidemiol. 23:3–40.
- Brown S. 2012. Clinical antimicrobial photodynamic therapy: phase II studies in chronic wounds. J Natl Compr Canc Netw. 10 Suppl 2:S80–S83.
- Burmølle M, Webb JS, Rao D, Hansen LH, Sørensen SJ, Kjelleberg S. 2006. Enhanced biofilm formation and increased resistance to antimicrobial agents and bacterial invasion are caused by synergistic interactions in multispecies biofilms. Appl Environ Microbiol. 72:3916–3923.
- Busscher HJ, van der Mei HC, Subbiahdoss G, Jutte PC, van den Dungen JJAM Zaat SAJ, Schultz MJ, Grainger DW. 2012. Biomaterial-associated infection: locating the finish line in the race for the surface. Sci Transl Med. 4:153rv10.
- Cadet J, Douki T, Ravanat J-L. 2010. Oxidatively generated base damage to cellular DNA. Free Radic Biol Med. 49:9–21.
- Caro H. 1878. Improvement in the production of dye-stuffs from methyl-aniline. Patent No. 204,796. Mannheim: US Patent Office.
- Cassidy CM, Donnelly RF, Tunney MM. 2010. Effect of sub-lethal challenge with Photodynamic Antimicrobial Chemotherapy (PACT) on the antibiotic susceptibility of clinical bacterial isolates. J Photochem Photobiol B Biol. 99:62–66.
- Chambless JD, Hunt SM, Stewart PS. 2006. A three-dimensional computer model of four hypothetical mechanisms protecting biofilms from antimicrobials. Appl Environ Microbiol. 72:2005–2013.
- Choy MH, Stapleton F, Willcox MDP, Zhu H. 2008. Comparison of virulence factors in Pseudomonas aeruginosa strains isolated from contact lens- and non-contact lens-related keratitis. J Med Microbiol. 57:1539–1546.
- Chrepa V, Kotsakis GA, Pagonis TC, Hargreaves KM. 2014. The effect of photodynamic therapy in root canal disinfection: a systematic review. J Endod. 40:891–898.
- Cieplik F, Buchalla W, Hellwig E, Al-Ahmad A, Hiller K-A, Maisch T, Karygianni L. 2017. Antimicrobial photodynamic therapy as an adjunct for treatment of deep carious lesions-A systematic review. Photodiagnosis Photodyn Ther. 18:54–62.
- Cieplik F, Pummer A, Leibl C, Regensburger J, Schmalz G, Buchalla W, Hiller K-A, Maisch T. 2016. Photodynamic Inactivation of root canal bacteria by light activation through human dental hard and simulated surrounding tissue. Front Microbiol. 7:929.
- Cieplik F, Pummer A, Regensburger J, Hiller K-A, Späth A, Tabenski L, Buchalla W, Maisch T. 2015. The impact of absorbed photons on antimicrobial photodynamic efficacy. Front Microbiol. 6:706.
- Cieplik F, Späth A, Leibl C, Gollmer A, Regensburger J, Tabenski L, Hiller K-A, Maisch T, Schmalz G. 2014. Blue light kills Aggregatibacter actinomycetemcomitans due to its endogenous photosensitizers. Clin Oral Investig. 18:1763–1769.
- Cieplik F, Späth A, Regensburger J, Gollmer A, Tabenski L, Hiller K-A, Bäumler W, Maisch T, Schmalz G. 2013. Photodynamic biofilm inactivation by SAPYR-an exclusive singlet oxygen photosensitizer. Free Radic Biol Med. 65:477–487.
- Cieplik F, Steinwachs V-S, Muehler D, Hiller K-A, Thurnheer T, Belibasakis GN, Buchalla W, Maisch T. 2018. Phenalen-1-one mediated antimicrobial photodynamic therapy: antimicrobial efficacy in a periodontal biofilm model and flow cytometric evaluation of cytoplasmic membrane damage. Front Microbiol. 9:688.
- Cieplik F, Tabenski L, Buchalla W, Maisch T. 2014. Antimicrobial photodynamic therapy for inactivation of biofilms formed by oral key pathogens. Front Microbiol. 5:405.
- Cieplik F, Wimmer F, Muehler D, Thurnheer T, Belibasakis GN, Hiller K-A, Maisch T, Buchalla W. 2018. Phenalen-1-one-mediated antimicrobial photodynamic therapy and chlorhexidine applied to a novel caries biofilm model. Caries Res. 52:447–453.
- Collins TL, Markus EA, Hassett DJ, Robinson JB. 2010. The effect of a cationic porphyrin on Pseudomonas aeruginosa biofilms. Curr Microbiol. 61:411–416.
- Czaplewski L, Bax R, Clokie M, Dawson M, Fairhead H, Fischetti VA, Foster S, Gilmore BF, Hancock REW, Harper D, et al. 2016. Alternatives to antibiotics-a pipeline portfolio review. Lancet Infect Dis. 16:239–251.
- Davey ME, O’Toole GA. 2000. Microbial biofilms: from ecology to molecular genetics. Microbiol Mol Biol Rev. 64:847–867.
- de Aguiar Coletti TMSF, de Freitas LM, Almeida AMF, Fontana CR. 2017. Optimization of antimicrobial photodynamic therapy in biofilms by inhibiting efflux pump. Photomed Laser Surg. 35:378–385.
- de Miranda RG, Colombo APV. 2018. Clinical and microbiological effectiveness of photodynamic therapy on primary endodontic infections: a 6-month randomized clinical trial. Clin Oral Investig. 22:1751–1761.
- de Oliveira RR, Novaes AB, Garlet GP, de Souza RF, Taba M, Sato S, de Souza SLS, Palioto DB, Grisi MFM, Feres M. 2010. The effect of a single episode of antimicrobial photodynamic therapy in the treatment of experimental periodontitis. Microbiological profile and cytokine pattern in the dog mandible. Lasers Med Sci. 26:359–367.
- Demidova TN, Gad F, Zahra T, Francis KP, Hamblin MR. 2005. Monitoring photodynamic therapy of localized infections by bioluminescence imaging of genetically engineered bacteria. J Photochem Photobiol B Biol. 81:15–25.
- DeRosa M. 2002. Photosensitized singlet oxygen and its applications. Coord Chem Rev. 233-234:351–371.
- Dovigo LN, Carmello JC, Carvalho MT, Mima EG, Vergani CE, Bagnato VS, Pavarina AC. 2013. Photodynamic inactivation of clinical isolates of Candida using Photodithazine®. Biofouling. 29:1057–1067.
- Engel E, Schraml R, Maisch T, Kobuch K, König B, Szeimies R-M, Hillenkamp J, Bäumler W, Vasold R. 2008. Light-induced decomposition of indocyanine green. Invest Ophthalmol Vis Sci. 49:1777–1783.
- Exterkate RAM, Crielaard W, Cate ten JM. 2010. Different response to amine fluoride by Streptococcus mutans and polymicrobial biofilms in a novel high-throughput active attachment model. Caries Res. 44:372–379.
- Felbert von V, Hoffmann G, Hoff-Lesch S, Abuzahra F, Renn CN, Braathen LR, Merk HF. 2010. Photodynamic therapy of multiple actinic keratoses: reduced pain through use of visible light plus water-filtered infrared A compared with light from light-emitting diodes. Br J Dermatol. 163:607–615.
- Felgenträger A, Maisch T, Dobler D, Späth A. 2013. Hydrogen bond acceptors and additional cationic charges in methylene blue derivatives: photophysics and antimicrobial efficiency. BioMed Res Int. 2013:482167.
- Fernandez JM, Bilgin MD, Grossweiner LI. 1997. Singlet oxygen generation by photodynamic agents. J Photochem Photobiol B Biol. 37:131–140.
- Fernandez Y, Mostajo M, Exterkate RAM, Buijs MJ, Beertsen W, van der Weijden GA, Zaura E, Crielaard W. 2017. A reproducible microcosm biofilm model of subgingival microbial communities. J Periodont Res. 52:1021–1031.
- Flors C, Nonell S. 2006. Light and singlet oxygen in plant defense against pathogens: phototoxic phenalenone phytoalexins. Acc Chem Res. 39:293–300.
- Fuchs SM, Fluhr JW, Bankova L, Tittelbach J, Hoffmann G, Elsner P. 2004. Photodynamic therapy (PDT) and waterfiltered infrared A (wIRA) in patients with recalcitrant common hand and foot warts. Ger Med Sci. 2:Doc08.
- Garcez AS, Núñez SC, Hamblin MR, Suzuki H, Ribeiro MS. 2010. Photodynamic therapy associated with conventional endodontic treatment in patients with antibiotic-resistant microflora: a preliminary report. J Endod. 36:1463–1466.
- Gerschman R, Gilbert DL, Nye SW, Dwyer P, Fenn WO. 1954. Oxygen poisoning and x-irradiation: a mechanism in common. Science. 119:623–626.
- Giuliani F, Martinelli M, Cocchi A, Arbia D, Fantetti L, Roncucci G. 2010. In vitro resistance selection studies of RLP068/Cl, a new Zn(II) phthalocyanine suitable for antimicrobial photodynamic therapy. Antimicrob Agents Chemother. 54:637–642.
- Gollmer A, Felgentraeger A, Maisch T, Flors C. 2017. Real-time imaging of photodynamic action in bacteria. J Biophotonics. 10:264–270.
- Gollmer A, Felgenträger A, Bäumler W, Maisch T, Späth A. 2015. A novel set of symmetric methylene blue derivatives exhibits effective bacteria photokilling – a structure-response study. Photochem Photobiol Sci. 14:335–351.
- Goulart R de C, Bolean M, Paulino T de P, Thedei G, Souza SLS, Tedesco AC, Ciancaglini P. 2010. Photodynamic therapy in planktonic and biofilm cultures of Aggregatibacter actinomycetemcomitans. Photomed Laser Surg. 28(Suppl 1):S53–S60.
- Gouterman M. 1961. Spectra of porphyrins. J Mol Spectrosc. 6:138–163.
- Guggenheim B, Giertsen E, Schüpbach P, Shapiro S. 2001. Validation of an in vitro biofilm model of supragingival plaque. J Dent Res. 80:363–370.
- Gursoy H, Ozcakir-Tomruk C, Tanalp J, Yilmaz S. 2013. Photodynamic therapy in dentistry: a literature review. Clin Oral Investig. 17:1113–1125.
- Haase M, Schäfer H. 2011. Upconverting nanoparticles. Angew Chem Int Ed Engl. 50:5808–5829.
- Hamblin MR, Hasan T. 2004. Photodynamic therapy: A new antimicrobial approach to infectious disease? Photochem Photobiol Sci. 3:436–450.
- Hamblin MR, O’Donnell DA, Murthy N, Contag CH, Hasan T. 2002. Rapid control of wound infections by targeted photodynamic therapy monitored by in vivo bioluminescence imaging. Photochem Photobiol. 75:51–57.
- Hamblin MR. 2017. Potentiation of antimicrobial photodynamic inactivation by inorganic salts. Expert Rev Anti Infect Ther. 15:1059–1069.
- Hamblin MR. 2018. Upconversion in photodynamic therapy: plumbing the depths. Dalton Trans. [in press; DOI: 10.1039/c8dt00087e]
- Hartel M, Hoffmann G, Wente MN, Martignoni ME, Büchler MW, Friess H. 2006. Randomized clinical trial of the influence of local water‐filtered infrared A irradiation on wound healing after abdominal surgery. BJS. 93:952–960.
- Hoffmann G, Hartel M, Mercer JB. 2016. Heat for wounds – water-filtered infrared-A (wIRA) for wound healing – a review. Ger Med Sci. 14:Doc08.
- Holmes AH, Moore LSP, Sundsfjord A, Steinbakk M, Regmi S, Karkey A, Guerin PJ, Piddock LJV. 2016. Understanding the mechanisms and drivers of antimicrobial resistance. Lancet. 387:176–187.
- Horner C, Mawer D, Wilcox M. 2012. Reduced susceptibility to chlorhexidine in staphylococci: is it increasing and does it matter? J Antimicrob Chemother. 67:2547–2559.
- Huang L, Szewczyk G, Sarna T, Hamblin MR. 2017. Potassium iodide potentiates broad-spectrum antimicrobial photodynamic inactivation using photofrin. ACS Infect Dis. 3:320–328.
- Huber H. 1905. Weitere Versuche mit photodynamischen, sensibilisierenden Farbstoffen (Eosin, Erythrosin). Prüfung der Wirkung des Tageslichtes auf Lebensfähigkeit und Virulenz von Bakterien, auf Toxine und Antitoxine und auf das Labferment. Archiv Hyg. 54:53–88.
- Imlay JA. 2013. The molecular mechanisms and physiological consequences of oxidative stress: lessons from a model bacterium. Nat Rev Microbiol. 11:443–454.
- Javed F, Samaranayake LP, Romanos GE. 2014. Treatment of oral fungal infections using antimicrobial photodynamic therapy: a systematic review of currently available evidence. Photochem Photobiol Sci. 13:726–734.
- Jesionek A, Tappeiner von H. 1905. Zur Behandlung der Hautcarcinome mit fluoreszierenden Stoffen. Dtsch Arch Klin Med. 85:223–239.
- Jodlbauer A, Tappeiner von H. 1904. Über die Wirkung photodynamischer (fluoreszierender) Stoffe auf Bakterien. Münch Med Wochenschr. 51:1096–1097.
- Joux F, Lebaron P. 2000. Use of fluorescent probes to assess physiological functions of bacteria at single-cell level. Microbes Infect. 2:1523–1535.
- Jung T, Grune T. 2012. Experimental basis for discriminating between thermal and athermal effects of water-filtered infrared A irradiation. Ann N Y Acad Sci. 1259:33–38.
- Kampf G. 2016. Acquired resistance to chlorhexidine – is it time to establish an “antiseptic stewardship” initiative? J Hosp Infect. 94:213–227.
- Karygianni L, Ruf S, Follo M, Hellwig E, Bucher M, Anderson AC, Vach K, Al-Ahmad A. 2014. Novel broad-spectrum antimicrobial photoinactivation of in situ oral biofilms by visible light plus water-filtered infrared A. Appl Environ Microbiol. 80:7324–7336.
- Kashef N, Hamblin MR. 2017. Can microbial cells develop resistance to oxidative stress in antimicrobial photodynamic inactivation? Drug Resist Updat. 31:31–42.
- Kiesslich T, Gollmer A, Maisch T, Berneburg M, Plaetzer K. 2013. A comprehensive tutorial on in vitro characterization of new photosensitizers for photodynamic antitumor therapy and photodynamic inactivation of microorganisms. BioMed Res Int. 2013:840417.
- Kishen A, Upadya M, Tegos GP, Hamblin MR. 2010. Efflux pump inhibitor potentiates antimicrobial photodynamic inactivation of Enterococcus faecalis biofilm. Photochem Photobiol. 86:1343–1349.
- Krieger-Liszkay A. 2005. Singlet oxygen production in photosynthesis. J Exp Bot. 56:337–346.
- Lauro FM, Pretto P, Covolo L, Jori G, Bertoloni G. 2002. Photoinactivation of bacterial strains involved in periodontal diseases sensitized by porphycene–polylysine conjugates. Photochem Photobiol Sci. 1:468–470.
- Laxminarayan R, Matsoso P, Pant S, Brower C, Røttingen J-A, Klugman K, Davies S. 2016. Access to effective antimicrobials: a worldwide challenge. Lancet. 387:168–175.
- Lei X, Liu B, Huang Z, Wu J. 2015. A clinical study of photodynamic therapy for chronic skin ulcers in lower limbs infected with Pseudomonas aeruginosa. Arch Dermatol Res. 307:49–55.
- Lewis K. 2001. Riddle of biofilm resistance. Antimicrob Agents Chemother. 45:999–1007.
- Lewis K. 2012. Antibiotics: recover the lost art of drug discovery. Nature. 485:439–440.
- López-Muñoz F, Alamo C, Cuenca E, Shen WW, Clervoy P, Rubio G. 2005. History of the discovery and clinical introduction of chlorpromazine. Ann Clin Psychiatry. 17:113–135.
- Lyon JP, Moreira LM, de Moraes PCG, Santos dos FV, de Resende MA. 2011. Photodynamic therapy for pathogenic fungi. Mycoses. 54:e265–e271.
- Madsen JS, Burmølle M, Hansen LH, Sørensen SJ. 2012. The interconnection between biofilm formation and horizontal gene transfer. FEMS Immunol Med Microbiol. 65:183–195.
- Mah T-FC, O'Toole GA. 2001. Mechanisms of biofilm resistance to antimicrobial agents. Trends Microbiol. 9:34–39.
- Maillard JY. 2002. Bacterial target sites for biocide action. J Appl Microbiol. 92:16S–27S.
- Maisch T, Baier J, Franz B, Maier M, Landthaler M, Szeimies R-M, Bäumler W. 2007. The role of singlet oxygen and oxygen concentration in photodynamic inactivation of bacteria. Proc Natl Acad Sci USA. 104:7223–7228.
- Maisch T, Bosl C, Szeimies R-M, Lehn N, Abels C. 2005. Photodynamic effects of novel XF porphyrin derivatives on prokaryotic and eukaryotic cells. Antimicrob Agents Chemother. 49:1542–1552.
- Maisch T, Eichner A, Späth A, Gollmer A, König B, Regensburger J, Bäumler W. 2014. Fast and effective photodynamic inactivation of multiresistant bacteria by cationic riboflavin derivatives. PLoS One. 9:e111792.
- Maisch T, Szeimies R-M, Jori G, Abels C. 2004. Antibacterial photodynamic therapy in dermatology. Photochem Photobiol Sci. 3:907–917.
- Maisch T. 2015a. Resistance in antimicrobial photodynamic inactivation of bacteria. Photochem Photobiol Sci. 14:1518–1526.
- Maisch T. 2015b. Strategies to optimize photosensitizers for photodynamic inactivation of bacteria. J Photochem Photobiol B, Biol. 150:2–10.
- Makdoumi K, Bäckman A. 2016. Photodynamic UVA-riboflavin bacterial elimination in antibiotic-resistant bacteria. Clin Experiment Ophthalmol. 44:582–586.
- Mang T, Rogers S, Keinan D, Honma K, Baier R. 2016. Antimicrobial photodynamic therapy (aPDT) induction of biofilm matrix architectural and bioadhesive modifications. Photodiagnosis Photodyn Ther. 13:22–28.
- Marsh PD. 2004. Dental plaque as a microbial biofilm. Caries Res. 38:204–211.
- Marsh PD, Moter A, Devine DA. 2011. Dental plaque biofilms: communities, conflict and control. Periodontol 2000. 55:16–35.
- McCarthy NL, Sullivan PS, Gaynes R, Rimland D. 2010. Health care-associated and community-associated methicillin-resistant Staphylococcus aureus infections: a comparison of definitions. Am J Infect Control. 38:600–606.
- McDonnell G, Russell AD. 1999. Antiseptics and disinfectants: activity, action, and resistance. Clin Microbiol Rev. 12:147–179.
- Mercer JB, Nielsen SP, Hoffmann G. 2008. Improvement of wound healing by water-filtered infrared-A (wIRA) in patients with chronic venous stasis ulcers of the lower legs including evaluation using infrared thermography. Ger Med Sci. 6:Doc11.
- Meyer B, Cookson B. 2010. Does microbial resistance or adaptation to biocides create a hazard in infection prevention and control? J Hosp Infect. 76:200–205.
- Miller RA, Britigan BE. 1997. Role of oxidants in microbial pathophysiology. Clin Microbiol Rev. 10:1–18.
- Mima EG, Vergani CE, Machado AL, Massucato EMS, Colombo AL, Bagnato VS, Pavarina AC. 2012. Comparison of photodynamic therapy versus conventional antifungal therapy for the treatment of denture stomatitis: a randomized clinical trial. Clin Microbiol Infect. 18:E380–E388.
- Mizuno K, Zhiyentayev T, Huang L, Khalil S, Nasim F, Tegos GP, Gali H, Jahnke A, Wharton T, Hamblin MR. 2011. Antimicrobial photodynamic therapy with functionalized Fullerenes: quantitative structure-activity relationships. J Nanomed Nanotechnol. 2:1–9.
- Morgan DJ, Okeke IN, Laxminarayan R, Perencevich EN, Weisenberg S. 2011. Non-prescription antimicrobial use worldwide: a systematic review. Lancet Infect Dis. 11:692–701.
- Morley S, Griffiths J, Philips G, Moseley H, O'Grady C, Mellish K, Lankester CL, Faris B, Young RJ, Brown SB, et al. 2013. Phase IIa randomized, placebo-controlled study of antimicrobial photodynamic therapy in bacterially colonized, chronic leg ulcers and diabetic foot ulcers: a new approach to antimicrobial therapy. Br J Dermatol. 168:617–624.
- Mroz P, Tegos GP, Gali H, Wharton T, Sarna T, Hamblin MR. 2007. Photodynamic therapy with fullerenes. Photochem Photobiol Sci. 6:1139–1149.
- Muehler D, Sommer K, Wennige S, Hiller K-A, Cieplik F, Maisch T, Späth A. 2017. Light-activated phenalen-1-one bactericides: efficacy, toxicity and mechanism compared with benzalkonium chloride. Future Microbiol. 12:1297–1310.
- Nagata JY, Hioka N, Kimura E, Batistela VR, Terada RSS, Graciano AX, Baesso ML, Hayacibara MF. 2012. Antibacterial photodynamic therapy for dental caries: evaluation of the photosensitizers used and light source properties. Photodiagnosis Photodyn Ther. 9:122–131.
- Nakonieczna J, Michta E, Rybicka M, Grinholc M, Gwizdek-Wiśniewska A, Bielawski KP. 2010. Superoxide dismutase is upregulated in Staphylococcus aureus following protoporphyrin-mediated photodynamic inactivation and does not directly influence the response to photodynamic treatment. BMC Microbiol. 10:323.
- Nammour S, Zeinoun T, Bogaerts I, Lamy M, Geerts SO, Bou Saba S, Lamard L, Peremans A, Limme M. 2010. Evaluation of dental pulp temperature rise during photo-activated decontamination (PAD) of caries: an in vitro study. Lasers Med Sci. 25:651–654.
- Nebe-von Caron G, Stephens P, Badley RA. 1998. Assessment of bacterial viability status by flow cytometry and single cell sorting. J Appl Microbiol. 84:988–998.
- Nitzan Y, Ashkenazi H. 1999. Photoinactivation of Deinococcus radiodurans: an unusual Gram‐positive microorganism. Photochem Photobiol. 69:505–510.
- Nonell S, Gonzalez M, Trull FR. 1993. 1H-Phenalen-1-one-2-sulfonic acid: an extremely efficient singlet molecular oxygen sensitizer for aqueous media. Afinidad. 50:445–450.
- Novo DJ, Perlmutter NG, Hunt RH, Shapiro HM. 2000. Multiparameter flow cytometric analysis of antibiotic effects on membrane potential, membrane permeability, and bacterial counts of Staphylococcus aureus and Micrococcus luteus. Antimicrob Agents Chemother. 44:827–834.
- Núñez SC, Yoshimura TM, Ribeiro MS, Junqueira HC, Maciel C, Coutinho-Neto MD, Baptista MS. 2015. Urea enhances the photodynamic efficiency of methylene blue. J Photochem Photobiol B, Biol. 150:31–37.
- Pedigo LA, Gibbs AJ, Scott RJ, Street CN. 2009. Absence of bacterial resistance following repeat exposure to photodynamic therapy. In: Proc. SPIE 7380, Photodynamic Therapy: Back to the Future, article 73803H.
- Perlin DS, Rautemaa-Richardson R, Alastruey-Izquierdo A. 2017. The global problem of antifungal resistance: Prevalence, mechanisms, and management. Lancet Infect Dis. 17:e383–e392.
- Piazena H, Meffert H, Uebelhack R. 2017. Spectral remittance and transmittance of visible and infrared-A radiation in human skin-comparison between in vivo measurements and model calculations. Photochem Photobiol. 93:1449–1461.
- Pinton PC, Rossi MT, Sala R, Venturini M. 2012. Photodynamic antifungal chemotherapy. Photochem Photobiol. 88:512–522.
- Pomposiello PJ, Demple B. 2002. Global adjustment of microbial physiology during free radical stress. Adv Microb Physiol. 46:319–341.
- Prates RA, Kato IT, Ribeiro MS, Tegos GP, Hamblin MR. 2011. Influence of multidrug efflux systems on methylene blue-mediated photodynamic inactivation of Candida albicans. J Antimicrob Chemother. 66:1525–1532.
- Preus HR, Scheie AA, Baelum V. 2014. Letter to the editor: Re: The clinical effect of scaling and root planing and the concomitant administration of systemic amoxicillin and metronidazole: a systematic review; Re: Effectiveness of systemic amoxicillin/metronidazole as adjunctive therapy to scaling and root planing in the treatment of chronic periodontitis: a systematic review and meta-analysis; Re: Effectiveness of systemic amoxicillin/metronidazole as an adjunctive therapy to full-mouth scaling and root planing in the treatment of aggressive periodontitis: a systematic review and meta-analysis. J Periodontol. 85:374–384.
- Preuß A, Zeugner L, Hackbarth S, Faustino MAF, Neves MGPMS, Cavaleiro JAS, Roeder B. 2013. Photoinactivation of Escherichia coli (SURE2) without intracellular uptake of the photosensitizer. J Appl Microbiol. 114:36–43.
- Pummer A, Knüttel H, Hiller K-A, Buchalla W, Cieplik F, Maisch T. 2017. Antimicrobial efficacy of irradiation with visible light on oral bacteria in vitro: a systematic review. Future Med Chem. 9:1557–1574.
- Raab O. 1900. Über die Wirkung fluorescierender Stoffe auf Infusoria. Z Biol. 39:524.
- Ragàs X, Agut M, Nonell S. 2010. Singlet oxygen in Escherichia coli: new insights for antimicrobial photodynamic therapy. Free Radic Biol Med. 49:770–776.
- Ramel F, Birtic S, Cuiné S, Triantaphylidès C, Ravanat J-L, Havaux M. 2012. Chemical quenching of singlet oxygen by carotenoids in plants. Plant Physiol. 158:1267–1278.
- Regensburger J, Knak A, Maisch T, Landthaler M, Bäumler W. 2012. Fatty acids and vitamins generate singlet oxygen under UVB irradiation. Exp Dermatol. 21:135–139.
- Renwick MJ, Simpkin V, Mossialos E. 2016. Targeting innovation in antibiotic drug discovery and development: the need for a One Health – One Europe – One World Framework. Copenhagen (Denmark): European Observatory on Health Systems and Policies. Health Policy Series, No. 45. ISBN-13: 9789289050401.
- Ribeiro APD, Andrade MC, Bagnato VS, Vergani CE, Primo FL, Tedesco AC, Pavarina AC. 2015. Antimicrobial photodynamic therapy against pathogenic bacterial suspensions and biofilms using chloro-aluminum phthalocyanine encapsulated in nanoemulsions. Lasers Med Sci. 30:549–559.
- Rineh A, Bremner JB, Hamblin MR, Ball AR, Tegos GP, Kelso MJ. 2018. Attaching NorA efflux pump inhibitors to methylene blue enhances antimicrobial photodynamic inactivation of Escherichia coli and Acinetobacter baumannii in vitro and in vivo. Bioorg Med Chem Lett. [in press; DOI: 10.1016/j.bmcl.2018.02.041]
- Rineh A, Dolla NK, Ball AR, Magana M, Bremner JB, Hamblin MR, Tegos GP, Kelso MJ. 2017. Attaching the NorA efflux pump inhibitor INF55 to methylene blue enhances antimicrobial photodynamic inactivation of methicillin-resistant Staphylococcus aureus in vitro and in vivo. ACS Infect Dis. 3:756–766.
- Schäfer M, Schmitz C, Horneck G. 1998. High sensitivity of Deinococcus radiodurans to photodynamically-produced singlet oxygen. Int J Radiat Biol. 74:249–253.
- Schumann H, Calow T, Weckesser S, Müller ML, Hoffmann G. 2011. Water-filtered infrared A for the treatment of chronic venous stasis ulcers of the lower legs at home: a randomized controlled blinded study. Br J Dermatol. 165:541–551.
- Sculean A, Aoki A, Romanos G, Schwarz F, Miron RJ, Cosgarea R. 2015. Is photodynamic therapy an effective treatment for periodontal and peri-implant infections? Dent Clin North Am. 59:831–858.
- Seneviratne G, Zavahir JS, Bandara WMMS, Weerasekara MLMAW. 2007. Fungal-bacterial biofilms: their development for novel biotechnological applications. World J Microbiol Biotechnol. 24:739–743.
- Sgolastra F, Petrucci A, Severino M, Graziani F, Gatto R, Monaco A. 2013. Adjunctive photodynamic therapy to non-surgical treatment of chronic periodontitis: a systematic review and meta-analysis. J Clin Periodontol. 40:514–526.
- Sharma SK, Chiang LY, Hamblin MR. 2011. Photodynamic therapy with fullerenes in vivo: reality or a dream? Nanomedicine (Lond). 6:1813–1825.
- Silva LAB, Novaes AB, de Oliveira RR, Nelson-Filho P, Santamaria M, Silva RAB. 2012. Antimicrobial photodynamic therapy for the treatment of teeth with apical periodontitis: a histopathological evaluation. J Endod. 38:360–366.
- Sissons CH. 1997. Artificial dental plaque biofilm model systems. Adv Dent Res. 11:110–126.
- Sivaramakrishnan G, Sridharan K. 2018. Photodynamic therapy for the treatment of peri-implant diseases: a network meta-analysis of randomized controlled trials. Photodiagnosis Photodyn Ther. 21:1–9.
- Slots J. 2004. Systemic antibiotics in periodontics. J Periodontol. 75:1553–1565.
- Smith AM, Mancini MC, Nie S. 2009. Bioimaging: second window for in vivo imaging. Nat Nanotechnol. 4:710–711.
- Soukos NS, Goodson JM. 2011. Photodynamic therapy in the control of oral biofilms. Periodontol 2000. 55:143–166.
- Spaeth A, Graeler A, Maisch T, Plaetzer K. 2017. CureCuma–cationic curcuminoids with improved properties and enhanced antimicrobial photodynamic activity. Eur J Med Chem. [in press; DOI: 10.1016/j.ejmech.2017.09.072]
- Späth A, Leibl C, Cieplik F, Lehner K, Regensburger J, Hiller K-A, Bäumler W, Schmalz G, Maisch T. 2014. Improving photodynamic inactivation of bacteria in dentistry: highly effective and fast killing of oral key pathogens with novel tooth-colored type-II photosensitizers. J Med Chem. 57:5157–5168.
- Spranley TJ, Winkler M, Dagate J, Oncale D, Strother E. 2012. Curing light burns. Gen Dent. 60:e210–e214.
- Staerck C, Gastebois A, Vandeputte P, Calenda A, Larcher G, Gillmann L, Papon N, Bouchara J-P, Fleury MJJ. 2017. Microbial antioxidant defense enzymes. Microb Pathog. 110:56–65.
- Stewart PS, Costerton JW. 2001. Antibiotic resistance of bacteria in biofilms. Lancet. 358:135–138.
- Szeimies RM. 2005. Geschichte der Photodynamischen Therapie. Akt Dermatol. 31:193–197.
- Tabenski I, Cieplik F, Tabenski L, Regensburger J, Hiller K-A, Buchalla W, Maisch T, Späth A. 2016. The impact of cationic substituents in phenalen-1-one photosensitizers on antimicrobial photodynamic efficacy. Photochem Photobiol Sci. 15:57–68.
- Tabenski L, Maisch T, Santarelli F, Hiller K-A, Schmalz G. 2014. Individual growth detection of bacterial species in an in vitro oral polymicrobial biofilm model. Arch Microbiol. 196:819–828.
- Tabenski L, Moder D, Cieplik F, Schenke F, Hiller K-A, Buchalla W, Schmalz G, Christgau M. 2016. Antimicrobial photodynamic therapy vs. local minocycline in addition to non-surgical therapy of deep periodontal pockets: a controlled randomized clinical trial. Clin Oral Investig. 21:2253–2264.
- Tappeiner von H. 1909. Die photodynamische Erscheinung (Sensibilisierung durch fluoreszierende Stoffe). Ergebn Physiol. 8:698–741.
- Tardivo JP, Adami F, Correa JA, Pinhal MAS, Baptista MS. 2014. A clinical trial testing the efficacy of PDT in preventing amputation in diabetic patients. Photodiagnosis Photodyn Ther. 11:342–350.
- Tardivo JP, Serrano R, Zimmermann LM, Matos LL, Baptista MS, Pinhal MAS, Atallah ÁN. 2017. Is surgical debridement necessary in the diabetic foot treated with photodynamic therapy? Diabet Foot Ankle. 8:1373552.
- Tavares A, Carvalho CM, Faustino MA, Neves MG, Tomé JP, Tomé AC, Cavaleiro JA, Cunha A, Gomes NCM, Alves E, et al. 2010. Antimicrobial photodynamic therapy: study of bacterial recovery viability and potential development of resistance after treatment. Mar Drugs. 8:91–105.
- Tawakoli PN, Al-Ahmad A, Hoth-Hannig W, Hannig M, Hannig C. 2013. Comparison of different live/dead stainings for detection and quantification of adherent microorganisms in the initial oral biofilm. Clin Oral Investig. 17:841–850.
- Tegos GP, Hamblin MR. 2006. Phenothiazinium antimicrobial photosensitizers are substrates of bacterial multidrug resistance pumps. Antimicrob Agents Chemother. 50:196–203.
- Tegos GP, Masago K, Aziz F, Higginbotham A, Stermitz FR, Hamblin MR. 2008. Inhibitors of bacterial multidrug efflux pumps potentiate antimicrobial photoinactivation. Antimicrob Agents Chemother. 52:3202–3209.
- Tortik N, Spaeth A, Plaetzer K. 2014. Photodynamic decontamination of foodstuff from Staphylococcus aureus based on novel formulations of curcumin. Photochem Photobiol Sci. 13:1402–1409.
- Vecchio D, Gupta A, Huang L, Landi G, Avci P, Rodas A, Hamblin MR. 2015. Bacterial photodynamic inactivation mediated by methylene blue and red light is enhanced by synergistic effect of potassium iodide. Antimicrob Agents Chemother. 59:5203–5212.
- Wainwright M. 1998. Photodynamic antimicrobial chemotherapy (PACT). J Antimicrob Chemother. 42:13–28.
- Wainwright M. 2000. Methylene blue derivatives-suitable photoantimicrobials for blood product disinfection? Int J Antimicrob Agents. 16:381–394.
- Wainwright M. 2017a. Dyes, flies, and sunny skies: photodynamic therapy and neglected tropical diseases. Coloration Technol. 133:3–14.
- Wainwright M. 2017b. The problem with dyes in infection control. Dyes and Pigments. 146:402–407.
- Wainwright M, Maisch T, Nonell S, Plaetzer K, Almeida A, Tegos GP, Hamblin MR. 2017. Photoantimicrobials-are we afraid of the light? Lancet Infect Dis. 17:e49–e55.
- Wainwright M, Phoenix DA, Laycock SL, Wareing DR, Wright PA. 1998. Photobactericidal activity of phenothiazinium dyes against methicillin-resistant strains of Staphylococcus aureus. FEMS Microbiol Lett. 160:177–181.
- Wargo MJ, Hogan DA. 2006. Fungal-bacterial interactions: a mixed bag of mingling microbes. Curr Opin Microbiol. 9:359–364.
- Weerakoon AT, Meyers IA, Symons AL, Walsh LJ. 2002. Pulpal heat changes with newly developed resin photopolymerisation systems. Aust Endod J. 28:108–111.
- Wen X, Zhang X, Szewczyk G, El-Hussein A, Huang Y-Y, Sarna T, Hamblin MR. 2017. Potassium iodide potentiates antimicrobial photodynamic inactivation mediated by Rose Bengal in in vitro and in vivo studies. Antimicrob Agents Chemother. 61:e00467–17.
- Wilkinson F, Helman WP, Ross AB. 1993. Quantum yields for the photosensitized formation of the lowest electronically excited singlet state of molecular oxygen in solution. J Phys Chem Ref Data. 22:113–262.
- Wilson BC, Patterson MS. 2008. The physics, biophysics and technology of photodynamic therapy. Phys Med Biol. 53:R61–109.
- Wolcott R, Costerton JW, Raoult D, Cutler SJ. 2013. The polymicrobial nature of biofilm infection. Clin Microbiol Infect. 19:107–112.
- Wood S, Nattress B, Kirkham J, Shore R, Brookes S, Griffiths J, Robinson C. 1999. An in vitro study of the use of photodynamic therapy for the treatment of natural oral plaque biofilms formed in vivo. J Photochem Photobiol B Biol. 50:1–7.
- Zimmerli W, Trampuz A, Ochsner PE. 2004. Prosthetic-jointinfections. N Engl J Med. 351:1645–1654.