Abstract
Bdellovibrio bacteriovorus is a small Deltaproteobacterium which, since its discovery, has distinguished itself for the unique ability to prey on other Gram-negative bacteria. The studies on this particular “predatory bacterium”, have gained momentum in response to the rising problem of antibiotic resistance, because it could be applied as a potential probiotic and antibiotic agent. Hereby, we present recent advances in the study of B. bacteriovorus, comprehending fundamental aspects of its biology, obligatory intracellular life cycle, predation resistance, and potential applications. Furthermore, we discuss studies that pave the road towards the use of B. bacteriovorus as a “living antibiotic” in human therapy, focussing on its interaction with biofilms, the host immune response, predation susceptibility and in vivo application models. The available data imply that it will be possible to upgrade this predator bacterium from a predominantly academic interest to an instrument that could confront antibiotic resistant infections.
Introduction
Bdellovibrio bacteriovorus was discovered in 1963 by Stolp and Starr (Stolp and Starr Citation1963). While attempting to isolate bacteriophages from a soil sample, they observed unusual lytic plaques (Stolp and Starr Citation1963). Further investigations, uncovered that the growing plaques on the bacterial lawn were not caused by a bacteriophage but by a bacterium itself. The bacterium presented a phage-like life cycle, and its growth was contingent to the presence of a prey. After the discovery of B. bacteriovorus, different research groups have successfully isolated this predatory bacterium from different aquatic and terrestrial sample sites (Chu and Zhu Citation2010; Oyedara et al. Citation2016; Herencias et al. Citation2017). This diversity of sample sites indicates that B. bacteriovorus possesses remarkable ubiquitous capabilities. The predator isolation from different samples was made possible by the double plaque layer method as employed by Stolp and Starr half a century ago (Stolp and Starr Citation1963).
After its discovery, the fundamental characteristics of B. bacteriovorus were investigated and progressively unveiled. B. bacteriovorus takes its name from the Latin word “bdella”, meaning leach-like, and the word “vibrio” that means curved, due to the particular comma shape of this bacterium. This small Deltaproteobacterium is a monotrichous bacterium, with cell dimensions of about 0.3–0.5 µm by 0.5–1.4 µm (Strauch et al. Citation2007). B. bacteriovorus possesses a single sheathed flagellum localised at one of its poles; it also presents a Gram-negative bacterial morphology, with an inner membrane, a peptidoglycan layer and an outer membrane, with the noticeable presence of sphingophospolipids (Burnham et al. Citation1970; Steiner et al. Citation1973). A visualisation of the morphological characteristics of B. bacteriovorus during its lifecycle is presented in and . B. bacteriovorus is characterised by an obligatory intracellular lifestyle. In order to survive and multiply, it must invade the periplasm of other Gram-negative bacteria (Varon and Shilo Citation1969). Upon entry in the prey cell, the predator can then consume the prey’s nutrients, after which point the predator undergoes a septation phase culminating in the lysis of the prey. This reproductive mechanism used by B. bacteriovorus is also termed as Host-Dependent (HD), where the propagation of the predator is contingent on the presence of a suitable prey. Nevertheless, in case of shortage of prey, B. bacteriovorus may also revert to a saprophytic non-virulent state called Host-Independent (HI) (Lambert et al. Citation2010). From a clinical perspective, the HD form of B. bacteriovorus is certainly the most relevant since it has the capability to prey upon a vast range of Gram-negative pathogens (Dashiff et al. Citation2011). Due to the steady increase of antimicrobial resistance (AMR) that has been afflicting human health in the last few decades, particularly in Gram-negative bacteria, interest has risen regarding the investigation of similar predatory bacteria, also known as Bdellovibrio-and-like-organisms (BALO’s) (Snyder et al. Citation2002). For a more extensive understanding of the species belonging to the BALO’s, Pérez et al. provided an excellent overview of the field (Pérez et al. Citation2016). Furthermore, provides an essential overview of the other bacterial species that are known to present a predatory behaviour.
Figure 1. Schematic representation of the life cycle of B. bacteriovorus. Starting clockwise from top left of the image, the predator approaches and binds to the outer surface of its prey. The flagellum is lost and a pore is created. The predator penetrates and settles in the periplasmic space of its host. Subsequently, the pore is sealed and the predator starts consuming the intracellular components of its prey. A septation step follows, culminating in lysis of the host cell and the release of fresh B. bacteriovorus progeny. The new-born predators then start a new predation cycle either through the Host-Dependent cycle or can revert in the Host-Independent state until a suitable prey is encountered.
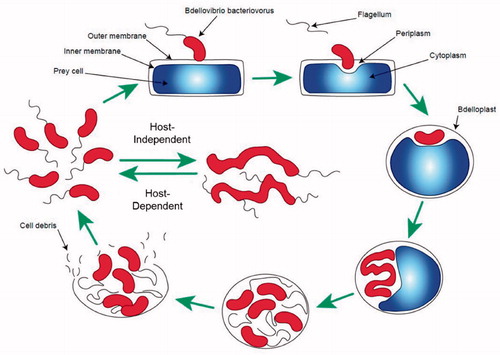
Figure 2. TEM images of various stages of predation. The prey in the images is an Enterobacter roggenkampii isolate. Images I, II and III show B. bacteriovorus HD100 (indicated with arrows) attached to the outer surface of a prey cell or in its immediate surroundings. Image IV shows a late stage of predation where the new-born predators are in the bdelloplast, prior to its disruption (our unpublished data).
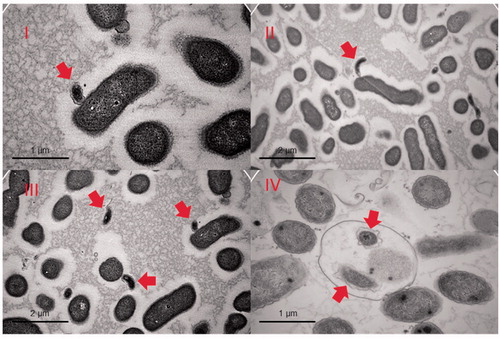
Table 1. Overview of bacteria known to display predatory lifestyles.
Research has revealed the remarkable potential of B. bacteriovorus to kill Gram-negative bacteria belonging to the so-called ESKAPE pathogens, a group currently including some of the most life-threatening human pathogens, such as the Enterobacter genus, Klebsiella pneumoniae, Staphylococcus aureus, Acinetobacter baumannii, Pseudomonas aeruginosa and Escherichia coli (Rice Citation2008; Boucher et al. Citation2009; Bassetti et al. Citation2013). The World Health Organisation has officially drawn the attention of the scientific community towards the current antibiotic crisis, raising awareness of the increased detection frequency of multi-drug resistant (MDR) pathogens throughout the world (Kern Citation2015). The so-called “Golden antibiotic era”, which humanity enjoyed in the second half of the previous century, was characterised by the discovery of nearly all currently known classes of antibiotics in a relatively short period of time (Lyddiard et al. Citation2016). Worth of notice is that among all of the different antibiotics developed and released to the public, none has avoided the insurgence of resistance (Ventola Citation2015; Aslam et al. Citation2018). A variety of factors has contributed to the steady raise of antibiotic resistance through the world, among the most prominent factors: overuse, indiscriminate prescription, extensive use in agriculture, lack of new antibiotics and the ever-growing regulatory criteria for drug development (Ventola Citation2015). Worrisome predictions have been made for the coming decades, suggesting mortality caused by MDR infections could increase from the present levels of ∼700,000 deaths per year to 10 million per year by 2050 (Neill Citation2014).
The reduced efficacy of available antibiotics and the long developing times for novel antibiotics have created a situation in which there is an urgent need for alternative antimicrobial therapies (Mobarki et al. Citation2019). This has led to the revival of old technologies like phage therapy for example, which was firstly developed in Eastern Europe, but was since then overshadowed by the success of Western medicine (Myelnikov Citation2018). Advocates of phage therapy propose that phages could overcome traditional disadvantages of antibiotics, namely specificity, biofilm penetration and toxicity (Lin et al. Citation2017; Kakasis and Panitsa Citation2019). Some of the advantages that phage therapy has traditionally had compared to antibiotics have been: high specificity to their target pathogen, attractive pharmacokinetic properties (i.e. phage propagation as long as the target pathogen is present), low cost of production, and the possibility to be combined in synergy with other treatments. Whilst the critics to the use of phages in therapy have stressed the ineffectiveness against intracellular pathogens, the scarce acceptance among the public, the possibility of transduction of AMR genes and the technology’s reliance on previous identification of the pathogen (Stanczak-Mrozek et al. Citation2017; Melo et al. Citation2020). The use of B. bacteriovorus would serve as an alternative to overcome some limitations of phages. For instance, the predator’s principal advantages on phage therapy could be summarised as: a broad spectre of activity, AMR genes that confer resistance to predation haven’t been reported, high penetration in biofilms, and the apparent absence of specific resistance towards B. bacteriovorus. However, it must also be noted that applications of this predator will have limitations as well. For instance, the inability to eradicate completely the prey population, the inhibitory effect of serum on predation (Im, Son, et al. Citation2017), the inability to attenuate systemic blood infections (Shatzkes et al. Citation2017), the scepticism associated to treating infections with a live bacteria, potential transmission of AMR genes, and non-specific predation that could affect non-pathogenic human commensal bacteria.
Another alternative to conventional antibiotic treatment, would be the use of so-called “amphibiotics” that can act both as antibiotic and probiotic (Dwidar et al. Citation2012). Applications to overcome AMR based on B. bacteriovorus would fall into this alternative category. However, compared to phage therapy, research into possible applications of B. bacteriovorus as antibiotic, probiotic or amphibiotic has received relatively little attention to date. To amend this paucity, the present manuscript highlights the progress achieved in this research field, exhibiting aspects ranging from the biology of this bacterium to the milestones that must be met in order for this fascinating predator to be used in human therapy (Jurkevitch and Jacquet Citation2017; Kowalska and Włodarczyk Citation2017; Negus et al. Citation2017; Popkov et al. Citation2017; Bratanis et al. Citation2020; Laloux Citation2019).
Bdellovibrio characteristics
The optimal growth conditions of B. bacteriovorus have been characterised extensively during the early stages of its discovery. From a culturomic point of view, B. bacteriovorus is an oligotrophic organism that shows a predilection for minimal media. Although regarded as a strictly aerobic bacterium in planktonic conditions, it has been observed that once in intra-periplasmic conditions, the predator was able to survive longer in conditions of oxygen deprivation (Schoeffield et al. Citation1996). Regarding the growth conditions, a pH range of 7.5 − 8.1 and a temperature of 30 °C was found to be optimal for its growth (Seidler and Starr Citation1969).
Similar to other BALO’s, B. bacteriovorus possesses an arsenal of genes through which the bacterium can unfold its predatory nature. The sequenced B. bacteriovorus HD100 strain has a genome of 3.7 Mb (Rendulic et al. Citation2004). Based on bioinformatics analyses of this complete genome sequence, Pasternak et al. defined a core set of genes as the B. bacteriovorus “predatome” (Pasternak et al. Citation2013). In particular, it was inferred that the B. bacteriovorus HD100 genome encodes for 293 lytic proteins, 10 glycanases, 9 RNases, 20 DNases and 15 lipases (Rendulic et al. Citation2004). In addition, this bacterium can employ the mevalonate pathway, a common feature in predatory bacteria, which would allegedly be fuelled by plundering the prey’s (aceto)acetyl-CoA pool. Furthermore, an underrepresented capability to biosynthesize some amino acids also contradistinguishes B. bacteriovorus, which is compensated by an extended capability of synthesising lytic enzymes and transporters. Finally, B. bacteriovorus shows deficits in some metabolic pathways for the synthesis of certain vitamins that need be supplied by the prey and, remarkably, it lacks the known quorum sensing mechanisms (Pasternak et al. Citation2013).
Life cycle
The life cycle of B. bacteriovorus, in its HD state, can be divided into four main phases (Seidler and Starr Citation1969). The predator must first approach and recognise a suitable prey (attack phase) (Stolp and Starr Citation1963), then breach the outer membrane and colonise the prey’s periplasm (invasion phase) (Seidler and Starr Citation1969). Once the breaching has occurred, prey and predator form a characteristic round-shaped structure called bdelloplast (Starr and Baigent Citation1966), which marks the start of the growth phase. Within the bdelloplast the predator grows at the expense of the prey’s constituents. Lastly, upon nutrient depletion, the predator undergoes septation to create new progeny, and finally it bursts the remnants of the host cell to restart its life cycle (septation and lysis phase) (Starr and Baigent Citation1966; Seidler and Starr Citation1969; Fenton et al. Citation2010). shows a schematic representation of the HD life cycle, whilst presents some transmission electron microscopy (TEM) images capturing different stages of predation.
Attack phase
During the initial minutes of the attack phase, the predator reversibly attaches itself to the external membrane of its prey (Stolp and Starr Citation1963; Starr and Baigent Citation1966). Propelled by its flagellum, B. bacteriovorus could reach a velocity of 160 μms-1, which in relative terms constitutes more than 100 times the predator’s own cell length (Lambert et al. Citation2006). Once a collision with a prey has occurred, the predator can either attach to the outer membrane of the prey by means of a rotary movement, or it can detach if it deems the host unsuitable (Starr and Baigent Citation1966; Burnham et al. Citation1968). It has been observed that in cases where the prey on which B. bateriovorus has anchored is somehow unsuitable, the predator detaches from the outer membrane, causing a discontinuity in the cell envelope of the prey (Abram et al. Citation1974). This phenomenon was initially observed by Stolp and Starr and subsequently confirmed by others (Starr and Baigent Citation1966; Evans et al. Citation2007; Mahmoud and Koval Citation2010). The current consent on the mechanisms that B. bacteriovorus uses to attach to its prey is by the mediation of type IV pili, which are small retractile proteinaceous filaments located on the pole opposite to the flagellum (Rendulic et al. Citation2004). The pili appear to be deployed only during the attack phase, specifically during the attachment to the prey, while otherwise residing with the cytoplasmic membrane of the predator (Evans et al. Citation2007). The assembly of type IV pili depends on various factors, such as Tfp, PilF, and PilG, as shown in dedicated studies performed in Neisseria meningitidis, where a role in attachment to human cells mediated by type IV pili was demonstrated (Carbonnelle et al. Citation2005). The role of B. bacteriovorus pili in successful predation has been investigated and many essential components have been identified (Evans et al. Citation2007). It was proven that the presence of the PilA protein is essential for predation to occur, by targeting the protein with an antibody to prove its role in the prey recognition (Evans et al. Citation2007). Additionally, it has been noticed that PilA is strongly expressed, both in the attack phase and in the growth phase, suggesting its involvement in the initial predation processes (Mahmoud and Koval Citation2010). Some authors also advocate the potential role that type IV pili would have in the entry of the predator into the prey, linking the high retraction capability of the pili to the ability to overcome the prey’s cell wall turgor pressure and allowing the predator to squeeze itself through the pore created (Rendulic et al. Citation2004; Evans et al. Citation2007; Borgnia et al. Citation2008; Mahmoud and Koval Citation2010). Nonetheless, in a study conducted by Chanyi et al. two proteins PilT1 and PilT2, which are involved in the retraction of the pilus by hydrolysing ATP, were shown to be neither essential for predation in liquid co-cultures nor for the invasion phase of the prey. Nonetheless PilT2, appears to have a role in successful predation of biofilms (Chanyi and Koval Citation2014).
Invasion phase
The formation of the pore has been considered as a central step to allow the entrance of the predator into the prey. This process is catalysed by a number of enzymatic reactions, as initially proposed by Stolp and Starr (Starr and Baigent Citation1966). Thomashow and Rittenberg, described a model to explain how B. bacteriovorus enters the prey based on glycanases, which would play a major role in the hydrolysis of the prey’s peptidoglycan layer (Thomashow and Rittenberg Citation1978). The investigators advocated a central role of either glycanase or peptidase activities in the formation of the characteristic round shape of the bdelloplast. However, a subsequent study by Tudor et al. affirmed that peptidases were responsible for the peptidoglycan hydrolysis rather than glycanases (Tudor et al. Citation1990). More recently, Lerner et al. discovered that the predator possesses two 4-like penicillin-binding-proteins (PBP) expressed early on in the predation cycle, with DD-carboxy and DD-endopeptidase activities (Lerner et al. Citation2012). These enzymes may contribute also to the round shaping of the bdelloplast, to reduce multiple invasion events by different predators and to catalyse the entry of the whole predator body through the outer membrane of the prey. Nonetheless, the same study also observed that these enzymes are not essential to invasion of the prey. After sequencing the B. bacteriovorus genome, genomic and proteomic studies have further clarified the process of entry and pore formation (Rendulic et al. Citation2004; Dori-Bachash et al. Citation2008). Lambert et al. identified several enzymes involved in the prey entry phase, among which, proteases, glycanases and deacetylases. The study demonstrated a particular role to the deacetylases regarding the weakening of the prey’s peptidoglycan layer, showing that two enzymes deacetylate GlcNAc (Lambert et al. Citation2016). This finding contradicts the earlier studies which asserted that deacetylation of the peptidoglycan would be a mechanism to prevent premature lysis of the bdelloplast by lytic glycanase activity (Lambert et al. Citation2016). Furthermore, the authors suggest that the deacetylation may actually facilitate the initial pore formation catalysed by glycosidase activity rather than hinder it.
Growth, septation and lysis
Immediately after entry into the prey cell, the pore in the prey’s outer membrane is promptly sealed, and the prey cell starts a morphological transition leading to the characteristic round shape of the bdelloplast (Lerner et al. Citation2012). The peptidoglycan layer of the prey is modified by the predator’s transpeptidases in order to make the bdelloplast more resilient to the intracellular osmotic pressure (Kuru et al. Citation2017). Once the entry is completed the predator starts to relentlessly consume all the available nutrients within the bdelloplast in order to replicate itself. A recent study by Bukowska-Faniband et al. demonstrated the involvement of two nucleases Bd0934 and Bd3507, produced by B. bacteriovorus within the bdelloplast especially between 1–4 h, which explains the breakdown of the prey’s nucleic acid within the bdelloplast (Bukowska-Faniband et al. Citation2020). The growth occurs in a filamentous manner from both sides of the poles of the predator (Thomashow and Cotter Citation1992). At this stage of the predator’s growth also its chromosomes are duplicated. It was observed by Makowski et al. that HD B. bacteriovorus replicates its genetic material solely within the bdelloplast (Makowski et al. Citation2019). Apparently, in B. bacteriovorus, chromosome replication is not immediately followed by a division, leading to a transient filamentous structure similar to the one encountered in the replication mechanism of Streptomyces occurs (Ruban-Ośmiałowska et al. Citation2006; Wolanski et al. Citation2011; Makowski et al. Citation2019).
Fenton et al. studied the final stages of B. bacteriovorus growth and reproduction within the prey cell (Fenton et al. Citation2010). The authors observed that a synchronous elongation occurs from both poles of the predator cell and that, once the maximum length is achieved, septation is completed with the spawning of newborn predators (Fenton et al. Citation2010). The septation process is started once the prey’s nutrients are depleted. Both the precise mechanisms behind the chromosome replication and segregation that lead to an odd number of newborn predators remain unknown. The newborn predators then need to lyse the remnants of the depleted prey cell in order to exit the bdelloplast. It has been observed that the time of exit is inversely proportional to the number of progeny present (Fenton et al. Citation2010). Finally, the progeny bursts from the pores, created in the remnants of the prey’s cell and complete their elongation process for a short time outside the host, until they are ready to start a new attack phase (Fenton et al. Citation2010).
Survival in search of prey
The ability of B. bacteriovorus to prey upon other bacteria does not make it invulnerable to environmental threats, nor is it able to indiscriminately prey on all Gram-negative bacteria. Prior to the localisation of a suitable prey, B. bacteriovorus is usually forced to survive in a perilous nutrient-limited environment with potential exposure to chemical and physical insults. A first challenge that B. bacteriovorus needs to overcome in the extracellular environment during the searching phase for a proper prey is to overcome the detrimental effects of secondary metabolites secreted by other organisms. Such secondary metabolites have important ecological and regulatory roles that are crucial in the interaction of B. bacteriovorus with other bacteria (Tyc et al. Citation2017). To date, no specific molecules have been described to specifically target B. bacteriovorus. Yet, some molecules were shown to have inhibiting or toxic effects on the predator’s survival. This is exemplified by cyanides, which were shown to inhibit predation, providing a protective effect for the bacteria that secreted such molecules (Mun et al. Citation2017). Furthermore, certain carbohydrates and pH play a role in predation inhibition, as exemplified by the protective effect of environmental acidification (Dashiff et al. Citation2011).
Predation resistance
Once B. bacteriovorus has successfully survived in its extracellular environment, the subsequent challenge for the predator is posed by the composition of the prey’s capsule and outer membrane layers. Although it has been observed that a wide variety of Gram-negative bacterial species is potentially eligible as prey, yet within the same bacterial species, different levels of susceptibility to predation have been observed (Dashiff et al. Citation2011). Many Gram-negative bacterial species produce a capsule layer, which represents a complex environment populated by different macromolecules that pose a potential challenge for the predator to overcome (Silhavy et al. Citation2010). Further, according to Koval and Hynes, the presence and composition of Gram-negative bacterial S-layers of paracrystalline proteins on the outer membrane would confer a level of protection against predation (Koval and Hynes Citation1991). On the contrary, in a subsequent study it was shown that the capsule of E. coli did not confer protection against B. bacteriovorus predation (Koval and Bayer Citation1997). Furthermore, the presence of a lipo-polysaccharide layer constitutes a barrier to reach the outer membrane and finally the periplasm of a prey. More recently also the O-antigen was investigated as another capsule component that would hinder predation. This was reported based on the higher susceptibility to predation that Vibrio cholerae isolates deficient in the O-antigen presented as compared to their respective wild-type counterparts (Seed et al. Citation2012). Interestingly, from the prey’s perspective, the fitness advantage gained by the increased resistance to B. bacteriovorus predation, comes at an increased susceptibility to bacteriophage attacks caused by the presence of phage receptors in the cell envelope (Seed et al. Citation2012). Altogether, these observations show that the prey of B. bacteriovorus has certain generic tools and strategies to avoid predation, which to date are not yet fully understood. Nevertheless, additional clarity on the essential mechanisms that B. bacteriovorus uses to predate was provided by Duncan et al. by identifying 104 genes involved in predation and additionally proposing a classification system based on the predation deficiency (Duncan et al. Citation2019). A consistently observed feature of predation is the inability to extirpate completely a susceptible prey population, since the surviving fraction manages to mount a momentary protective response, in the form of a transient phenotypic change, termed “plastic response” (Shemesh and Jurkevitch Citation2004). This is a commonly observed ecological mechanism of defense that prey organisms actuate to face threats from protozoa, bacteriophages and predatory bacteria (Hahn and Höfle Citation1999; Bohannan and Lenski Citation2000).
Biofilms
Many clinically relevant bacteria have the ability to produce extracellular polymeric substances (EPS) that create complex association networks, which are generally known as biofilms. The process of biofilm formation is also defined as biofouling which, when compared to planktonic growth, creates a niche microenvironment. The biofilm offers favourable conditions for the resident bacteria (Hall-Stoodley et al. Citation2004; Flemming et al. Citation2016). Major benefits that biofouling confers to the bacteria include shielding against antibiotics, protection against mechanical stress, luring of nutrients due to enhanced sorption, ease of quorum sensing, and colonisation of surfaces (Hall and Mah Citation2017). B. bacteriovorus in its HI state, possesses the ability to form its own biofilms, which would confer to the predator the advantage of being able to survive in environments where suitable prey is lacking (Medina and Kadouri Citation2009).
B. bacteriovorus possesses two characteristics that allow to contrast biofilm formation, namely the ability to effectively penetrate the biofilm’s EPS layer and the potential to kill the biofilm-forming bacteria (Kadouri and O’Toole Citation2005; Núñez et al. Citation2005; Mukherjee et al. Citation2015). The capability of the predator to counter biofilms has been investigated, particularly towards the ESKAPE group. Using the respective pathogens as a possible prey for B. bacteriovorus, Sun et al. showed that the predator does not only abate the prey in its planktonic state, but also reduces established biofilms and even prevents de novo biofilm formation (Sun et al. Citation2017).
Interestingly, Dharani et al. demonstrated the effectiveness of B. bacteriovorus towards colistin resistant mcr-1 mutants of Gram-negative bacteria of the ESKAPE group, some of which were capable of biofilm formation (Dharani et al. Citation2018). Mcr-1 is an enzyme capable of modifying lipid A through the addition of phosphoethanolamine moieties. This results in a change of the overall charge of LPS and, consequently, colistin resistance (Liu et al. Citation2017). Yet, the altered LPS did not preclude predation by B. bacteriovorus, even in a biofilm condition. A noteworthy difference between planktonic and biofilm predation is constituted by the different oxygen requirements of B. bacteriovorus. Oxygen is essential for planktonic predation as demonstrated by Dashiff et al. while the requirement of oxygen appears to be less stringent in cases of biofilm predation (Dashiff and Kadouri Citation2011; Kadouri and Tran Citation2013).
Remarkably, some studies have even shown the ability of B. bacteriovorus to interfere with biofilms formed by Gram-positive bacteria, like Staphylococcus aureus. This challenges the classical “dogma” that B. bacteriovorus predation would be confined only to Gram-negative bacteria (Iebba et al. Citation2014; Im, Dwidar, et al. Citation2018). It has been hypothesised by Pantanella et al. that through this “epibiotic-like” predation on Gram-positive bacterial biofilms, B. bacteriovorus could survive in conditions where Gram-negative bacterial prey is scarce (Pantanella et al. Citation2018). Since a proven effect, both in terms of biofilm reduction and nutrient capture by the predator, at the detriment of S. aureus has been observed, it seems plausible that B. bacteriovorus uses the nutrients from the Gram-positive biofilm to support its own metabolism. This phenomenon would then be enhanced by the production and secretion of proteases that degrade the prey’s proteins for extra provision of peptides and amino acids (Im et al. Citation2018). Furthermore, B. bacteriovorus can sense the presence of Gram-positive bacteria without attacking them, as evidenced by different gene expression profiles of the predator upon exposure to Gram-positive or Gram-negative bacteria (Im et al. Citation2018).
Overall, it can be concluded that B. bacteriovorus has the ability to reduce biofilm formation not only by Gram-negative bacteria, but also by Gram-positive bacteria, in particular S. aureus (Iebba et al. Citation2014; Im et al. Citation2018; Pantanella et al. Citation2018). Nonetheless, the reports that this predator can also interact with Gram-positive bacterial biofilms underscore its potential to contain major pathogens belonging to both bacterial types. This unlocks unique interventional possibilities conferring an edge over other therapeutic options, such as conventional antibiotics or immunisation, by the use of B. bacteriovorus as a “living antibiotic”.
Challenges for Bdellovibrio survival
Competition among living organisms is an unavoidable paradigm in any environment with limited resources. B. bacteriovorus makes no exemption to this archetype of nature. The first recorded image of a tailless icosahedral ssDNA bacteriophage infecting B. bacteriovorus was published in 1970 (Hashimoto et al. Citation1970). Thus opening a new field of research where, progressively, so-called “bdellophages” were discovered and characterised for both the HD and HI variants of B. bacteriovorus (Althauser et al. Citation1972; Varon and Levisohn Citation1972; Roberts et al. Citation1987). Bdellophages were shown to develop in the polar region of the predator’s cytosol, which was captured by electron microscopy images during the infection of an E. coli prey infected by B. bacteriovorus (Kessel and Varon Citation1973). In return the same authors showed that the prey was also infected by bdellophages, forming a so called “three-membered system” (Kessel and Varon Citation1973). Although early studies mention evidence of bdellophages existence, it was only in more recent times that the genomes of bdellophages belonging to the families of Microviridae were sequenced and characterised for the first time (Brentlinger et al. Citation2002; Ackermann et al. Citation2011). The perils for B. bacteriovorus do not only derive from the bacteriophages world, but also from phagotrophic protists that play a relevant role in the composition of bacterial communities. Furthermore, it was shown that ciliated protists are able to feed on both alive and dead B. bacteriovorus (Johnke et al. Citation2017).
Next to biological agents, also environmental and chemical factors can hinder predation by B. bacteriovorus, as reviewed by Mitchell et al. (Mitchell et al. Citation2020). These include the soil percentage in an aqueous solution, the osmolality of the medium used and its viscosity. Additionally, chemical molecules have been identified that are toxic for the predator. For instance, B. bacteriovorus is very sensitive to detergents, such as sodium dodecyl sulphate (SDS) and Triton X-100, which effectively kill the predator while leaving the prey populations unaffected. Thus, SDS kills B. bacteriovorus already at a concentration of 0.02%, demonstrating the effectiveness of such detergents as control agents for B. bacteriovorus (Cho et al. Citation2019). It was also observed that secondary metabolites, such as violacein and cyanide produced by Chromobacterium piscinae, may inhibit the predation process in a Ca/HEPES buffer, though not in diluted nutrient broth (Mun et al. Citation2017). Likewise, components, such as indole in the human gut, have the ability to hinder predation (Dwidar et al. Citation2015). Such physical and chemical factors, along with the afore-mentioned bdellophages and protists may set natural limits to the application of B. bacteriovorus as a biological control agent or antibiotic.
Paving the way towards a “living antibiotic”
Following an initial period of scrutiny characterised by the identification of fundamental aspects regarding the biology of B. bacteriovorus, contemporary investigators have drifted towards applied studies for therapeutic applications of the predator. Based on the in vitro evidence proving the effectiveness of B. bacteriovorus towards human pathogens, a variety of in vivo models has been used to elucidate relevant fundamental aspects and possible concerns. These include the host immune response, toxicity of the predator, effects on the gut microbiota and overall efficacy in vivo. A showcase of some of the principal studies conducted in different animal models regarding B. bacteriovorus and its uses towards in vivo infection models is provided in .
Table 2. Overview of in vivo studies that used B. bacteriovorus as a biocontrol agent against pathogens.
In vivo models and in vitro toxicity studies
The rodent model has been extensively used to characterise the interaction of B. bacteriovorus with a living host. Findlay et al. reported the first successful study proving the capability of B. bacteriovorus to confer protection against a lethal systemic infection caused by Yersinia pestis in SKH-1 mice (Findlay et al. Citation2019). As an additional novelty revealed by this study, it was demonstrated that host adipose tissue acts as a reservoir in which the predator accumulates throughout the duration of the infection. Shatzkes et al. proved the effectiveness of topic intranasal inoculation of B. bacteriovorus, which resulted in a decrease of up to 3.4 log10 CFU/ml of an infection by Enterobacteriaceae in rat lungs (Shatzkes et al. Citation2016). In a subsequent study from the same authors, a systemic injection of B. bacteriovorus was attempted to control a K. pneumoniae infection in a rat model (Shatzkes et al. Citation2017). The host immune response confirmed a low toxicity of the predator, with no rat morbidity or adverse histopathology of different organs due to the administration of the predatory bacteria. An increase in pro-inflammatory cytokines (TNF-α and KC/GRO) was shown, but they returned to baseline levels within 18 h. Efficient clearance of B. bacteriovorus was observed within 20 days. However, the study concluded that the injected B. bacteriovorus was unable to contain the systemic infection, and therefore may not be effective for treatment of acute blood stream infections. The non-toxicity of B. bacteriovorus may be explained by the peculiarly neutral charge of its LPS layer compared to the more negatively-charged LPS present in other Gram-negative bacteria (Schwudke et al. 2003). In contrast with the positive reduction of prey bacteria observed with a peripheral administration of the predator, upon injection of B. bacteriovorus directly into the blood stream the predator appears to lose its ability to reduce the infection caused by the prey. A possible explanation for the struggles of B. bacteriovorus predation in blood stream infection has been proposed by Baker et al. who reported that the complex composition of serum has inhibiting capabilities upon B. bacteriovorus, particularly in the early predation stages (Baker et al. Citation2017). The presence of serum induces the predator to undergo a transient morphological modification involving the rounding of its body and induction of an adaptation period that B. bacteriovorus must overcome before regaining its ability to prey. Nonetheless, further investigations are required to elucidate the exact nature of such inhibition.
The toxicity of the predator in vitro has been characterised using epithelial cells and professional phagocytes. Cell lines exposed to the predator presented a lower inflammatory and endo-toxic response, when compared to the response triggered by the E. coli control bacteria (Gupta et al. Citation2016; Monnappa et al. Citation2016). The in vitro observations on the predator’s toxicity response constituted a starting point for further investigations of the interaction of B. bacteriovorus with the host immune system. Through the use of a zebra fish larval model, it was shown that the predator could work alongside the host immune system to clear lethal infections in vivo. Here B. bacteriovorus displayed both a sufficiently durable persistence in order for predation to occur, and was ultimately cleared by the host phagocytes. In this study, the best effects in terms of infection survival were observed when the synergistic interaction of predator and host was investigated (Willis et al. Citation2016). Upon phagocytosis, a viable persistence of the predator was observed within the phagocytes for 24 h, although in a non-replicative state (Raghunathan et al. Citation2019). Additional in vivo studies were performed to further confirm the low toxicity status of B. bacteriovorus in regard to the gastrointestinal tract and the ocular surface. For instance, a study conducted by Atterbury et al. investigated the effect of ingestion of B. bacteriovorus in a poultry model. This revealed a mild effect of the predator passage on the native gut flora and at the same time the ability to reduce the infection burden caused by an enteric pathogen (Atterbury et al. Citation2011). Concerning B. bacteriovorus’ ability to transit and passage the gastro-intestinal tract, to date there are still contradictory evidences with respect to the predator’s survival, as recently reviewed by Bonfiglio et al. (Bonfiglio et al. Citation2020). Topical administration of B. bacteriovorus on the ocular surface has also been a field investigated by some scientists, considering scenarios of ocular tract infections caused by Gram-negative bacteria (Shanks and Kadouri Citation2014). The precursor study that investigated the potential of B. bacteriovorus as a potential tool to contrast eye infections in vivo, was performed by Nakamura (Nakamura Citation1972). Keratoconjunctivitis (IBK) was prevented through co-infection of Shigella flexneri and the predator. Although the study presented some validity issues, due to the poor behaviour of the controls used, the results obtained managed to highlight the low toxicity of B. bacteriovorus towards the host. Following the original idea of Nakamura, more recently Boileau et al. investigated in an IBK infection the effectiveness of B. bacteriovorus to prey upon the bovine pathogen Mycobacterium bovis (Boileau et al. Citation2011). The authors initially activated the predator towards M. bovis through a series of culture passages spanning a 10-day period, after which it was shown that the predator successfully managed to prevent, within 12 h, the attachment of M. bovis on an epithelial surface. Definite evidence regarding the low toxicity that B. bacteriovorus poses to the cornea epithelium, has been presented recently by Romanowski et al. where both human keratocyte cytotoxicity and in vivo ocular toxicity were assessed for the predator (Romanowski et al. Citation2016). This evidenced a transient production of pro-inflammatory cytokine IL-8, but not of IL-1β. As for the rabbit model used, a lack of toxicity for the ocular epithelial cells was observed with the additional feature of not hindering eventual healing processes of the corneal epithelium.
Combination therapies and potential probiotic application
In natural conditions B. bacteriovorus is part of a complex ecological system characterised by the presence of different species and organisms competing for the same space and resources. This makes the predator subject to certain prey-predator dynamics. The Lotka- Volterra equation defines the equilibrium between prey-predator populations, determining the fluctuation of both groups in regard to each other. According to the model, neither of the two populations could eradicate the other completely (Lotka Citation1920; Volterra Citation1926). The most relevant consequence for B. bacteriovorus to comply with such dynamics is the inability to fully extirpate a prey population (Dwidar et al. Citation2012). One strategy to contrast this natural deficiency of the predator is to couple it with another agent to achieve a more complete annihilation of the targeted pathogen. In order to combine an antibiotic with the predator, a crucial information is the predator’s antibiotic sensitivity. Marine et al. evaluated the antibiotic profile of B. bacteriovorus by developing a liquid co-culture assay composed of the predator and E. coli. The outcomes of the study revealed the antibiogram of B. bacteriovorus towards a range of antibiotics, highlighting the predator’s resistance particularly towards trimethoprim. This is probably due to natural resistance, attributable to the lack of affinity of the predator’s dihydrofolate reductase (Marine et al. Citation2020). One investigation that elucidated the synergistic effect of B. bacteriovorus with an antibiotic was performed by Durán et al. where the inability of the predator to affect Gram-positive bacteria was compensated by the presence of violacein, which is a bisindole antibiotic active towards this class of bacteria (Durán et al. Citation2007). Violacein has been used in combination with B. bacteriovorus HD100 to counter A. baumannii, Bacillus cereus, K. pneumoniae and S. aureus co-cultures. An outstanding antimicrobial activity of up to 98.98% was observed, underlying the potential benefits of combining an alive antibiotic to a conventional drug (Im et al. Citation2017). This combination therapy would potentially have the advantage to minimise the risk of horizontal transfer of antibiotic resistance genes, which may occur upon therapy with conventional chemical antibiotics. Since the predator not only kills the pathogen, but also degrades the DNA present within the prey, it will limit the dispersion of resistance genes (Monnappa et al. Citation2013). Another example of combination treatment and probiotic or amphibiotic application was explored by Bonfiglio et al. who investigated the protective effects of B. bacteriovorus on the gut mucosa in cases of inflammatory bowel disease (Bonfiglio et al. Citation2019). The authors observed the capability of the predator to attenuate adherent-invasive E. coli strains (AIEC) both in planktonic and biofilm conditions. Aside from the killing capability of the B. bacteriovorus, the authors also reported that the presence of the predator would prevent the attachment of the pathogen to Caco-2 cell lines and an additional protective action was observed in larvae of the wax moth Galleria mellonella when B. bacteriovorus was used as a prophylactic or probiotic (Bonfiglio et al. Citation2019). Finally, the combined effect of bacteriophages and B. bacteriovorus has been investigated. In a study conducted by Hobley et al. it was reported that the synergy between predator and prey-specific bacteriophages is effective in countering E. coli (Hobley et al. Citation2020). In this synergistic scenario, the combination of phages with the predator overcomes the inability of the predator to eradicate the complete prey population and, at the same time, the rapid development of phage resistance, resulting in elimination of the preyed pathogen.
Other applications of bdellovibrio
In addition to the afore-mentioned applications, other potential uses of B. bacterivorous as an unorthodox biocontrol agent have been investigated, including environmental, food industry and oral health applications. The implications of B. bacterivorous could, thus, be more far-reaching than just healthcare-related. Regarding the food industry sector, the characteristics of the predator could be implemented to counter the degradation of aliments. For instance, B. bacteriovorus has been investigated as an agent to reduce the presence of bacteria belonging to the Pectobacterium and Dickeya species. These are plant pathogens that damage potato roots, which are responsible for losses in agricultural production (Youdkes et al. Citation2020). B. bacterivorous showed a concentration-dependent activity as well as underlining the protective effect that glucose has in regards to predation. B. bacteriovorus was also used as a biocontrol agent by Cao et al. who investigated the potential applications in regards to the fishing industry, specifically as a bio-disinfectant in countering shrimp pathogens (Cao et al. Citation2015). The same authors also implemented the first successful encapsulation of the predator, achieving to extend the bacterial viability and its stability at room temperature for up to 120 days (Cao et al. Citation2019). Another more environmental application of the predator was attempted in response to the need of pre-treating waters in order to reduce the concentration of pathogenic bacteria in rainwater prior to solar disinfection techniques based on UV light (Waso et al. Citation2020). Finally, another potential target for B. bacteriovorus has been identified in periodontal pathogens. Although the oral microbiota consists of a diverse community of bacteria, a recent study from Patini et al. documented the capability of B. bacteriovorus to effectively prey upon some oral pathogens (Patini et al. Citation2019). Evidence was presented that the predator was capable of killing aerobic species that colonise the oral cavity. Unfortunately, anaerobic conditions in which microorganisms, such as Porphyromonas gingivalis or F. nucleatum thrive, remain an insurmountable obstacle for the predator. The use of B. bacteriovorus in the contest of periodontitis-related infection was further investigated by inducing experimental periodontitis in rats. In such conditions, it was observed that the predator promoted a protective effect against bone loss (Silva et al. Citation2019).
Conclusion and outlook
The early stages in the B. bacteriovorus research history were marked by investigations focussed on fundamental aspects regarding the phenotypic characterisation of the predator. This included the identification of growth conditions, the life cycle and definition of the predatory capability. After these initial investigations, relatively few studies were documented essentially up to the beginning of the new millennium, until advances in genomic techniques allowed the complete sequencing of the bacterium. With the genome unveiled, a new frontier for studies on B. bacteriovorus opened and investigations on genotypic, proteomic, toxicologic and in vivo studies thrived. In parallel with progressing understanding of the predator, it became evident that the remarkable predatory capability on human pathogenic bacteria represents a characteristic that could be exploited to potentially use B. bacteriovorus as a “living antibiotic”. Likewise, the same traits could allow usage of B. bacteriovorus as a probiotic to prevent dangerous Gram-negative bacterial infections. Yet, the application of the predator as a probiotic should be considered with great care, as uncontrolled administration might damage the microbiome and rapidly elicit predation resistance.
Despite all recent advances, there are still many areas that require further investigations. These include a more detailed characterisation of the predator’s proteome and its functions. Regarding the predator’s application in vivo, further investigations should focus on the bacterium’s impact on the ecology of the microbiota of humans and livestock, the resistance strategies that prey uses to escape predation, the distinction of susceptible and resistant bacteria, or the possibilities for administration of the predator in the fight against systemic infections. Altogether, many challenges lie on the path of B. bacteriovorus, in order for this fascinating predator to be a useful tool in therapy. One of the main bottlenecks that B. bacteriovorus poses is its difficulty to be genetically modified. To address this issue, Flannagan et al. succeeded in proving the relevance for the flagellar motor complex operon MotAB and to insert a plasmid encoding the green fluorescent protein GFP in the predator (Flannagan et al. Citation2004). Another useful imaging tool was developed by Mukherjee et al. where a tdTomato fluorescent protein was engineered into the B. bacteriovorus strains 109 J HD and HI. This immensely facilitates the detection of the predator and eases the traditional reliance on culturomic techniques (Mukherjee et al. Citation2015). Further advances have been achieved in the field of synthetic biology by Dwidar and Yokobayashi, who developed a synthetic riboswitch for B. bacteriovorus, in order to be able to induce chemically the expression of genes (Dwidar and Yokobayashi Citation2017). From a pharmacodynamic perspective, Cao et al. achieved encapsulation of the predator allowing to considerably extend the predator’s usability and shelf life (Cao et al. Citation2019).
In conclusion, the coming decades are likely to be burdened by a progressive ineffectiveness of conventional antibiotics, which calls for alternative therapeutic options. As highlighted in the present review, B. bacteriovorus can potentially help us to meet this challenge as an attractive future control agent in the fight against antibiotic resistant pathogens.
Geolocation information
University Medical Centre Groningen. Hanzeplein 1, 9713 GZ Groningen, Netherlands. 050 361 6161/+31 50 361 6161. http://www.umcg.nl/. Geological coordinates: 53.2218504°N, 6.5760631°E.
Author contributions
FMC, CG and JMvD conceived and designed the review. FMC and LJ drafted, and AWF, CG and JMvD critically revised the manuscript. All authors have read and approved the final version.
Disclosure statement
The authors report no conflicts of interest.
Additional information
Funding
References
- Abram D, Castro e Melo J, Chou D. 1974. Penetration of Bdellovibrio bacteriovorus into host cells. J Bacteriol. 118(2):663–680.
- Ackermann H, Krisch HM, Comeau AM. 2011. Morphology and genome sequence of phage ϕ1402: a dwarf myovirus of the predatory bacterium Bdellovibrio bacteriovorus. Bacteriophage. 1(3):138–142.
- Althauser M, Samsonoff WA, Anderson C, Conti SF. 1972. Isolation and preliminary characterization of bacteriophages for Bdellovibrio bacteriovorus. J Virol. 10(3):516–523.
- Ashton PJ, Robarts RD. 1987. Apparent predation of microcystis aeruginosa kütz. Emend elenkin by a saprospira -like bacterium in a hypertrophic lake (Hartbeespoort dam, South Africa). J Limnol Soc South Afr. 13(1):44–47.
- Aslam B, Wang W, Arshad MI, Khurshid M, Muzammil S, Rasool MH, Nisar MA, Alvi RF, Aslam MA, Qamar MU, et al. 2018. Antibiotic resistance: a rundown of a global crisis. Infect Drug Resist. 11:1645–1658.
- Atterbury RJ, Hobley L, Till R, Lambert C, Capeness MJ, Lerner TR, Fenton AK, Barrow P, Sockett RE. 2011. Effects of Orally Administered Bdellovibrio bacteriovorus on the well-being and salmonella colonization of young chicks. Appl Environ Microbiol. 77(16):5794–5803.
- Baker M, Negus D, Raghunathan D, Radford P, Moore C, Clark G, Diggle M, Tyson J, Twycross J, Sockett RE, et al. 2017. Measuring and modelling the response of Klebsiella pneumoniae KPC prey to Bdellovibrio bacteriovorus predation, in human serum and defined buffer. Sci Rep. 7(1):8329.
- Bassetti M, Merelli M, Temperoni C, Astilean A. 2013. New antibiotics for bad bugs: where are we? Ann Clin Microbiol Antimicrob. 12(1):22.
- Bernardet J-F, Segers P, Vancanneyt M, Berthe F, Kersters K, Vandamme P. 1996. Cutting a Gordian Knot: emended classification and description of the genus flavobacterium, emended description of the family flavobacteriaceae, and proposal of Flavobacterium hydatis nom. nov. (Basonym, Cytophaga aquatilis Strohl and Tait 1978). Int J Syst Bacteriol. 46(1):128–148.
- Bohannan BJM, Lenski RE. 2000. Linking genetic change to community evolution: insights from studies of bacteria and bacteriophage. Ecol Letters. 3(4):362–377.
- Boileau MJ, Clinkenbeard KD, Iandolo JJ. 2011. Assessment of Bdellovibrio bacteriovorus 109J killing of Moraxella bovis in an in vitro model of infectious bovine keratoconjunctivitis. Can J Vet Res. 75(4):285–291.
- Bonfiglio G, Neroni B, Radocchia G, Pompilio A, Mura F, Trancassini M, Di Bonaventura G, Pantanella F, Schippa S. 2019. Growth control of adherent-invasive Escherichia coli (AIEC) by the predator bacteria Bdellovibrio bacteriovorus: a new therapeutic approach for Crohn’s disease patients. Microorganisms. 8(1):17.
- Bonfiglio G, Neroni B, Radocchia G, Marazzato M, Pantanella F, Schippa S. 2020. Insight into the possible use of the predator Bdellovibrio bacteriovorus as a probiotic. Nutrients. 12(8):2252.
- Borgnia MJ, Subramaniam S, Milne JLS. 2008. Three-dimensional imaging of the highly bent architecture of Bdellovibrio bacteriovorus by using cryo-electron tomography. J Bacteriol. 190(7):2588–2596.
- Boucher HW, Talbot GH, Bradley JS, Edwards JE, Gilbert D, Rice LB, Scheld M, Spellberg B, Bartlett J. 2009. Bad bugs, no drugs: no ESKAPE! An update from the infectious diseases society of America. Clin Infect Dis. 48(1):1–12.
- Bratanis E, Andersson T, Lood R, Bukowska-Faniband E. 2020. Biotechnological potential of bdellovibrio and like organisms and their secreted enzymes. Front Microbiol. 11(April):1–14.
- Brentlinger KL, Hafenstein S, Novak CR, Fane BA, Borgon R, McKenna R, Agbandje-McKenna M. 2002. Microviridae, a family divided: isolation, characterization, and genome sequence of phimh2k, a bacteriophage of the obligate intracellular parasitic bacterium Bdellovibrio bacteriovorus. J Bacteriol. 184(4):1089–1094.
- Bukowska-Faniband E, Andersson T, Lood R. 2020. Studies on Bd0934 and Bd3507, two secreted nucleases from bdellovibrio bacteriovorus, reveal sequential release of nucleases during the predatory cycle. J Bacteriol. 202(18):e00150-20.
- Burnham JC, Hashimoto T, Conti SF. 1968. Electron microscopic observations on the penetration of Bdellovibrio bacteriovorus into gram-negative bacterial hosts. J Bacteriol. 96(4):1366–1381.
- Burnham JC, Hashimoto T, Conti SF. 1970. Ultrastructure and cell division of a facultatively parasitic strain of Bdellovibrio bacteriovorus. J Bacteriol. 101(3):997–1004.
- Cao H, An J, Zheng W, He S. 2015. Vibrio cholerae pathogen from the freshwater-cultured whiteleg shrimp Penaeus vannamei and control with Bdellovibrio bacteriovorus. J Invertebr Pathol. 130 :13–20.
- Cao H, Wang H, Yu J, An J, Chen J. 2019. Encapsulated bdellovibrio powder as a potential bio-disinfectant against whiteleg shrimp-pathogenic vibrios. Microorganisms. 7(8):244.
- Carbonnelle E, Hélaine S, Prouvensier L, Nassif X, Pelicic V. 2005. Type IV pilus biogenesis in Neisseria meningitidis: PilW is involved in a step occurring after pilus assembly, essential for fibre stability and function. Mol Microbiol. 55(1):54–64.
- Casida LE. 1982. Ensifer adhaerens gen. nov., sp. nov.: a bacterial predator of bacteria in soil. Int J Syst Bacteriol. 32(3):339–345.
- Chanyi RM, Koval SF. 2014. Role of type IV Pili in predation by Bdellovibrio bacteriovorus. PLoS One. 9(11):e113404.
- Cho G, Kwon J, Soh SM, Jang H, Mitchell RJ. 2019. Sensitivity of predatory bacteria to different surfactants and their application to check bacterial predation. Appl Microbiol Biotechnol. 103(19):8169–8178.
- Christensen P, Cook FD. 1978. Lysobacter, a new genus of nonfruiting, gliding bacteria with a high base ratio. Int J Syst Bacteriol. 28(3):367–393.
- Chu W-H, Zhu W. 2010. Isolation of bdellovibrio as biological therapeutic agents used for the treatment of Aeromonas hydrophila infection in fish. Zoonoses Public Health. 57(4):258–264.
- Dashiff A, Junka RA, Libera M, Kadouri DE. 2011. Predation of human pathogens by the predatory bacteria Micavibrio aeruginosavorus and Bdellovibrio bacteriovorus. J Appl Microbiol. 110(2):431–444.
- Dashiff A, Kadouri DE. 2011. Predation of oral pathogens by Bdellovibrio bacteriovorus 109J. Mol Oral Microbiol. 26(1):19–34.
- Dashiff A, Keeling TG, Kadouri DE. 2011. Inhibition of predation by Bdellovibrio bacteriovorus and Micavibrio aeruginosavorus via host cell metabolic activity in the presence of carbohydrates. Appl Environ Microbiol. 77(7):2224–2231.
- Dharani S, Kim DH, Shanks RMQ, Doi Y, Kadouri DE. 2018. Susceptibility of colistin-resistant pathogens to predatory bacteria. Res Microbiol. 169(1):52–55.
- Dori-Bachash M, Dassa B, Pietrokovski S, Jurkevitch E. 2008. Proteome-based comparative analyses of growth stages reveal new cell cycle-dependent functions in the predatory bacterium Bdellovibrio bacteriovorus. Appl Environ Microbiol. 74(23):7152–7162.
- Duncan MC, Gillette RK, Maglasang MA, Corn EA, Tai AK, Lazinski DW, Shanks RMQ, Kadouri DE, Camilli A. 2019. High-throughput analysis of gene function in the bacterial predator Bdellovibrio bacteriovorus. mBio. 10(3):1–12.
- Durán N, Justo GZ, Ferreira CV, Melo PS, Cordi L, Martins D. 2007. Violacein: properties and biological activities. Biotechnol Appl Biochem. 48(Pt 3):127–133.
- Dwidar M, Monnappa AK, Mitchell RJ. 2012. The dual probiotic and antibiotic nature of Bdellovibrio bacteriovorus. BMB Rep. 45(2):71–78.
- Dwidar M, Nam D, Mitchell RJ. 2015. Indole negatively impacts predation by Bdellovibrio bacteriovorus and its release from the bdelloplast. Environ Microbiol. 17(4):1009–1022.
- Dwidar M, Yokobayashi Y. 2017. Controlling Bdellovibrio bacteriovorus gene expression and predation using synthetic riboswitches. ACS Synth Biol. 6(11):2035–2041.
- Evans KJ, Lambert C, Sockett RE. 2007. Predation by Bdellovibrio bacteriovorus HD100 requires type IV Pili. J Bacteriol. 189(13):4850–4859.
- Fenton AK, Kanna M, Woods RD, Aizawa S-I, Sockett RE. 2010. Shadowing the actions of a predator: backlit fluorescent microscopy reveals synchronous nonbinary septation of predatory bdellovibrio inside prey and exit through discrete bdelloplast pores. J Bacteriol. 192(24):6329–6335.
- Filippini M, Kaech A, Ziegler U, Bagheri HC. 2011. Fibrisoma limi gen. nov., sp. nov., a filamentous bacterium isolated from tidal flats. Int J Syst Evol Microbiol. 61(6):1418–1424.
- Filippini M, Svercel M, Laczko E, Kaech A, Ziegler U, Bagheri HC. 2011. Fibrella aestuarina gen. nov., sp. nov., a filamentous bacterium of the family Cytophagaceae isolated from a tidal flat, and emended description of the genus Rudanella Weon et al. 2008. Int J Syst Evol Microbiol. 61(Pt 1):184–189.
- Findlay JS, Flick-Smith HC, Keyser E, Cooper IA, Williamson ED, Oyston PCF. 2019. Predatory bacteria can protect SKH-1 mice from a lethal plague challenge. Sci Rep. 9(1):7225.
- Flannagan RS, Valvano MA, Koval SF. 2004. Downregulation of the motA gene delays the escape of the obligate predator Bdellovibrio bacteriovorus 109J from bdelloplasts of bacterial prey cells. Microbiology. 150(Pt 3):649–656.
- Flemming H-C, Wingender J, Szewzyk U, Steinberg P, Rice SA, Kjelleberg S. 2016. Biofilms: an emergent form of bacterial life. Nat Rev Microbiol. 14(9):563–575.
- Gledhill WE, Casida LE. 1969. Predominant catalase-negative soil bacteria. III. Agromyces, gen. n., microorganisms intermediary to actinomyces and nocardia. Appl Microbiol. 18(3):340–349.
- Guerrero R, Pedros-Alio C, Esteve I, Mas J, Chase D, Margulis L. 1986. Predatory prokaryotes: predation and primary consumption evolved in bacteria. Proc Natl Acad Sci U S A. 83(7):2138–2142.
- Gupta S, Tang C, Tran M, Kadouri DE. 2016. Effect of predatory bacteria on human cell lines. PLOS One. 11(8):e0161242.
- Hahn MW, Höfle MG. 1999. Flagellate predation on a bacterial model community: interplay of size-selective grazing, specific bacterial cell size, and bacterial community composition. Appl Environ Microbiol. 65(11):4863–4872.
- Hall CW, Mah T-F. 2017. Molecular mechanisms of biofilm-based antibiotic resistance and tolerance in pathogenic bacteria. FEMS Microbiol Rev. 41(3):276–301.
- Hall-Stoodley L, Costerton JW, Stoodley P. 2004. Bacterial biofilms: from the Natural environment to infectious diseases. Nat Rev Microbiol. 2(2):95–108.
- Hart BA, Zahler SA. 1966. Lytic enzyme produced by Myxococcus xanthus. J Bacteriol. 92(6):1632–1637.
- Hashimoto T, Diedrich DL, Conti SF. 1970. Isolation of a bacteriophage for Bdellovibrio bacteriovorus. J Virol. 5(1):97–98.
- Herencias C, Prieto MA, Martínez V. 2017. Determination of the predatory capability of bdellovibrio bacteriovorus HD100. Bio-Protocol. 7(6). DOI:10.21769/BioProtoc.2177.
- Hobley L, Summers JK, Till R, Milner DS, Atterbury RJ, Stroud A, Capeness MJ, Gray S, Leidenroth A, Lambert C, et al. 2020. Dual predation by bacteriophage and Bdellovibrio bacteriovorus can eradicate Escherichia coli prey in situations where single predation cannot. J Bacteriol. 202(6):1–18.
- Hugh R, Leifson E. 1963. A description of the type strain of Pseudomonas maltophilia. Int Bull Bact Nomen Tax. 13(3):133–138.
- Iebba V, Totino V, Santangelo F, Gagliardi A, Ciotoli L, Virga A, Ambrosi C, Pompili M, De Biase RV, Selan L, et al. 2014. Bdellovibrio bacteriovorus directly attacks Pseudomonas aeruginosa and Staphylococcus aureus cystic fibrosis isolates. Front Microbiol. 5:1–9.
- Imai I, Ishida Y, Hata Y. 1993. Killing of marine phytoplankton by a gliding bacterium Cytophaga sp., isolated from the coastal sea of Japan. Mar Biol. 116(4):527–532.
- Im H, Choi SY, Son S, Mitchell RJ. 2017. Combined application of bacterial predation and violacein to kill polymicrobial pathogenic communities. Sci Rep. 7(1):14415.
- Im H, Dwidar M, Mitchell RJ. 2018. Bdellovibrio bacteriovorus HD100, a predator of Gram-negative bacteria, benefits energetically from Staphylococcus aureus biofilms without predation. ISME J. 12(8):2090–2095.
- Im H, Son S, Mitchell RJ, Ghim C-M. 2017. Serum albumin and osmolality inhibit Bdellovibrio bacteriovorus predation in human serum. Sci Rep. 7(1):5896.
- Johnke J, Boenigk J, Harms H, Chatzinotas A. 2017. Killing the killer: predation between protists and predatory bacteria. FEMS Microbiol Lett. 364(9):1–8.
- Jurkevitch É, Jacquet S. 2017. [Bdellovibrio and like organisms: outstanding predators!]. Med Sci (Paris). 33(5):519–527.
- Kadouri D, O’Toole GA. 2005. Susceptibility of biofilms to Bdellovibrio bacteriovorus attack. Appl Environ Microbiol. 71(7):4044–4051.
- Kadouri DE, Tran A. 2013. Measurement of predation and biofilm formation under different ambient oxygen conditions using a simple gasbag-based system. Appl Environ Microbiol. 79(17):5264–5271.
- Kakasis A, Panitsa G. 2019. Bacteriophage therapy as an alternative treatment for human infections. A comprehensive review. Int J Antimicrob Agents. 53(1):16–21.
- Kern WV. 2015. Antibacterial agents. In Infections in hematology. Berlin, Heidelberg: Springer Berlin Heidelberg; p. 229–258.
- Kessel M, Varon M. 1973. Development of bdellophage VL-1 in parasitic and saprophytic bdellovibrios. J Virol. 12(6):1522–1533.
- Koval SF, Hynes SH, Flannagan RS, Pasternak Z, Davidov Y, Jurkevitch E. 2013. Bdellovibrio exovorus sp. nov., a novel predator of Caulobacter crescentus. Int J Syst Evol Microbiol. 63(Pt 1):146–151.
- Koval SF, Bayer ME. 1997. Bacterial capsules: no barrier against Bdellovibrio. Microbiology. 143(3):749–753.
- Koval SF, Hynes SH. 1991. Effect of paracrystalline protein surface layers on predation by Bdellovibrio bacteriovorus. J Bacteriol. 173(7):2244–2249.
- Kowalska J, Włodarczyk M. 2017. Predation among microorganisms: a huge potential of interspecies dependencies. Postepy Hig Med Dosw. 71:906–914.
- Kumbhar C, Mudliar P, Bhatia L, Kshirsagar A, Watve M. 2014. Widespread predatory abilities in the genus Streptomyces. Arch Microbiol. 196(4):235–248.
- Kuru E, Lambert C, Rittichier J, Till R, Ducret A, Derouaux A, Gray J, Biboy J, Vollmer W, VanNieuwenhze M, et al. 2017. Fluorescent D-amino-acids reveal bi-cellular cell wall modifications important for Bdellovibrio bacteriovorus predation. Nat Microbiol. 2(12):1648–1657.
- Laloux G. 2019. Shedding light on the cell biology of the predatory bacterium Bdellovibrio bacteriovorus. Front Microbiol. 10(January):3136–3138.
- Lambert C, Evans KJ, Till R, Hobley L, Capeness M, Rendulic S, Schuster SC, Aizawa S-I, Sockett RE. 2006. Characterizing the flagellar filament and the role of motility in bacterial prey-penetration by Bdellovibrio bacteriovorus. Mol Microbiol. 60(2):274–286.
- Lambert C, Chang C-Y, Capeness MJ, Sockett RE. 2010. The First bite-profiling the predatosome in the bacterial pathogen Bdellovibrio. PLoS One. 5(1):e8599.
- Lambert C, Lerner TR, Bui NK, Somers H, Aizawa S-I, Liddell S, Clark A, Vollmer W, Lovering AL, Sockett RE, et al. 2016. Interrupting peptidoglycan deacetylation during Bdellovibrio predator-prey interaction prevents ultimate destruction of prey wall, liberating bacterial-ghosts. Sci Rep. 6:26010.
- Lambina VA, et al. 1983. [New species of exoparasitic bacteria of the genus Micavibrio infecting gram-positive bacteria]. Mikrobiologiia. 52(5):777–780.
- Lerner TR, Lovering AL, Bui NK, Uchida K, Aizawa S-i, Vollmer W, Sockett RE. 2012. Specialized peptidoglycan hydrolases sculpt the intra-bacterial niche of predatory bdellovibrio and increase population fitness. PLoS Pathog. 8(2):e1002524.
- Lin DM, Koskella B, Lin HC. 2017. Phage therapy: an alternative to antibiotics in the age of multi-drug resistance. World J Gastrointest Pharmacol Ther. 8(3):162–173.
- Liu Y-Y, Chandler CE, Leung LM, McElheny CL, Mettus RT, Shanks RMQ, Liu J-H, Goodlett DR, Ernst RK, Doi Y, et al. 2017. Structural modification of lipopolysaccharide conferred by mcr-1 in gram-negative ESKAPE pathogens. Antimicrob Agents Chemother. 61(6):1–9.
- Lotka AJ. 1920. Analytical note on certain rhythmic relations in organic systems. Proc Natl Acad Sci U S A. 6(7):410–415.
- Lyddiard D, Jones GL, Greatrex BW. 2016. Keeping it simple: lessons from the golden era of antibiotic discovery. FEMS Microbiol Lett. 363(8):fnw084.
- Mahmoud KK, Koval SF. 2010. Characterization of type IV pili in the life cycle of the predator bacterium Bdellovibrio. Microbiology. 156(Pt 4):1040–1051.
- Makkar NS, Casida LE. 1987. Cupriavidus necator gen. nov., sp. nov.; a nonobligate bacterial predator of bacteria in soil. Int J Syst Bacteriol. 37(4):323–326.
- Makowski Ł, Trojanowski D, Till R, Lambert C, Lowry R, Sockett RE, Zakrzewska-Czerwińska J. 2019. Dynamics of chromosome replication and its relationship to predatory attack lifestyles in Bdellovibrio bacteriovorus. Appl Environ Microbiol. 85(14):e00730–19.
- Marine E, Milner DS, Lambert C, Sockett RE, Pos KM. 2020. A novel method to determine antibiotic sensitivity in Bdellovibrio bacteriovorus reveals a DHFR-dependent natural trimethoprim resistance. Sci Rep. 10(1):5315.
- Medina AA, Kadouri DE. 2009. Biofilm formation of Bdellovibrio bacteriovorus host-independent derivatives. Res Microbiol. 160(3):224–231.
- Melo LDR, Oliveira H, Pires DP, Dabrowska K, Azeredo J. 2020. Phage therapy efficacy: a review of the last 10 years of preclinical studies. Crit Rev Microbiol. 46(1):78–99.
- Mitchell RJ, Mun W, Mabekou SS, Jang H, Choi SY. 2020. Compounds affecting predation by and viability of predatory bacteria. Appl Microbiol Biotechnol. 104(9):3705–3713.
- Mobarki N, Almerabi B, Hattan A. 2019. Antibiotic resistance crisis. IJMDC. 40(4):561–564.
- Monnappa AK, Bari W, Choi SY, Mitchell RJ. 2016. Investigating the responses of human epithelial cells to predatory bacteria. Sci Rep. 6(1):33485.
- Monnappa AK, Dwidar M, Mitchell RJ. 2013. Application of bacterial predation to mitigate recombinant bacterial populations and their DNA. Soil Biol Biochem. 57 :427–435.
- Mu D-S, Wang S, Liang Q-Y, Du Z-Z, Tian R, Ouyang Y, Wang X-P, Zhou A, Gong Y, Chen G-J, et al. 2020. Bradymonabacteria, a novel bacterial predator group with versatile survival strategies in saline environments. Microbiome. 8(1):126.
- Mukherjee S, Brothers KM, Shanks RMQ, Kadouri DE. 2015. Visualizing Bdellovibrio bacteriovorus by using the tdtomato fluorescent protein. Appl Environ Microbiol. 82(6):1653–1661.
- Mun W, Kwon H, Im H, Choi SY, Monnappa AK, Mitchell RJ. 2017. Cyanide production by Chromobacterium piscinae shields It from Bdellovibrio bacteriovorus HD100 predation. mBio. 8(6):1–12.
- Myelnikov D. 2018. An alternative cure: the adoption and survival of bacteriophage therapy in the USSR, 1922–1955. J Hist Med Allied Sci. 73(4):385–411.
- Nakamura M. 1972. Alteration of Shigella pathogenicity by other bacteria. Am J Clin Nutr. 25(12):1441–1451.
- Negus D, Moore C, Baker M, Raghunathan D, Tyson J, Sockett RE. 2017. Predator versus pathogen: how does predatory Bdellovibrio bacteriovorus interface with the challenges of killing gram-negative pathogens in a host setting? Annu Rev Microbiol. 71(1):441–457.
- Neill JO. 2014. Review on Antimicrobial Resistance. Antimicrobial Resistance: Tackling a Crisis for the Health and Wealth of Nations. http://amr-review.org/Publications.
- Núñez ME, Martin MO, Chan PH, Spain EM. 2005. Predation, death, and survival in a biofilm: bdellovibrio investigated by atomic force microscopy. Colloids Surf B Biointerfaces. 42(3–4):263–271.
- Oyedara OO, De Luna-Santillana E. d J, Olguin-Rodriguez O, Guo X, Mendoza-Villa MA, Menchaca-Arredondo JL, Elufisan TO, Garza-Hernandez JA, Garcia Leon I, Rodriguez-Perez MA, et al. 2016. Isolation of Bdellovibrio sp. from soil samples in Mexico and their potential applications in control of pathogens. Microbiologyopen. 5(6):992–1002.
- Pantanella F, Iebba V, Mura F, Dini L, Totino V, Neroni B, Bonfiglio G, Maria T, Passariello C, Schippa S, et al. 2018. Behaviour of Bdellovibrio bacteriovorus in the presence of Gram-positive Staphylococcus aureus. New Microbiol. 41(2):145–152.
- Pasternak Z, Pietrokovski S, Rotem O, Gophna U, Lurie-Weinberger MN, Jurkevitch E. 2013. By their genes ye shall know them: genomic signatures of predatory bacteria. ISME J. 7(4):756–769.
- Patini R, Cattani P, Marchetti S, Isola G, Quaranta G, Gallenzi P. 2019. Evaluation of predation capability of periodontopathogens bacteria by Bdellovibrio Bacteriovorus HD100. an in vitro study. Materials. 12(12):2008.
- Pérez J, Moraleda-Muñoz A, Marcos-Torres FJ, Muñoz-Dorado J. 2016. Bacterial predation: 75 years and counting! Environ Microbiol. 18(3):766–779.
- Popkov VA, Plotnikov EY, Silachev DN, Zorova LD, Pevzner IB, Jankauskas SS, Zorov SD, Andrianova NV, Babenko VA, Zorov DB, et al. 2017. Bacterial therapy and mitochondrial therapy. Biochemistry (Mosc). 82(12):1549–1556.
- Quinn GR, Skerman VBD. 1980. Herpetosiphon—Nature’s scavenger? Curr Microbiol. 4(1):57–62.
- Raghunathan D, Radford PM, Gell C, Negus D, Moore C, Till R, Tighe PJ, Wheatley SP, Martinez-Pomares L, Sockett RE, et al. 2019. Engulfment, persistence and fate of Bdellovibrio bacteriovorus predators inside human phagocytic cells informs their future therapeutic potential. Sci Rep. 9(1):4293.
- Rendulic S, Jagtap P, Rosinus A, Eppinger M, Baar C, Lanz C, Keller H, Lambert C, Evans KJ, Goesmann A, et al. 2004. A predator unmasked: life cycle of Bdellovibrio bacteriovorus from a genomic perspective. Science. 303(5658):689–692.
- Rice LB. 2008. Federal funding for the study of antimicrobial resistance in nosocomial pathogens: no ESKAPE. J Infect Dis. 197(8):1079–1081.
- Roberts RC, Keefer MA, Ranu RS. 1987. Characterization of Bdellovibrio bacteriovorus Bacteriophage MAC-1. J Gen Microbiol. 133(11):3065–3070.
- Romanowski EG, Stella NA, Brothers KM, Yates KA, Funderburgh ML, Funderburgh JL, Gupta S, Dharani S, Kadouri DE, Shanks RMQ, et al. 2016. Predatory bacteria are nontoxic to the rabbit ocular surface. Sci Rep. 6(1):30987.
- Ruban-Ośmiałowska B, Jakimowicz D, Smulczyk-Krawczyszyn A, Chater KF, Zakrzewska-Czerwińska J. 2006. Replisome localization in vegetative and aerial hyphae of Streptomyces coelicolor. J Bacteriol. 188(20):7311–7316.
- Schoeffield AJ, Williams HN, Turng B, Fackler WA. 1996. A comparison of the survival of intraperiplasmic and attack phase bdellovibrios with reduced oxygen. Microb Ecol. 32(1):35–46.
- Schwudke D, Linscheid M, Strauch E, Appel B, Zahringer U, Moll H, Muller M, Brecker L, Gronow S, Lindner B, et al. 2003. The obligate predatory Bdellovibrio bacteriovorus possesses a neutral lipid A containing alpha-D-mannoses that replace phosphate residues: similarities and differences between the lipid As and the lipopolysaccharides of the wild type strain B. bacteriovorus HD100 and its host-independent derivative HI100. J Biol Chem. 278(30):27502–27512.
- Seed KD, Faruque SM, Mekalanos JJ, Calderwood SB, Qadri F, Camilli A. 2012. Phase variable O antigen biosynthetic genes control expression of the major protective antigen and bacteriophage receptor in vibrio cholerae O1. PLoS Pathog. 8(9):e1002917.
- Seidler RJ, Starr MP. 1969. Factors affecting the intracellular parasitic growth of Bdellovibrio bacteriovorus developing within Escherichia coli. J Bacteriol. 97(2):912–923.
- Shanks RM, Kadouri DE. 2014. Predatory prokaryotes wage war against eye infections. Future Microbiol. 9(4):429–432.
- Shatzkes K, Chae R, Tang C, Ramirez GC, Mukherjee S, Tsenova L, Connell ND, Kadouri DE. 2015. Examining the safety of respiratory and intravenous inoculation of Bdellovibrio bacteriovorus and Micavibrio aeruginosavorus in a mouse model. Sci Rep. 5(1):12899.
- Shatzkes K, Singleton E, Tang C, Zuena M, Shukla S, Gupta S, Dharani S, Onyile O, Rinaggio J, Connell ND, et al. 2016. Predatory bacteria attenuate klebsiella pneumoniae burden in rat lungs. mBio. 7(6):1–9.
- Shatzkes K, Singleton E, Tang C, Zuena M, Shukla S, Gupta S, Dharani S, Rinaggio J, Kadouri DE, Connell ND, et al. 2017. Examining the efficacy of intravenous administration of predatory bacteria in rats. Sci Rep. 7(1):1864.
- Shemesh Y, Jurkevitch E. 2004. Plastic phenotypic resistance to predation by Bdellovibrio and like organisms in bacterial prey. Environ Microbiol. 6(1):12–18.
- Silhavy TJ, Kahne D, Walker S. 2010. The bacterial cell envelope. Cold Spring Harb Perspect Biol. 2(5):a000414.
- Silva PHF, Oliveira LFF, Cardoso RS, Ricoldi MST, Figueiredo LC, Salvador SL, Palioto DB, Furlaneto FAC, Messora MR. 2019. The impact of predatory bacteria on experimental periodontitis. J Periodontol. 90(9):1053–1063.
- Snyder AR, Williams HN, Baer ML, Walker KE, Stine OC. 2002. 16S rDNA sequence analysis of environmental Bdellovibrio-and-like organisms (BALO) reveals extensive diversity. Int J Syst Evol Microbiol. 52(Pt 6):2089–2094.
- Stanczak-Mrozek KI, Laing KG, Lindsay JA. 2017. Resistance gene transfer: induction of transducing phage by sub-inhibitory concentrations of antimicrobials is not correlated to induction of lytic phage. J Antimicrob Chemother. 72(6):1624–1631.
- Starr MP, Baigent NL. 1966. Parasitic interaction of Bdellovibrio bacteriovorus with other bacteria. J Bacteriol. 91(5):2006–2017.
- Steiner S, Conti SF, Lester RL. 1973. Occurrence of phosphonosphingolipids in Bdellovibrio bacteriovorus Strain UKi2. J Bacteriol. 116(3):1199–1211.
- Stolp H, Starr MP. 1963. Bdellovibrio bacteriovorus gen. et sp. n., a predatory, ectoparasitic, and bacteriolytic microorganism. Antonie Van Leeuwenhoek. 29(1):217–248.
- Strauch E, Schwudke D, Linscheid M. 2007. Predatory mechanisms of bdellovibrio and like organisms. Future Microbiol. 2(1):63–73.
- Sun Y, Ye J, Hou Y, Chen H, Cao J, Zhou T. 2017. Predation efficacy of Bdellovibrio bacteriovorus on multidrug-resistant clinical pathogens and their corresponding biofilms. Jpn J Infect Dis. 70(5):485–489.
- Thomashow MF, Cotter TW. 1992. Bdellovibrio host dependence: the search for signal molecules and genes that regulate the intraperiplasmic growth cycle. J Bacteriol. 174(18):5767–5771.
- Thomashow MF, Rittenberg SC. 1978. Intraperiplasmic growth of Bdellovibrio bacteriovorus 109J: solubilization of Escherichia coli peptidoglycan. J Bacteriol. 135(3):998–1007.
- Tudor JJ, McCann MP, Acrich IA. 1990. A new model for the penetration of prey cells by bdellovibrios. J Bacteriol. 172(5):2421–2426.
- Tyc O, Song C, Dickschat JS, Vos M, Garbeva P. 2017. The ecological role of volatile and soluble secondary metabolites produced by soil bacteria. Trends Microbiol. 25(4):280–292.
- Varon M, Levisohn R. 1972. Three-membered parasitic system: a bacteriophage, Bdellovibrio bacteriovorus, and Escherichia coli. J Virol. 9(3):519–525.
- Varon M, Shilo M. 1969. Interaction of Bdellovibrio bacteriovorus and Host Bacteria II. Intracellular Growth and Development of Bdellovibrio bacteriovorus in Liquid Cultures. J Bacteriol. 99(1):136–141.
- Ventola CL. 2015. The antibiotic resistance crisis: part 1: causes and threats. P T. 40(4):277–283.
- Volterra V. 1926. Fluctuations in the abundance of a species considered mathematically1. Nature. 118(2972):558–560.
- Waso M, Khan S, Singh A, McMichael S, Ahmed W, Fernández-Ibáñez P, Byrne JA, Khan W. 2020. Predatory bacteria in combination with solar disinfection and solar photocatalysis for the treatment of rainwater. Water Res. 169 :115281.
- Willis AR, Moore C, Mazon-Moya M, Krokowski S, Lambert C, Till R, Mostowy S, Sockett RE. 2016. Injections of predatory bacteria work alongside host immune cells to treat shigella infection in zebrafish larvae. Curr Biol. 26(24):3343–3351.
- Wolanski M, Wali R, Tilley E, Jakimowicz D, Zakrzewska-Czerwinska J, Herron P. 2011. Replisome trafficking in growing vegetative hyphae of Streptomyces coelicolor A3(2). J Bacteriol. 193(5):1273–1275.
- Youdkes D, Helman Y, Burdman S, Matan O, Jurkevitch E. 2020. Potential control of potato soft rot disease by the obligate predators bdellovibrio and like organisms. Appl Environ Microbiol. 86(6):1–13.